DOI:
10.1039/C6RA20691C
(Paper)
RSC Adv., 2016,
6, 94661-94668
Injectable camptothecin conjugated hydrogels with simultaneous drug release and degradation†
Received
17th August 2016
, Accepted 20th September 2016
First published on 26th September 2016
Abstract
Hydrogels loaded with anticancer drugs can be regarded as depots for intratumoral chemotherapy to downsize tumors. However, the low drug loading content, the rapid drug release, and the subsequently undegraded blank hydrogel matrix may limit their applications. In this work, we prepared an injectable camptothecin (CPT) conjugated hydrogel with simultaneous drug release and degradation to resolve these problems. Poly[oligo(ethylene glycol) maleate] (POEGM) with two terminal hydroxyl groups was first synthesized by the direct polycondensation of oligo(ethylene glycol) (OEG) with maleic acid (MA). CPT, a highly hydrophobic anticancer drug, was conjugated to the chain end of POEGM via carbonic ester bond. The resultant water-soluble CPT–polymer conjugate could be in situ crosslinked by poly[oligo(ethylene glycol) mercaptosuccinate] (POEGMS) rapidly through thiol-ene “click” reaction under physiological conditions, which can be used as injectable CPT conjugated hydrogels. Bright blue fluorescence emitted from CPT-conjugated hydrogel under UV lamp demonstrated the homogeneity of CPT loading. The CPT release by the breakage of carbonic ester bond and the degradation of hydrogels by the breakage of ester bonds were observed simultaneously within one week to 16 days, depending on the solid content of hydrogels. The CPT-conjugated hydrogels exhibited significant cytotoxicity to HepG2 cells based on in vitro cell viability assays. As a contrast, the blank hydrogels without CPT conjugation showed excellent biocompatibility under the same conditions. This kind of CPT conjugated hydrogels could be a potential candidate for intratumor drug delivery.
Introduction
Removing tumors by surgical resection is the most effective treatment for localized solid tumors. However, treatment of cancer is a complex project and needs much deliberation according to the size, stage, rate of growth, location, as well as the type of tumor. Surgical resection is not suitable for many cancers, and not every patient is a candidate for surgical procedure.1,2 Chemotherapy is a parallel treatment to downsize solid tumors. While an anticancer agent is administrated intravenously, high plasma concentrations in the systemic circulation can result in undesirable side effects with just a portion of the entire administered dose reaching the tumor site.3–5 Compared to intravenous administration of anticancer agents, local treatment or intratumor therapy presents a number of advantages, such as finer control over drug concentration at solid tumor sites, less off-target side effects and longer drug release time (weeks to months). At each stage of cancer, there is potential intervention point, in which localized therapy could be implemented or completely replace the existing treatments. It is applicable to both improving the efficiency of treatment and lowering the recurrence rate.6–9
Pre-shaped implant and injectable gelling depots are two types of intratumor carriers for anticancer drugs. In situ forming depots with injectability have shown less invasive than pre-formed implants, which can greatly reduce the pain of the patients, making them desirable systems for local delivery of anticancer drugs.10–13 Drug-loaded hydrogels permit drug releasing through their three-dimensional cross-linked networks, and now has sparked particular interest as intratumor delivery system.14–22 While problems relating to the drug delivery properties of hydrogels should be regarded with some attention. The quantity of drug loading into hydrogels may be limited, particularly in the case of hydrophobic drugs. The high water content and large pore sizes of hydrogels result in relatively rapid drug release, over a few hours to a couple of days.23–25 In most cases, the sustained release time of drugs from hydrogels is much shorter than the degradation time of hydrogels. Undegraded blank hydrogels can re-load the drug released from the newly injected hydrogels, which will discount the drug concentration in the tumor site and lower the efficiency of chemotherapy.
CPT and its derivatives such as topotecan (TPT) and 10-hydrocamptothecin (HCPT) are effective topomerase inhibitors, and have been widely applied in tumor treatments.26–28 However, their use is hampered by high hydrophobicity, rapid metabolism, short plasma half-life, or low selectivity towards tumor tissue.29 Therefore, local delivery of CPT to tumor site is expected to be an effective strategy to fight cancer.
Poly(ethylene glycol) (PEG) is a kind of FDA approved polymer, and has been widely used in biomedical applications.30–38 In situ fabrication of physically or chemically cross-linked PEG-based hydrogels represents a promising matrix for drug delivery.39–41 In our previous work, we have synthesized a serious of “clickable” PEG derivatives with multiple thiols or double bonds by direct polycondensation of oligo(ethylene glycol) (OEG) diol with corresponding functional diacids, which can in situ form PEG hydrogels rapidly via thiol-ene “click” reaction under physiological conditions.42–45 Most recently, we further synthesized a novel “clickable” PEG based amphiphilic triblock by a combination of above mentioned polycondensation and ring-opening polymerization. Poly(ε-caprolactone) (PCL), a hydrophobic biocompatible polyester block in the copolymer, was responsible for the solubilisation of hydrophobic drugs.46 The hydrogels based on the triblock copolymer can release the loaded drugs within 12 h to three days. However, the degradation time of hydrogels was up to three weeks. Furthermore, many antitumor agents, such as CPT, can't be dispersed into the hydrogels with PCL blocks because of their strong hydrophobicity.
The conjugation of drug onto water-soluble polymer represents a general method to deliver hydrophobic drugs.47–56 In this study, poly[oligo(ethylene glycol) maleate] (POEGM) with two terminal hydroxyl groups was first synthesized by OEG and maleic acid (MA), controlling the feed ratio of OEG to MA as 10/9. Then highly hydrophobic CPT was conjugated to the chain end of POEGM through a phosgene strategy, to give water soluble CPT–POEGM conjugate, which was further crosslinked by poly[oligo(ethylene glycol) mercaptosuccinate] (POEGMS) rapidly under physiological condition, as shown in Scheme 1. Thanks to the similar hydrolyzability of ester bond and carbonic ester bond, the CPT-loaded hydrogels exhibited simultaneous drug release and degradation behaviors.
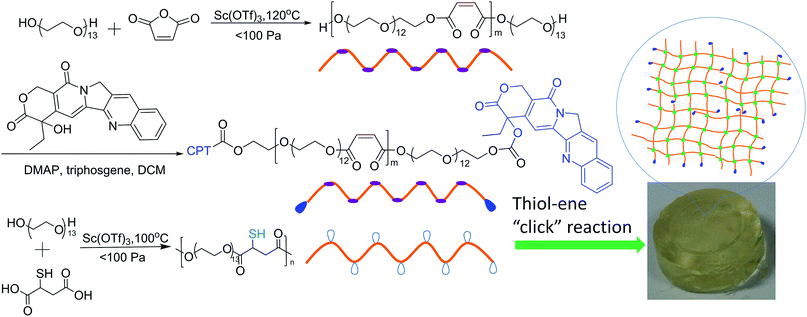 |
| Scheme 1 Preparation of injectable CPT conjugated hydrogel. | |
Experimental
Materials
OEG (Mn = 600 g mol−1, Adamas-beta) was dried by azeotropic distillation in the presence of dry toluene. Mercaptosuccinic acid (MSA, 98%, Adamas-beta), maleic anhydride (MA, 99.5%, Adamas-beta), 4-dimethylaminopyridine (DMAP, 98%, Energy Chemical, China), triphosgene (98%, Energy Chemical, China) and CPT (99%, Shanxi Herbchem biotech Co., Ltd, China) were used without further purification. Scandium(III) trifluoromethanesulfonate [Sc(OTf)3] was synthesized according to our previous report.57 All other reagents were purchased from Shanghai Chemical Reagent and used as received.
Measurements
Molecular weight and molecular weight distributions of the two precursors were determined by gel permeation chromatography (GPC). GPC curves were recorded on a Waters Breeze 1525 GPC analysis system with two PL mix-D column and a Waters 2414 RI detector, using tetrahydrofuran (THF) as eluents and polystyrene (PS) as calibration sample at the flow rate of 1.0 mL min−1 at 40 °C. Samples were dissolved in THF at a concentration of 5 g L−1 and filtered prior to analysis. Proton nuclear magnetic resonance (1H NMR) spectra were recorded on a Bruker Avance DMX500 spectrometer in CDCl3 with tetramethylsilane as internal standard. Samples were dissolved in CDCl3 at a concentration of 10 g L−1. Fourier transform infrared (FT-IR) spectra were recorded on a PE Paragon 1000 spectrometer (film or KBr disk). Polymers were dissolved in dichloromethane (DCM) and coated at the KBr disks. Freeze dried hydrogel was prepared as KBr films. UV-Vis spectra were recorded on a UV-2450 UV-Vis spectrophotometer (SHIMADZU). The absorbance spectra of the precursors, CPT–polymer conjugate and CPT were recorded in the range of 200–500 nm. The concentrations of CPT released from hydrogels were determined using an Agilent 1260 high performance liquid chromatographic (HPLC) system. Chromatographic separation was performed on an Agilent ZORBAX SB-C18 column (4.6 × 150 mm, 5 μm) at 30 °C with methanol and 0.1% phosphoric acid aqueous solutions (75/25, v/v) as a mobile phase at a flow rate of 1.0 mL min−1. A wavelength of 365 nm was used to detect the signal of CPT. The concentration of CPT was calculated on the basis of the linear regression equations. Rheological behavior of the samples was characterized on a TA AR-G2 stress-controlled rheometer (TA Instruments, U. S. A.), equipped with a parallel plate of 20 mm in diameter. Elastic (G′) and loss (G′′) moduli of the mixed solution of two precursors were monitored as a function of time and frequency to characterize the viscoelastic properties. The time sweeping tests were carried out for 15 min with 1% strain and 1 rad s−1. Subsequently the frequency sweeping tests were carried out from 0.5 rad s−1 to 50 rad s−1 with 1% strain.
Synthesis of CPT–POEGM conjugate
The CPT–POEGM conjugate was synthesized by two steps. Firstly, POEGM terminated with two hydroxyl groups was synthesized by polycondensation similar to our previous report.46 In brief, OEG (Mn = 600, 36.0 g, 0.06 mol), MA (6.3 g, 0.054 mol) and Sc(OTf)3 (0.29 g, 0.6 mmol) were added into a 250 mL eggplant-shaped bottle immersed in an oil bath preheated to 80 °C under the nitrogen atmosphere with vigorous stirring for esterification for 12 h. Then polycondensation was started at 120 °C with a pressure below 30 mm Hg. After 2 h, the vacuum degree was gradually increased to 0.3–3 mm Hg and maintained for 10 h to complete the polymerization. The crude product was dissolved in DCM and passed through an aluminum column to remove the catalyst. The resultant solution was concentrated and precipitated into cold diethyl ether for three times. The final product was dried in a vacuum oven for 48 hours to constant weight. Yield: 34.8 g (90%). Secondly, triphosgene strategy was used to coupling CPT and POEGM. In brief, CPT (1.0 g, 2.87 mmol) and DMAP (1.12 g, 9.6 mmol) were suspended in 50 mL of dry DCM under argon atmosphere. Triphosgene (0.284 g, 0.96 mmol) was added and the mixture was stirred for 30 min at room temperature. 20.0 g POEGM dissolved in 100 mL of dry DCM was added dropwise via a constant pressure funnel. The reaction mixture was stirred overnight, filtered, washed continuously once with water, twice with 1.0 M HCl, and twice with brine. The organic layer was dried over anhydrous MgSO4, filtered, concentrated on a rotary evaporator and precipitated into cold diethyl ether for three times. The final product was dried in a vacuum oven for 48 hours to constant weight. Yield: 18.3 g (87%).
Synthesis of POEGMS
POEGMS was also synthesized similar to our previous reports.58 In brief, OEG (Mn = 600, 36.0 g, 0.06 mol), MSA (9.0 g, 0.06 mol), and Sc(OTf)3 (0.29 g, 0.6 mmol) were added into a 250 mL eggplant-shaped bottle immersed in an oil bath preheated to 80 °C under the nitrogen atmosphere with vigorous stirring for esterification for 12 h. Then polycondensation was started at 100 °C with a pressure below 30 mm Hg. After 2 h, the vacuum degree was gradually increased to 0.3–3 mm Hg and maintained for 10 h to complete the polymerization. The crude product was dissolved in DCM and passed through an aluminum column to remove the catalyst. The yield was 33.5 g (81%).
Preparation of CPT conjugated hydrogels
CPT conjugated hydrogel with a solid content of 100 g L−1 was prepared as follows: 100 mg of CPT–POEGM was dissolved into PBS solution (0.01 M, pH = 7.4) and fixed the volume to 1.0 mL. Then PBS solution of POEGMS (100 g L−1) with equimolar mercapto group was mixed with the CPT–POEGM solution under 37 °C, to give in situ forming CPT-hydrogel within tens of seconds. Hydrogels with other solid contents were prepared via similar method. The gelation time of hydrogels was determined by the test-tube inverting method.59 No visible flow within 60 s was regarded as the criteria for gel formation when the vial was vertically inverted. A stopwatch was used to record the gelation time.
Swelling tests
Hydrogels for swelling tests were prepared in a PBS solution (0.01 M, pH = 7.4) with a precursor concentration of 100, 200 and 300 g L−1, respectively. Swelling tests were performed by immersing the weighed dry hydrogels (by lyophilization) in PBS solution (0.01 M, pH = 7.4) at 37 °C. At regular time intervals, the swollen hydrogels were weighed after removal of the solution on the surface. The swelling ratio (SR) of the hydrogels was calculated from the equation:
SR (%) = (Wt − W0)/W0 × 100, |
where Wt is the weight of the swollen hydrogel and W0 is the weight of the dry hydrogel.
Degradation studies
1.0 mL of hydrogel was incubated in 10 mL of PBS solution (pH = 7.4, 0.01 M) at 37 °C in vials to assess the hydrolytic degradation. At a pre-defined time degradation medium was taken out and replenished with an equal volume of fresh medium. Masses of dry gels (by lyophilization) were measured at a pre-defined time. The degraded fractions (DF) were calculated as follows:
DF (%) = (W0 − Wt)/W0 × 100, |
where W0 is the mass of original dry gel and Wt is the mass of undegraded dry gel.
In vitro drug release
1.0 mL of CPT conjugated hydrogel was immersed into the release medium (PBS, 10.0 mL, pH = 7.4, 0.01 M, 37 °C). At predetermined time intervals, 3.0 mL of the release medium was collected periodically and replenished with an equal volume of fresh medium. The amount of released CPT was determined by HPLC.
In vitro cytotoxicities of precursors and hydrogels
The cytotoxicities of precursors and hydrogels were evaluated by the Cell Counting Kit-8 (CCK-8) (Dojindo, Japan) assays. All the tests were run five times. The solutions of precursors were prepared in PBS solution (0.01 M, pH = 7.4) and then sterilized by filtration (0.22 μm). 0.2 mL of CPT-hydrogels or blank hydrogel were placed in 2 mL cell-culture medium and soaked at 37 °C for 48 h, respectively. The hydrogels were then removed, and the extracts were stored for the evaluation of cytotoxicities. All extracts were sterilized by filtration (0.22 μm). HepG2 cells (a human liver hepatocellular carcinoma cell, Cell Bank of the Chinese Academy of Science, China) were pre-incubated in a 96-well plate (5 × 103 cells per well) with a culture medium of 10% FBS/α-MEM (Invitrogen Co., Carlsbad, CA) in a humidified 5% CO2 containing atmosphere at 37 °C for 24 h. Then the cells were further incubated with precursor solutions and hydrogel extracts for 48 h. Subsequently, the media was aspirated and replenished with 100 μL of fresh culture medium. 10 μL of CCK-8 reagents were added into each well, and the cells were incubated in the dark for another 1 h. The absorbance at a wavelength of 450 nm of each well was measured using a microplate reader (Sunrise™ Basic, TECAN, Zurich, Switzerland). Non-treated cells were used as a negative control, and wells without cells but culture medium were used as the blank. The relative cell viability (CV) was calculated as follows:
where OD450 samples is the absorbance of the cells treated with gel precursor extracts or hydrogels with different concentrations, OD450 control is the absorbance of the control group and OD450 blank is the absorbance of the well with no cells but culture medium. Data are presented as the average ± SD (n = 5).
Results and discussion
Synthesis and characterization
POEGM terminated with two hydroxyls was synthesized by polycondensation of OEG diol and MA, which was characterized by 1H NMR was shown in Fig. 1A. The peaks at 6.3 (Hb) ppm and 4.4 ppm (Hc) indicate the stability of MA's double bonds and the esterification of OEG by MA. The molar ratio of OEG to MA units in POEGM can be calculated by the integral ratio of Hc to Hb, which is in agreement with the feed ratio. Fig. S1† shows the GPC trace of POEGM, in which a monomodal peak was observed. The relative molecular weight of POEGM was 8100 g mol−1 (PDI = 2.23). These results indicate that POEGM terminated with two hydroxyls was successfully synthesized.
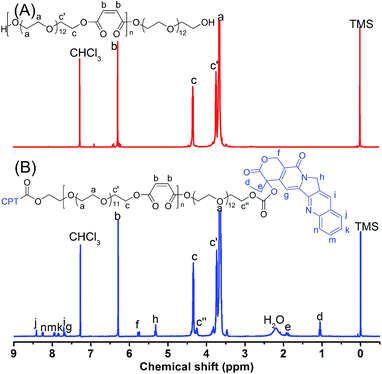 |
| Fig. 1 1H NMR spectra of POEGM (A) and CPT–POEGM (B). | |
CPT–POEGM conjugate was synthesized by a triphosgene strategy. The 1H NMR spectrum of CPT–POEGM conjugate was displayed in Fig. 1B. The signal at 4.25 ppm (Hc′′) demonstrated that CPT has been conjugated to the end of POEGM by carbonic ester bond. The characteristic peaks of CPT were at 1.02 (Hd), 1.90 (He), 5.32 (Hh), 5.78 (Hf), 7.67 (Hg), 7.70 (Hi), 7.83 (Hk), 7.96 (Hm), 8.26 (Hn), 8.43 (Hj) ppm, which showed correct integrated area according to the chemical structure of CPT. The content of CPT in the conjugate was 1.29 wt%, calculated by the integral ratio of Hb to Hd. After conjugation, the GPC trace of CPT–POEGM (Fig. S1†) only slightly shifted, which can be attributes to the fact that the coupling of CPT on the POEGM chain end hardly influenced the volume of molecular coil in THF.
POEGMS was synthesized by polycondensation of OEG diol and MSA. The molecular weight of POEGMS was 9100 g mol−1 with a molecular weight distribution of 1.97 determined by GPC (Fig. S2†). Fig. S3† shows the 1H NMR spectrum of POEGMS. The characteristic signal at 2.2 ppm demonstrates the presence of thiol groups, which strongly suggests that the thiols were stable during the polycondensation process. Additionally, the signal of PEG end hydroxymethyl, which was esterified by MSA [–CH2CH2OCOCH(SH)CH2CO–], was detected at 4.30 ppm.
The transparent aqueous solution of CPT–POEGM conjugate emitted bright blue fluorescence under the UV irradiation excited at 365 nm (Fig. 2), which confirmed that water solubility of CPT has been greatly improved, and there is no aggregation of CPT moieties in the solution. Meanwhile, no absorption at 365 nm was recorded at the UV-Vis spectra of POEGM and POEGMS, as shown in Fig. 2.
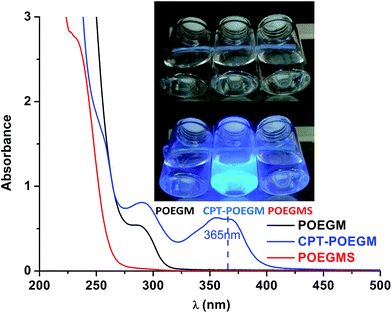 |
| Fig. 2 UV-vis spectra and digital images of POEGM, CPT–POEGM and POEGMS in aqueous solution. The up photograph was taken under room light and the down photograph was taken under UV lamp at 365 nm. The concentration of the polymers is 1 g L−1. | |
Preparation and characterization of CPT conjugated hydrogels
The Michael-type thiol-ene “click” reaction involves the direct addition of a dissociated thiolate nucleophile onto an unsaturated carbon double bond, activated by a neighboring electron withdrawing group such as an ester or amide, which can be carried out under mild conditions that are similar to human physiological conditions without the production of free radicals.60–62 In this study, the gelation process of CPT–POEGM conjugate and POEGMS with equal mole number of thiols and enes was monitored under different concentrations of precursors by the tube-inversion method. The gelation conditions are similar to human physiological conditions, namely 37 °C and pH 7.4. The gelation process was rapid and the gelation time of hydrogels with 100, 200 and 300 g L−1 solid content is 87 ± 4, 69 ± 2 and 44 ± 2 s respectively, as shown in Fig. 3. The instant gelation demonstrates the high reactivity of thiol-ene “click” reaction, which is conducive to avoid the diffusion of CPT–POEGM conjugate to the surrounding healthy tissues after the injecting the solution into the tumor site.63 Furthermore, FT-IR spectra (Fig. S4†) clearly state the high conversion of thiol-ene reaction under physiological conditions. After the gelation, the absorption peaks ascribed to the double bonds (1637 cm−1) and thiol groups (2540 cm−1) in the spectrum of hydrogel were hardly detected.
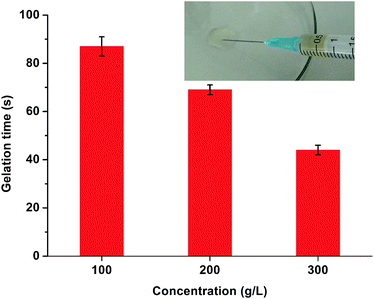 |
| Fig. 3 Gelation time of CPT-hydrogels with 100, 200, and 300 g L−1 solid content under physiological conditions. The insert photograph is hydrogel injected from syringe. | |
The moduli of CPT-hydrogels were determined using an AR-G2 stress-controlled rheometer. Time scanning (Fig. 4A) was first conducted to observe the gelation process. Injectable hydrogels are usually in situ formed from hydrosol (solution of precursors), and the rheological property of hydrosol is resemble to liquid whose storage modulus G′ is lower than loss modulus G′′. After the sol–gel transition, hydrogels are formed and the rheological property of hydrogel is resemble to solid with greater storage modulus G′ than loss modulus G′′. The crossover point of G′ and G′′ is defined to the gelation point, and the corresponding time is the gelation time.64 Unfortunately, no gelation point was recorded on the curve of rheology because of the high reactivity of thiol-ene “click” reaction. Hydrogels were rapidly formed within tens of seconds, the moduli of hydrogels approached plateau values rapidly. The final moduli of hydrogels ranged from 2.1 kPa to 51 kPa depending on the initial concentration of precursors. Moreover, for the resultant hydrogels, all G′ values showed a weak dependence on frequency (Fig. 4B), which further confirmed that the hydrogels were well cross-linked with insignificant hydrosol fraction.
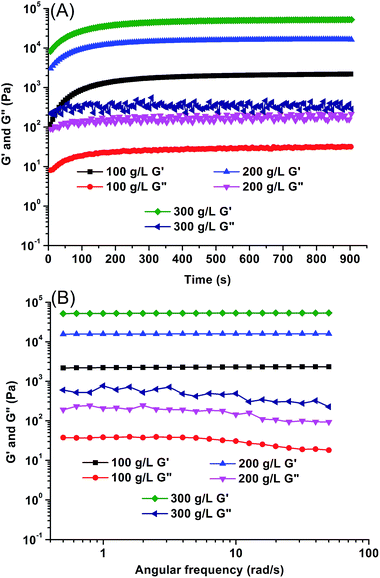 |
| Fig. 4 (A) Effect of initial concentration of precursors on gelation. Test conditions: 1.0 rad s−1 frequency, 1.0% strain, 37 °C. (B) Frequency dependence of hydrogels with 100, 200 and 300 g L−1 solid content. Test conditions: 1.0% strain, 37 °C. | |
The swelling ratio is an important parameter for evaluating the performance of hydrogels. In this study, the water absorption capability of the lyophilized hydrogels was determined by a gravimetric method. Curves of swelling ratios as a function of time for the lyophilized hydrogels are presented in Fig. S5.† All of the hydrogels with different solid content reached equilibrium swelling within 12 h. Due to more perfect network, swelling ratio of hydrogels with 300 g L−1 solid content is lower than those of other hydrogels.
In this study, highly hydrophobic CPT was bonded to POEGM with carbonic ester bond and resultant water-soluble CPT–POEGM conjugate can be in situ crosslinked via thiol-ene “click” reaction under physiological conditions. Strong bright blue fluorescence of obtained CPT-hydrogels under UV lamp excited at 365 nm further confirms the homogeneity of CPT loading, as shown in Fig. 5. The CPT loading quantity of hydrogels can be calculated by the followed equation:
where
MCgel is the CPT loading mass of CPT-hydrogels (mg),
Rprodrug is the CPT mass ratio in CPT–POEGM (%),
C is the concentration of CPT–POEGM aqueous solution (g L
−1),
V is the volume of CPT–POEGM aqueous solution (mL).
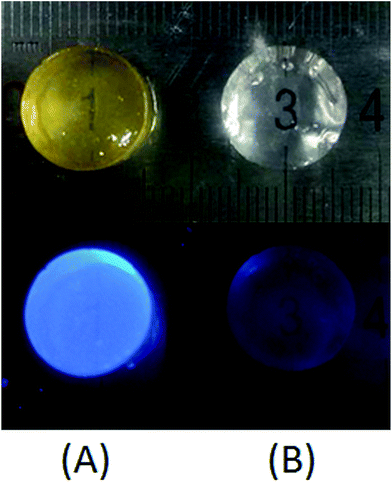 |
| Fig. 5 Photographs of (A) CPT-hydrogel and (B) blank hydrogel. The up photograph was taken under room light and the down photograph was taken under UV lamp excited at 365 nm, the solid content of hydrogels is 300 g L−1. | |
In vitro degradation and CPT release profile
Injectable hydrogels loaded with anticancer drug for intratumor drug delivery should be degradable after the loaded drug was released completely. In this work, degradable PEG derivatives with ester bonds were chosen as the framework of the hydrogels, which can be degraded through the hydrolysis of ester bonds. Highly hydrophobic CPT was conjugated to gel matrix by the degradable carbonic ester bond, which is very similar to ester bond. Profiles of in vitro degradation and release are shown in Fig. 6. Degradation periods of CPT-hydrogels with 100, 200 and 300 g L−1 solid content were 7, 11 and 16 days respectively. Hydrogels with high solid content had longer degradation period due to their more compact network and smaller pore structure than hydrogels with low solid content. Meanwhile the CPT release rates also depend on the solid content. For example, at the fourth day, more than 50% loaded CPT was released from the hydrogel with 100 g L−1 solid content, and only about 35% loaded CPT was released from depots with 300 g L−1 solid content within the same period. Notably, the release profiles showed that no significant initial burst release was observed. The completion time of CPT release from hydrogels with 100, 200 and 300 g L−1 solid content was about 7, 11 and 16 days respectively, which is highly consistent with the degradation time. This phenomenon can be attributed to the similarity of carbonic ester bond and ester bond. The processes of CPT release and hydrogel degradation were simultaneous. In most cases concerning on drug-loaded hydrogels, drug was released rapidly over few hours to a couple of days, and hydrogel was degraded much slowly. The undegraded blank hydrogel without drug is useless for cancer treatment. Furthermore, it can even re-load the drug released from the newly injected hydrogels, which will discount the drug concentration in the tumor site and lower the efficiency of chemotherapy. This study has achieved the simultaneity of drug release and gel degradation, which has potential for highly efficient cancer localized treatment.
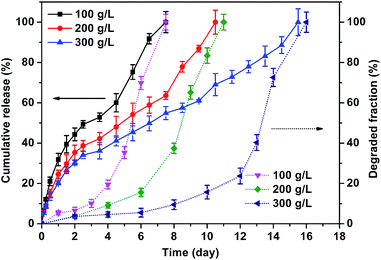 |
| Fig. 6 In vitro degradation and release profiles of CPT-hydrogels with solid contents of 100, 200 and 300 g L−1 under pH = 7.4 at 37 °C. | |
In vitro cytotoxicity
Cell viabilities in the presence of precursors and hydrogel extracts were evaluated by CCK-8 assay. HepG2 cells were incubated in various concentrations of POEGM, POEGMS and CPT–POEGM for 48 h. As shown in Fig. 7A, no obvious cytotoxicity on the growth inhibition of HepG2 cells was observed, even when the concentration of POEGMS and POEGM was very high (10 g L−1). Meanwhile, CPT–POEGM conjugate showed significant cytotoxicity to HepG2 cells. Even at a concentration of 0.1 g L−1, less than 30% cells were living. This result evidenced that the conjugated CPT can be degraded from framework of hydrogel to kill cancer cells. Afterwards, we further test the cytotoxicity of CPT-hydrogels in vitro. As displayed in Fig. 7B, after incubation with HepG2 cells for 48 h, the extracts of CPT-hydrogels exhibited significant cytotoxicity. The cytotoxicity of CPT-hydrogels with 300 g L−1 solid content is higher than those of hydrogels with 200 and 100 g L−1 solid content due to the high concentration of loaded and released CPT. These results indicate that this kind of CPT conjugated hydrogels with simultaneous release and degradation could be a potential hydrophobic drug carrier for local treatment of tumor.
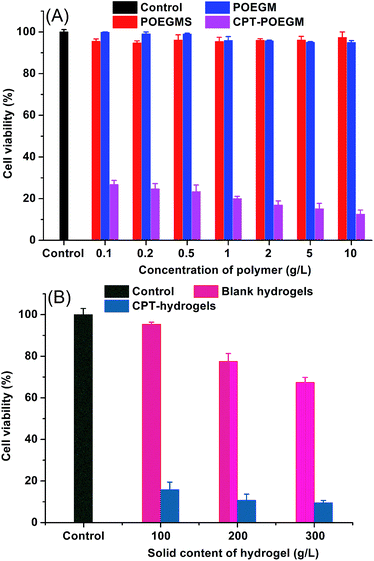 |
| Fig. 7 (A) Relative cell viabilities of HepG2 cells with precursors and CPT–POEGM, the concentration of precursors is from 0.1 to 10 g L−1, the concentration of conjugated CPT in solution is from 1.29 to 12.9 μg mL−1; (B) relative cell viabilities of HepG2 cells in the presence of CPT-hydrogel extracts. | |
Conclusions
In conclusion, we have developed a kind of novel injectable CPT conjugated hydrogels for potential local chemotherapy of solid tumor. Highly hydrophobic CPT was conjugated to the chain end of a PEG derivative containing multiple maleates in the backbone, which was in situ crosslinked by another PEG derivative with numerous thiols through thiol-ene “click” reaction under physiological conditions. Simultaneous drug release and degradation behaviors were observed, and the two processes continued for one week to 16 days, depending on the solid content of the hydrogels. In vitro cell viability assays showed that this kind of CPT conjugated hydrogels had significant cytotoxicity to HepG2 cells, which could be a potential intratumor drug carrier for localized chemotherapy.
Acknowledgements
The authors are grateful to the financial supports from the National Natural Science Foundation of China (21274121, 21674094 and 51603180), the China Postdoctoral Science Foundation (2015M580517), the Fundamental Research Funds for the Central Universities (2015QNA4036) and the Key Project of Natural Science Foundation of Zhejiang Province (LZ16H140001).
Notes and references
- B. D. Weinberg, E. Blanco and J. M. Gao, J. Pharm. Sci., 2008, 97, 1681–1702 CrossRef CAS PubMed.
- A. Fakhari and J. Anand Subramony, J. Controlled Release, 2015, 220, 465–475 CrossRef CAS PubMed.
- K. B. Wallace, Pharmacol. Toxicol., 2003, 93, 105–115 CAS.
- J. Crawford, D. C. Dale and G. H. Lyman, Cancer, 2004, 100, 228–237 CrossRef PubMed.
- Y. Brudno and D. J. Mooney, J. Controlled Release, 2015, 219, 8–17 CrossRef CAS PubMed.
- A. A. Exner and G. M. Saidel, Expert Opin. Drug Delivery, 2008, 5, 775–788 CrossRef CAS PubMed.
- S. Kim, J.-H. Kim, O. Jeon, I. C. Kwon and K. Park, Eur. J. Pharm. Biopharm., 2009, 71, 420–430 CrossRef CAS PubMed.
- R. De Souza, P. Zahedi, C. J. Allen and M. Piquette-Miller, Drug Delivery, 2010, 17, 365–375 CrossRef CAS PubMed.
- C. J. Kearney and D. J. Mooney, Nat. Mater., 2013, 12, 1004–1017 CrossRef CAS PubMed.
- B. Jeong, Y. H. Bae, D. S. Lee and S. W. Kim, Nature, 1997, 388, 860–862 CrossRef CAS PubMed.
- J. B. Wolinsky, Y. L. Colson and M. W. Grinstaff, J. Controlled Release, 2012, 159, 14–26 CrossRef CAS PubMed.
- S. Kempe and K. Mäder, J. Controlled Release, 2012, 161, 668–679 CrossRef CAS PubMed.
- H. J. Moon, D. Y. Ko, M. H. Park, M. K. Joo and B. Jeong, Chem. Soc. Rev., 2012, 41, 4860–4883 RSC.
- M. Konishi, Y. Tabata, M. Kariya, A. Suzuki, M. Mandai, K. Nanbu, K. Takakura and S. Fujii, J. Controlled Release, 2003, 92, 301–313 CrossRef CAS PubMed.
- C. D. Pritchard, T. M. O'Shea, D. J. Siegwart, E. Calo, D. G. Anderson, F. M. Reynolds, J. A. Thomas, J. R. Slotkin, E. J. Woodard and R. Langer, Biomaterials, 2011, 32, 587–597 CrossRef CAS PubMed.
- Y. Li, J. Rodrigues and H. Tomas, Chem. Soc. Rev., 2012, 41, 2193–2221 RSC.
- Y. L. Cheng, C. L. He, J. X. Ding, C. S. Xiao, X. L. Zhuang and X. S. Chen, Biomaterials, 2013, 34, 10338–10347 CrossRef CAS PubMed.
- J. B. Shi, W. Guobao, H. L. Chen, W. Zhong, X. Z. Qiu and M. M. Q. Xing, Polym. Chem., 2014, 5, 6180–6189 RSC.
- L. Xiong, Q. J. Luo, Y. Wang, X. D. Li, Z. Q. Shen and W. P. Zhu, Chem. Commun., 2015, 51, 14644–14647 RSC.
- K. Nagahama, D. Kawano, N. Oyama, A. Takemoto, T. Kumano and J. Kawakami, Biomacromolecules, 2015, 16, 880–889 CrossRef CAS PubMed.
- G. Chang, T. Ci, L. Yu and J. Ding, J. Controlled Release, 2011, 156, 21–27 CrossRef CAS PubMed.
- H. T. Ta, C. R. Dass and D. E. Dunstan, J. Controlled Release, 2008, 126, 205–216 CrossRef CAS PubMed.
- T. R. Hoare and D. S. Kohane, Polymer, 2008, 49, 1993–2007 CrossRef CAS.
- N. Shpaisman, L. Sheihet, J. Bushman, J. Winters and J. Kohn, Biomacromolecules, 2012, 13, 2279–2286 CrossRef CAS PubMed.
- L. Gao, X. Li, Y. Wang, W. Zhu, Z. Shen and X. Li, J. Polym. Sci., Part A: Polym. Chem., 2016, 54, 2651–2655 CrossRef CAS.
- M. E. Wall, M. C. Wani, C. E. Cook, K. H. Palmer, A. T. McPhail and G. A. Sim, J. Am. Chem. Soc., 1966, 88, 3888–3890 CrossRef CAS.
- Y. Pommier, Nat. Rev. Cancer, 2006, 6, 789–802 CrossRef CAS PubMed.
- V. J. Venditto and E. E. Simanek, Mol. Pharm., 2010, 7, 307–349 CrossRef CAS PubMed.
- P. Botella, I. Abasolo, Y. Fernandez, C. Muniesa, S. Miranda, M. Quesada, J. Ruiz, S. Schwartz Jr and A. Corma, J. Controlled Release, 2011, 156, 246–257 CrossRef CAS PubMed.
- F. M. Veronese and J. M. Harris, Adv. Drug Delivery Rev., 2008, 60, 1–2 CrossRef CAS.
- P. F. Gou, W. P. Zhu and Z. Q. Shen, Biomacromolecules, 2010, 11, 934–943 CrossRef CAS PubMed.
- P. F. Gou, W. P. Zhu and Z. Q. Shen, Polym. Chem., 2010, 1, 1205–1214 RSC.
- S. N. S. Alconcel, A. S. Baas and H. D. Maynard, Polym. Chem., 2011, 2, 1442–1448 RSC.
- W. P. Zhu, M. J. Zhong, W. W. Li, H. C. Dong and K. Matyjaszewski, Macromolecules, 2011, 44, 1920–1926 CrossRef CAS.
- K. Zhang, Y. Wang, W. P. Zhu, X. D. Li and Z. Q. Shen, J. Polym. Sci., Part A: Polym. Chem., 2012, 50, 2045–2052 CrossRef CAS.
- Y. Wang, H. Du, L. Gao, H. Ni, X. Li, W. Zhu and Z. Shen, Polym. Chem., 2013, 4, 1657–1663 RSC.
- G. Xu, X. Wang, C. Deng, X. Teng, E. J. Suuronen, Z. Shen and Z. Zhong, Acta Biomater., 2015, 15, 55–64 CrossRef CAS PubMed.
- E. M. Pelegri O'Day, E. W. Lin and H. D. Maynard, J. Am. Chem. Soc., 2014, 136, 14323–14332 CrossRef PubMed.
- C. Hiemstra, W. Zhou, Z. Zhong, M. Wouters and J. Feijen, J. Am. Chem. Soc., 2007, 129, 9918–9926 CrossRef CAS PubMed.
- L. Zhang, Y. I. Jeong, S. Zheng, D. H. Kang, H. Suh and I. Kim, Macromol. Biosci., 2014, 14, 401–410 CrossRef CAS PubMed.
- L. Chen, X. Q. Li, L. P. Cao, X. L. Li, J. R. Meng, J. Dong, L. Yu and J. D. Ding, Chin. J. Polym. Sci., 2016, 34, 147–163 CrossRef CAS.
- H. Du, G. Y. Zha, L. L. Gao, H. Wang, X. D. Li, Z. Q. Shen and W. P. Zhu, Polym. Chem., 2014, 5, 4002–4008 RSC.
- R. Sun, Q. Luo, C. Gao, Y. Wang, L. Gao, H. Du, Y. Huang, X. Li, Z. Shen and W. Zhu, Polym. Chem., 2014, 5, 4879–4883 RSC.
- H. Wang, G. Y. Zha, H. Du, L. L. Gao, X. D. Li, Z. Q. Shen and W. P. Zhu, Polym. Chem., 2014, 5, 6489–6494 RSC.
- H. Du, Y. Wang, X. Yao, Q. Luo, W. Zhu, X. Li and Z. Shen, Polym. Chem., 2016, 7, 5620–5624 RSC.
- L. L. Gao, G. Y. Zha, Y. Wang, Q. J. Luo, W. P. Zhu, Z. Q. Shen and X. D. Li, Polym. Chem., 2015, 6, 8240–8243 RSC.
- Y. Shen, E. Jin, B. Zhang, C. J. Murphy, M. Sui, J. Zhao, J. Wang, J. Tang, M. Fan, E. Van Kirk and W. J. Murdoch, J. Am. Chem. Soc., 2010, 132, 4259–4265 CrossRef CAS PubMed.
- V. Delplace, P. Couvreur and J. Nicolas, Polym. Chem., 2014, 5, 1529–1544 RSC.
- Y. Wang, Q. Luo, R. Sun, G. Zha, X. Li, Z. Shen and W. Zhu, J. Mater. Chem. B, 2014, 2, 7612–7619 RSC.
- Y. Wang, Q. Luo, L. Gao, C. Gao, H. Du, G. Zha, X. Li, Z. Shen and W. Zhu, Biomater. Sci., 2014, 2, 1367–1376 RSC.
- T. Plyduang, L. Lomlim, S. Yuenyongsawad and R. Wiwattanapatapee, Eur. J. Pharm. Biopharm., 2014, 88, 351–360 CrossRef CAS PubMed.
- W. P. Zhu, L. Xiong, H. Wang, G. Y. Zha, H. Du, X. D. Li and Z. Q. Shen, Polym. Chem., 2015, 6, 7097–7099 RSC.
- H. R. Wang, J. L. He, D. L. Cao, M. Z. Zhang, F. Li, K. C. Tam and P. H. Ni, Polym. Chem., 2015, 6, 4809–4818 RSC.
- F. Li, J. L. He, M. Z. Zhang and P. H. Ni, Polym. Chem., 2015, 6, 5009–5014 RSC.
- C. Di Meo, F. Cilurzo, M. Licciardi, C. Scialabba, R. Sabia, D. Paolino, D. Capitani, M. Fresta, G. Giammona, C. Villani and P. Matricardi, Pharm. Res., 2015, 32, 1557–1569 CrossRef CAS PubMed.
- Y. Wang, Q. J. Luo, W. P. Zhu, X. D. Li and Z. Q. Shen, Polym. Chem., 2016, 7, 2665–2673 RSC.
- H. Du, L. L. Gao, W. P. Zhu and Z. Q. Shen, Chin. Chem. Lett., 2012, 23, 879–882 CrossRef CAS.
- W. P. Zhu, L. L. Gao, Q. J. Luo, C. Gao, G. Y. Zha, Z. Q. Shen and X. D. Li, Polym. Chem., 2014, 5, 2018–2026 RSC.
- L. Yu, T. Y. Ci, S. C. Zhou, W. J. Zeng and J. D. Ding, Biomater. Sci., 2013, 1, 411–420 RSC.
- C. E. Hoyle and C. N. Bowman, Angew. Chem., Int. Ed., 2010, 49, 1540–1573 CrossRef CAS PubMed.
- D. P. Nair, M. Podgórski, S. Chatani, T. Gong, W. Xi, C. R. Fenoli and C. N. Bowman, Chem. Mater., 2013, 26, 724–744 CrossRef.
- Q. Song, Z. Luo, X. M. Tong, Y. Du and Y. B. Huang, Chin. J. Polym. Sci., 2014, 32, 1003–1009 CrossRef CAS.
- K. K. Nishi and A. Jayakrishnan, Biomacromolecules, 2007, 8, 84–90 CrossRef CAS PubMed.
- C. X. Ding, L. L. Zhao, F. Y. Liu, J. Cheng, J. X. Gu, S. Dan, C. Y. Liu, X. Z. Qu and Z. Z. Yang, Biomacromolecules, 2010, 11, 1043–1051 CrossRef CAS PubMed.
Footnotes |
† Electronic supplementary information (ESI) available. See DOI: 10.1039/c6ra20691c |
‡ L. L. Gao and Y. D. Chen contributed equally to this work. |
|
This journal is © The Royal Society of Chemistry 2016 |
Click here to see how this site uses Cookies. View our privacy policy here.