DOI:
10.1039/C6RA16020D
(Paper)
RSC Adv., 2016,
6, 94651-94660
Si-Doped single-walled carbon nanotubes interacting with isoniazid-a density functional and molecular docking study†
Received
20th June 2016
, Accepted 17th September 2016
First published on 19th September 2016
Abstract
The interaction of antitubercular drug isoniazid (INH), with pristine and Si-doped (5,5) and (9,0) single-walled carbon nanotubes (SWNTs) have been reported. The introduction of Si-dopant facilitates adsorption of INH on the otherwise inert nanotubes, as observed from adsorption energies, charge transfer, and global reactivity descriptor values. Compared to covalent functionalization, noncovalent functionalization is more facile and proffers greater mobility for INH with the tube sidewall, and parallel adsorption of INH exhibits enhanced charge transfer compared to perpendicular orientation of adsorption. Docking studies on INH, and INH-functionalized SWNTs with wild and mutant types of InhA protein, provide valuable insights on INH drug delivery aspects mediated by SWNTs.
Introduction
The chemical functionalization (modification) of single-walled carbon nanotubes is one of the most effective routes to modify chemical, structural, and electronic properties, which can induce localized changes within the nanotube framework and expand its potential application. Some of the effective methods involve1 substitutional doping of heteroatoms like boron and nitrogen,2 adsorption of small organic molecules along the nanotube sidewall,3 interfacial defects within the nanotube, e.g. Stone–Wales (SW) defect, line defect, and insertion of guest atoms and molecules inside CNTs.1–4
In biomedical research, the incorporation of SWNTs as delivery modules for therapeutic drugs and bio-conjugates necessitates the active release of therapeutic agent within the target site, for which a proper means of functionalization is a prerequisite.5–7 In this regard, noncovalent functionalization is well foreseen, as it does not involve chemical deformation in the nanotube structure, but induces significant interaction mediated through π–π stacking and/or van der Waals interaction.8,9 Small aromatic organic molecules,10 vitamins,11 polymers,12 porphyrins,13 and metallocenes14,15 constitute the widely studied molecules that noncovalently interact with nanotube sidewall and modify its electronic and structural properties.16,17
In the case of substitutional doping of SWNTs by boron and/or nitrogen atoms, the percentage and nature of adsorbed dopant atom strongly influence the electronic properties. Although doping of SWNTs with boron and nitrogen atoms is widely reported,18–21 Si doping,22,23 on the other hand, is less predominant and not well documented in the literature.24,25 The Si atom has a similar electronic configuration to carbon and, under high temperature and pressure, it exhibits better properties compared to carbon. Doping of Si is an effective way to foster nanotube electronic properties and chemical reactivity towards the adsorbing molecules.26 Si-Doped nanotubes have been studied for novel nanoelectronic devices, such as chemical sensors, and nanobiosensors.27,28 Guo et al.29 studied the structural properties and reactivity of Si-doped SWNTs towards the adsorption of small molecules like CO, H2O, and H2CO, in gas sensing devices. Froudakis and co-workers performed detailed studies on the structure and electronic properties of Si-doped SWNTs to understand the extent of the Si doping concentration in modulating nanotube electronic properties.30
The functionalization of SWNTs has been successfully studied for the delivery of anticancer drugs31–34 and antitubercular drugs like INH,35,36 pyrazinamide (PZA),37 and 2-methylheptyl isonicotinate (MHI).38 Tuberculosis (TB) is caused by the bacterium Mycobacterium tuberculosis and, according to the 2010 consensus conducted by the World Health Organization (WHO), TB incidence and prevalence are estimated at 8.8 and 12 million cases, of which 1.1 million among HIV-negative people and 0.35 million among HIV-positive people have died from TB.39,40 Isonicotinic acid hydrazide, or INH, belongs to the class of first-line antitubercular drugs that has been in clinical practice41 since the 1950s, and is usually administered in combination with PZA, rifampicin, and ethambutol.42 INH is a prodrug, activated by M. tuberculosis catalase peroxidase enzyme (KatG),43,44 which interferes with the biosynthesis of mycolic acid (a major constituent of the fatty acid components of the mycobacterial cell wall). KatG catalyzes the conversion of MnII to MnIII and the MnIII ion facilitates the oxidation of INH.45 Incorporation of SWNTs as a carrier module for INH can facilitate the target-specific drug loading and delivery at the active binding site, and prevents premature degradation of INH upon administration within the body. Fagan and co-workers investigated the potential application of Si-doped SWNTs for the loading and interaction of the anti-inflammatory drug, nimesulide, using ab initio DFT simulations.46 The study showed that nimesulide undergoes strong interaction with Si-doped nanotubes, followed by subsequent modification of the electronic and structural properties of the nanotubes.
Motivated by Si-doped capped SWNTs finding potential application in drug delivery, we carried out density functional and molecular docking studies on preferential interaction of INH with perfect and Si-doped SWNTs through covalent and noncovalent functionalization approaches. We considered both periodic and cluster models of nanotube/INH system to simulate the effect of an adsorbed INH molecule on the electronic properties of the two nanotubes. Subsequently, the study is extended towards investigating the interaction of SWNT/INH conjugate with both wild and mutant proteins, which act as target receptors for INH. Docking endows a detailed insight into the selective binding of INH with the protein and secondly, the effectual role of SWNTs in instigating the active release of INH.
Computational details
DFT methodology
The electronic structure calculations are performed with the DMol3 program, which uses the all-electron linear combination of atomic orbital DFT approach. For the cluster models, non-spin-polarized gas-phase calculations are performed for INH, pristine armchair (5,5) SWNTs comprising 4 unit cells (10.465 Å in length and 6.87 Å in diameter), and zigzag (9,0) SWNTs comprising 3 unit cells (13.549 Å in length and 7.05 Å in diameter) with the edges passivated by hydrogen to saturate the dangling bonds. The substitutional doping of (5,5) and (9,0) SWNTs involves replacing a carbon atom with a Si atom.
In the periodic models of (5,5) and (9,0) SWNTs, similar unit cells of the nanotubes are considered, and to eliminate the lateral interaction between the periodic images, cubic supercells of dimensions 20 × 20 × 9.843 Å3 for (5,5) SWNTs and 20 × 20 × 13.100 Å3 for (9,0) SWNTs are considered. The Brillouin zone is sampled by 1 × 1 × 5 k-point using the Monkhorst–Pack scheme47 for the geometry optimization, and a higher Monkhorst pack k-grid of 1 × 1 × 10 k-points is used, along with a thermal smearing value of 0.01 Ha, for calculating the DOS and PDOS. The double numerical basis set with polarization function (DNP) equivalent to the 6-31G** basis set of the Gaussian basis set,48 and Perdew Wang 91 (PW91) exchange correlation functional within the generalized gradient approximation (GGA) are used.
The covalent functionalization of INH was facilitated through the Si dopant atom (reactive adsorption site), with elimination of one hydrogen atom from the amine group of INH. The adsorption energy of INH onto pristine/Si-doped (5,5) and (9,0) SWNTs, via noncovalent and covalent functionalization, are calculated using the following equations:
|
Eads = (EINH + ESWNT/Si-doped SWNT) − Etotal
| (1) |
where
EINH is the total energy of INH,
ESWNT/Si-doped SWNT is the total energy of the SWNT/Si-doped SWNT, and
Etotal is the total energy of the system.
The Eads for covalent functionalization is defined as
|
Eads = (EINH(deprotonated) + ESi-doped SWNT) − Etotal
| (2) |
where
EINH(deprotonated) is the total energy of deprotonated INH molecule,
ESi-doped SWNT is the total energy of Si-doped (5,5) and (9,0) SWNTs, and
Etotal is the total energy of the system for covalent functionalization.
The DFT-GGA functional has shortcomings in terms of accounting for weak noncovalent van der Waals (vdW) interactions, as it is unable to describe the attractive dispersion interactions that decay with R−6 at large intermolecular distances.49 Thus, inclusion of Grimme's dispersion correction term (GD2) can reasonably describe noncovalent interactions and help to capture the essence of the energetics in conjugated systems.50 The noncovalent π–π stacking interaction was treated using the semi-empirical dispersion corrections to DFT developed by Grimme (D2),51,52 with the GAUSSIAN09 code.53 Here, pristine and Si-doped (5,5) SWNT systems were considered for the dispersion calculations using the Perdew, Burke, and Ernzerhof (PBE) functional and 6-31G(d,p) basis set with inclusion of Grimme's GD2 correction,51 and the corresponding adsorption energies of the conjugates were calculated using eqn (1) and (2), as discussed above.
The quantum molecular descriptors, namely global hardness (η), chemical potential (μ), and electrophilicity indices (ω), which are helpful to interpret the reactivity and/or stability of the systems, are calculated as follows. In an N-electron system with total energy (E) and external potential ν(
), the electronegativity (χ) is given by:
|
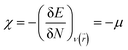 | (3) |
where
μ is defined as the negative of electronegativity. The
η is expressed in terms of the second derivative of energy with respect to the external potential
ν(
![[r with combining right harpoon above (vector)]](https://www.rsc.org/images/entities/i_char_0072_20d1.gif)
) and is given by:
|
 | (4) |
According to Mulliken,54 the working equations for μ and η using the finite difference method are given by:
|
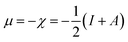 | (5) |
and
|
 | (6) |
where
I is the ionization potential and
A the electron affinity of the molecule.
The electrophilicity index defined by Parr55 is given as:
|
 | (7) |
The electrophilicity index is a measure of the electrophilicity of an electrophile; the higher the electrophilicity of a molecule, the greater is its electrophilic character.
Molecular docking
Molecular docking analysis can help in: (1) characterization of the binding cavity of the protein, (2) orientation of ligand with respect to the receptor protein, and (3) interaction of protein with functionalized SWNTs. The crystal structure of wild and mutant-type M. tuberculosis catalase-peroxidase (mtCP) KatG enzyme is retrieved from the protein data bank (PDB 1SJ2 and 2CCD). The docking is performed using Molegro Virtual Docker (MVD) for active binding of the SWNT/INH onto the protein. MolDock is based on a new hybrid search algorithm, called glided differential evolution, which combines the differential evolution optimization technique with a cavity prediction algorithm.56 The docking score function is an extension of the piecewise linear potential (PLP),57 which includes the H-bonding and electrostatic terms, and the re-rank scoring function identifies the most promising docking solution, further increasing the docking accuracy. For each of the complexes, we performed 50 independent runs, with each of the runs returning one solution (pose) in addition to docking scoring function terms, sp2–sp2 torsion, and Lennard-Jones 12–6 terms.58 The best docked conformation was selected based on MolDock score and re-rank score values and visualized using Molegro Virtual Viewer and Chimera software. We performed docking simulations on INH-functionalized pristine and Si-doped (5,5) SWNTs with both wild and mutant-type proteins. The population size, maximum interactions, scaling factor, and crossover rate are set to 150, 1000, 0.50, and 0.90, respectively. Glided differential evolution and tripos force-field-based docking scoring functions are used to search for the binding orientation and conformation of INH molecule and INH-functionalized SWNT systems.
Results and discussion
Geometries of INH, pristine and Si-doped SWNTs
The optimized geometries of INH, pristine and Si-doped (5,5) and (9,0) SWNTs are depicted in Fig. 1.
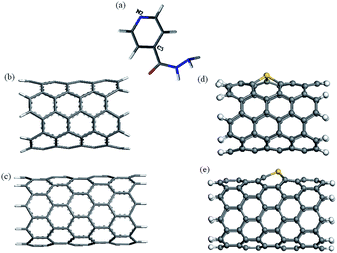 |
| Fig. 1 Optimized geometries of (a) INH, (b) (5,5) SWNT, (c) (9,0) CNT, (d) Si-doped (5,5) SWNT (e) Si-doped (9,0) SWNT. | |
INH is a non-planar molecule with the amine group protruding out of the molecular plane, as shown in Fig. 1a. The calculated bond lengths and bond angles for INH are in good agreement with reported X-ray crystallographic data.59 The C–C bond lengths in pristine (5,5) and (9,0) SWNTs are calculated as 1.42 Å, which corresponds to sp2 hybridization and is concurrent with the experimental value of 1.44 Å.60 Si doping in (5,5) SWNT increases the C–Si bond length to 1.514 Å, whereas in (9,0) SWNT, it increases to 1.517 Å, with the Si atom protruding out of plane (Fig. 1d and e). Si doping breaks the delocalization in pristine nanotubes, with a loss in symmetry at the site of substitution, as was observed in previous DFT reports.61,62
INH-functionalized SWNTs
The two possible orientations of INH along pristine and Si-doped (5,5) and (9,0) SWNTs are investigated: parallel and perpendicular (see Fig. 2 and ESI, Fig. S1†), along with covalent functionalization of INH onto Si-doped (5,5) and (9,0) SWNTs.
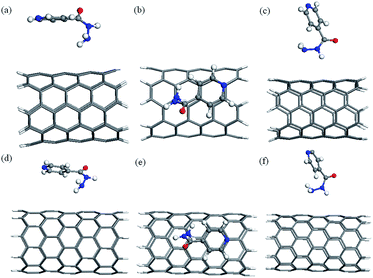 |
| Fig. 2 Noncovalent functionalization of INH onto (5,5) and (9,0) SWNTs; (a) side view of INH/(5,5) SWNT at hollow site (parallel orientation), (b) front view, (c) side view of INH/(5,5) SWNT with perpendicular orientation, (d) side view of INH/(9,0) SWNT at hollow site (parallel orientation), (e) front view, (f) perpendicular adsorption of INH/(9,0) SWNT. | |
In the parallel orientation of INH, interaction is manifested through the π-stacking between the nanotube sidewall and INH, in addition to interaction via the amine group. For INH/(5,5) SWNT (Fig. 2a and b) and INH/(9,0) SWNT (Fig. 2d and e), INH is adsorbed at hollow site at optimum interaction distances of 2.98 and 3.07 Å, respectively. In INH/(5,5) SWNT, the parallel orientation is energetically preferred over perpendicular conformation due to maximum orbital overlap. The perpendicular and parallel orientations of the INH/(5,5) SWNT are degenerate in energy, whereas for INH/(9,0) SWNT, the adsorption energy is 0.073 and 0.064 eV for the parallel and perpendicular adsorption sites (ESI, Table S1†).
The introduction of Si dopant influences the adsorption of INH in the otherwise inert nanotube, leading to significant enhancement in adsorption energy and a lowering of interaction distance, as doping perturbs the electronic structure by breaking the sp2 delocalization. In INH/Si-doped (5,5) and (9,0) SWNTs with the perpendicular orientation, the adsorption energy values are 0.243 and 0.290 eV (ESI, Table S1†), indicating that adsorption is energetically favoured onto nanotubes bearing defects, compared to pristine nanotubes.
HOMO–LUMO gap and global reactivity descriptors
The variation in HOMO–LUMO gap and quantum descriptors for INH, pristine and Si-doped (5,5) and (9,0) SWNTs, along with the conjugates, is provided in Table 1 and the ESI, Table S1.† INH has a energy gap of 3.476 eV. Pandey et al.63 reported energy gaps of 4.97 and 4.95 eV for INH calculated at the level of DFT with B3LYP/6-311G(d,p) and B3LYP/LANL2DZ methods. On the other hand, pristine (9,0) SWNT has an energy gap of 0.617 eV compared to (5,5) SWNT (0.941 eV). Low-temperature STM measurements reported an energy gap of 0.08 eV for the (9,0) SWNT,64 and for a finite C80H20 atom (5,5) SWNT, the reported band gap is 1.73 eV calculated at the level of B3LYP/6-31G*.65 An energy gap of 0.25 eV was reported for a C108H18 atom (9,0) SWNT, calculated using B3LYP/6-31G(d,p) with the GAUSSIAN03 code.66
Table 1 Optimum distance of interaction (Å), adsorption energy, Eads (eV), HOMO–LUMO energy gap (eV), and global reactivity descriptors (all units in eV) of INH, pristine and Si-doped (5,5) SWNTs, along with their functionalization with INH (cluster models). Here, I refers to PW91/DNP (DMol3) and II refers to vdW-corrected PBE/6-31G(d,p)-GD2 (G09) levels of calculation
System |
Optimum distance |
Eads |
HOMO–LUMO gap |
η |
μ |
ω |
I |
II |
I |
II |
I |
II |
INH |
— |
|
— |
|
3.476 |
3.296 |
1.738 |
−4.117 |
4.876 |
(5,5) SWNT |
— |
|
— |
|
0.941 |
0.368 |
0.470 |
−3.776 |
15.156 |
Si-Doped (5,5) SWNT |
— |
|
— |
|
0.680 |
0.372 |
0.340 |
−4.096 |
24.672 |
INH/(5,5) SWNT (parallel) |
2.98 |
3.13 |
0.089 |
0.676 |
0.856 |
0.375 |
0.428 |
−3.863 |
17.433 |
INH/(5,5) SWNT (perpendicular) |
2.84 |
3.13 |
0.088 |
0.592 |
0.859 |
0.371 |
0.429 |
−3.819 |
16.983 |
INH/Si-doped (5,5) SWNT (parallel) |
3.12 |
3.41 |
0.274 |
1.351 |
0.69 |
0.369 |
0.345 |
−4.149 |
24.948 |
INH/Si-doped (5,5) SWNT (perpendicular) |
2.72 |
1.78 |
0.243 |
1.293 |
0.645 |
0.363 |
0.322 |
−4.134 |
26.502 |
INH/Si-doped (5,5) SWNT (covalent functionalization) |
1.75 |
1.75 |
2.747 |
2.962 |
0.764 |
0.405 |
0.382 |
−3.983 |
20.765 |
(N–Si bond length) |
|
Substitutional doping of an Si atom results in a lowering of energy gap for both the nanotubes (see Tables 1 and S1†). The η value for Si-doped (5,5) SWNT is higher than Si-doped (9,0) SWNT, suggesting that the (9,0) nanotube is more reactive than (5,5) SWNT. In INH-functionalized SWNTs, the change in η is not very dramatic, as observed in Table 1. The chemical potentials (μ) of Si-doped pristine and conjugates are higher than those of the pristine SWNT and INH/SWNT, which indicates increased reactivity upon doping and subsequent adsorption of INH. On the other hand, for covalent functionalization, the μ value is lower than for noncovalent functionalization. Thus, covalent functionalization imparts stability to the conjugate, and immobilization of INH molecule over the nanotube sidewall is restricted, as opposed to noncovalent functionalization. The high ω value for (9,0) SWNT indicates that it is a better electrophile than (5,5) SWNT. The INH/Si-doped (9,0) SWNT exhibits a higher ω value compared to INH/Si-doped (5,5) SWNT, indicating that (9,0) SWNT shows a high tendency to behave as a better electron scavenger, over (5,5) SWNT.
The noncovalent functionalization of INH does not drastically modify the electronic states of the doped (9,0) SWNT, but for the (5,5) SWNT complex, it significantly affects the electronic properties, as (5,5) SWNTs are more polarizable compared to (9,0) SWNTs, and the strong electron delocalization affects the adsorption along the tube sidewall, rendering stability to the adsorbed molecule.
Covalent functionalization of INH onto Si-doped nanotubes
The optimized geometries of INH onto Si-doped (5,5) and (9,0) SWNTs is illustrated in the ESI, Fig. S1d and h.† For the INH/Si-doped (5,5) SWNT (ESI, Fig. S1d†), the aromatic ring is oriented almost perpendicularly to the nanotube basal plane, whereas for the INH/Si-doped (9,0) SWNT, the aromatic ring is adsorbed parallel to nanotube sidewall (Fig. S1h†).
The major difference between the two orientations is the alignment of INH along the (5,5) and (9,0) SWNTs. In the INH/(5,5) SWNT, the INH aromatic ring was not aligned along the nanotube periodicity, whereas for the INH/(9,0) SWNT the INH ring lies along the tube axis, which led to the aromatic ring becoming adsorbed parallel to the tube sidewall. Nevertheless, from an energetics perspective, the two systems yield comparable adsorption energy values of 2.747 and 2.782 eV, respectively and the torsional flexibility within INH would facilitate varied orientations of adsorption along the nanotube sidewall. However, inclusion of the dispersion correction to DFT, calculated at the level of DFT (discussed in the next section) suggests a similar orientation of INH along the (5,5) SWNT with a adsorption energy of 2.962 eV. This shows that, energetically, the aromatic ring of INH prefers to orient almost perpendicular to the nanotube (see Fig. S3c of ESI†) irrespective of the inclusion of a vdW correction.
The covalent attachment of INH onto pristine SWNTs has not been studied here, as it is rather difficult to determine the preferential site for bonding. The equilibrium Si–N bond distance, adsorption energies, HOMO–LUMO energy gap, and quantum molecular descriptors are detailed in Table 1 and the ESI, Table S1.† The adsorption energy of the INH/Si-doped (5,5) SWNT is lower than that of the Si-doped (9,0) CNT by 0.04 eV, which is mainly contributed from the parallel alignment of the INH aromatic ring along the nanotube sidewall, which favors better orbital overlap. The HOMO–LUMO energy gap in the INH/Si-doped (9,0) SWNT system is lower than for the INH/Si-doped (5,5) SWNT (see Table 1 and ESI, Table S1†). For covalent functionalization, there is a significant increase in the ω values for the Si-doped (9,0) SWNT and a lowering of the ω values for the Si-doped (5,5) SWNT system, suggesting that the Si-doped (9,0) SWNT system behaves as a better electrophile, compared to the Si-doped (5,5) SWNT.
Dispersion correction to the noncovalent interaction
The vdW dispersion correction to DFT for pristine and Si-doped conjugates of the INH/(5,5) SWNT is provided in Table 1. Here, a comparison is carried out for the model (5,5) SWNT system, which can also provide an insight into a similar trend expected for the INH/(9,0) SWNT complex. The inclusion of the dispersion correction results in a significant increase in the adsorption energy for both pristine and Si-doped conjugates, as depicted in Table 1. The parallel stacking of INH is energetically favoured over the perpendicular orientation with energy values of 0.676 and 1.351 eV for pristine and Si-doped nanotubes. The orientation corresponding to the amine group situated away from the SWNT was also considered, and the optimized minimum energy conformations are provided in the ESI, Fig. S2b.† The site with the amine away from the SWNT sidewall yields a lower adsorption energy of 0.62 eV and an interaction distance of 3.16 Å. Interestingly, significant structural distortion within INH is observed, suggesting a prominent influence of vdW dispersion on the overall stability of the conjugate.
However, for covalent functionalization, the dispersion correction to the adsorption is not very drastic, with a difference in adsorption energy of 0.215 eV, with no change in the interaction distance. This suggests that the vdW dispersion correction is crucial for explaining the weak noncovalent adsorption of INH. The inclusion of a dispersion correction can provide better correlation towards the energetics of interaction for weakly bound conjugates through noncovalent over covalent functionalization.
Noncovalent functionalization of INH onto pristine and Si-doped nanotube
Structure and energetics for periodic models. The optimized geometries of pristine and Si-doped (5,5) and (9,0) SWNTs is depicted in the ESI Fig. S4.† The adsorption energy, interaction distance, and variation in Fermi energy are provided in Table 2. The parallel orientation of adsorption was considered along the hollow site, as shown in Fig. 3. For Si-doped SWNTs, INH prefers the hollow site at a comparatively lower interaction distance (Table 2).
Table 2 Optimum distance of interaction (Å), adsorption energy (eV), and Fermi energy (eV), of INH, and pristine and Si-doped (5,5) and (9,0) SWNTs, along with their functionalization with INH (periodic system)
System |
Optimum distance (Å) |
Eads (eV) |
Fermi energy (eV) |
INH |
— |
— |
−0.154 |
(5,5) SWNT |
— |
— |
−0.167 |
Si-Doped (5,5) SWNT |
— |
— |
−0.165 |
INH/(5,5) SWNT (parallel) |
2.78 |
0.22 |
−0.169 |
INH/Si–doped (5,5) SWNT (parallel) |
2.60 |
0.51 |
−0.178 |
(9,0) SWNT |
— |
— |
−0.170 |
Si-Doped (9,0) SWNT |
— |
— |
−0.175 |
INH/(9,0) SWNT (parallel) |
2.73 |
0.2 |
−0.172 |
INH/Si-doped (9,0) SWNT (parallel) |
2.12 |
0.55 |
−0.180 |
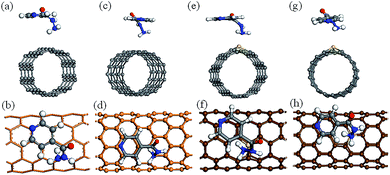 |
| Fig. 3 The periodic models of (a) INH/(5,5) SWNT, depicting the front view, (b) side view, (c) INH/(9,0) SWNT, (d) side view, (e) INH/Si-doped (5,5) SWNT, (f) side view with Si atom shown in yellow, (g) INH/Si-doped (9,0) SWNT, (h) side view. For clarity, the SWNT is shown in orange and brown. | |
The trends in adsorption energies are quite similar to those of cluster models, with the (9,0) SWNT exhibiting a higher adsorption energy than the (5,5) SWNT and Si doping enhancing the adsorption energy within the conjugates, as shown in Table 2.
The DOS and PDOS for perfect and Si-doped nanotube systems is illustrated in Fig. 4 and the ESI, Fig. S5.† The pristine (5,5) SWNT is metallic, with an energy gap of ∼1.7 eV between the Fermi level and conduction band at the first van Hove singularity. Adsorption of INH leads to the enhancement of electronic states, within the valence and conduction band regions. The PDOS for the perfect and INH-adsorbed (5,5) SWNTs shows the major contribution to the electronic states from the p-orbital, with s and d-orbitals signifying low-lying states with no contribution. However, weakly adsorbed INH does not induce any significant changes in the band gap (Fig. 4a and b). Si doping in the (5,5) SWNT results in the reduction of the band gap to ∼1.5 eV, between the Fermi level and the conduction band (Fig. 4c).
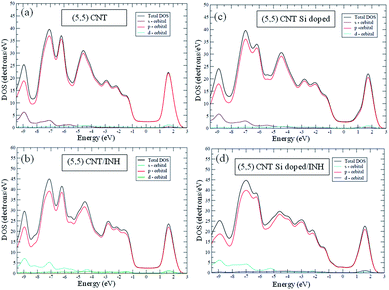 |
| Fig. 4 The DOS and PDOS corresponding to s, p, and d orbitals of (a) (5,5) SWNT, (b) INH/(5,5) SWNT, (c) Si-doped (5,5) SWNT, and (d) INH/Si-doped (5,5) SWNT. | |
Significant perturbation in the electronic states within the valence band region, along with the enhancement of electronic states around −7.5 to −3.0 eV is observed for the Si-doped (5,5) SWNT system, with the shifting of the conduction band to ∼1.5 eV (Fig. 4c). The PDOS shows a major contribution to the total DOS from the p-orbital, with the s and d-orbitals being low-lying states, with no contribution in the Fermi region.
The (9,0) SWNT (ESI, Fig. S5a†) is metallic, with a finite DOS at the Fermi region, exhibiting an energy gap of ∼1.0 eV between the Fermi level and conduction band. Unlike the (5,5) SWNT, where the conduction band has only one peak, in the (9,0) SWNT, the conduction band has two shoulder peaks. Adsorption of INH on the (9,0) SWNT results in a significant enhancement in the electronic states, both at the valence and conduction regions, with modulation of the valence bands around −7.0 to −1.5 eV. Si-Doping of the (9,0) SWNT results in a reduction in its band gap (ESI, Fig. S5c†), with perturbation in the electronic states corresponding to the conduction band region around 1–2 eV. Adsorption of INH onto the Si-doped (9,0) SWNT leads to enhancement of the electronic states, corresponding to the valence and conduction band regions (ESI, Fig. S5d†) and the PDOS shows a contribution from the p-orbital, with s and d-orbitals signifying low-lying states with no contribution in the Fermi region.
Frontier orbital analysis. The HOMO and LUMO isosurfaces of INH, and pristine and Si-doped (5,5) and (9,0) SWNTs are given in the ESI, Fig. S6 and S7.† The modulation of energy states upon functionalization is prominent in the pristine (9,0) SWNT, compared to the (5,5) SWNT. For the INH/Si-doped (5,5) SWNT, the HOMO, although delocalized along the tube sidewall, has a major contribution to the Si atom (Fig. S7a†). For perpendicular adsorption of INH, the HOMO (Fig. S7c†) has a contribution to the Si atom, whereas the LUMO has some contribution from the amine group (Fig. S7d†). Parallel adsorption of INH onto the (9,0) SWNT demonstrates the HOMO to be delocalized along the nanotube sidewall, with charge localization on the Si atom (Fig. S7e†), whereas the LUMO has no contribution on the Si atom and is localized along one of the edges (Fig. S7f†). The perpendicular adsorption of the INH/Si-doped (9,0) SWNT demonstrates a similar trend in the HOMO (Fig. S7g†), whereas the LUMO remains localized along the nanotube edges, with no contribution on the sidewall (Fig. S7h†). The frontier orbital contribution in the INH/Si-doped (5,5) and (9,0) SWNTs exemplifies significant delocalization and charge transfer mediated through the Si dopant atom. Covalent functionalization leads to significant perturbation in the electronic states, thereby modulating the frontier orbitals around the site of functionalization (Fig. S8 of ESI†).
Mulliken charge analysis. In the Si-doped (5,5) SWNT system, the charge on the Si atom is 0.85e. After parallel adsorption of INH onto the Si-doped (5,5) SWNT, the charge on the Si atom increases to 0.89e, and for perpendicular adsorption of INH, the charge on the Si-atom increases to 0.86e. The parallel adsorption of INH results in charge transfer from INH to the Si-atom of the nanotube by 0.04e (parallel orientation) and 0.01e (perpendicular orientation). Interestingly, for covalent adsorption of INH, an enhancement of 1.39e is observed on the Si atom. Covalent functionalization leads to enhanced charge transfer from INH to the Si atom in the doped (5,5) SWNT by 0.54e.In the Si-doped (9,0) SWNT/INH system, the charge on the Si atom for the (9,0) SWNT is 0.87e, which is high compared to the Si-doped (5,5) SWNT. Upon parallel adsorption of INH onto the nanotube sidewall, the charge on the Si atom increases to 0.97e, which suggests charge transfer from INH to the nanotube, mediated by the Si dopant atom. For perpendicular adsorption of INH, depletion of charge (0.80e) is observed on the Si atom. Thus parallel adsorption of INH onto the Si-doped (9,0) SWNT leads to enhanced interaction and charge transfer. Covalent functionalization results in significant charge transfer from INH to the Si atom of the nanotube (charge on Si = 1.38e). In both the Si-doped (5,5) and (9,0) SWNT/INH complexes, covalent functionalization envisages enhanced charge transfer from INH to the Si-doped nanotube.
Molecular docking of INH onto wild and mutant types of KatG
Crystal structure of KatG. The crystal structure of M. tuberculosis catalase-peroxidase (mtCP) KatG enzyme comprises two polypeptide chains, each having a length of 743 residues.67 This contains a heme protoporphyrin IX moiety, which is embedded within the active site. Surrounding the heme are the active site residues, Arg104 and His108 in the distal pocket, and His270, Trp321, and Asp381 in the proximal pocket, as depicted in Fig. 5.
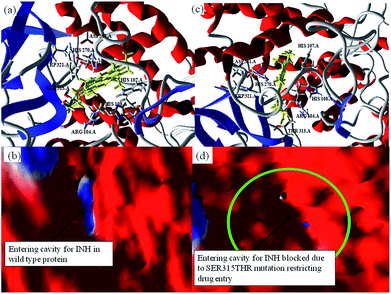 |
| Fig. 5 (a) Secondary structure of wild-type mtCP (1SJ2) protein, depicting the porphyrin ring and the active amino acid residues, (b) hydrophobic surface on wild-type protein, (c) secondary structure of mutant-type (2CCD) protein, (d) hydrophobic surface of mutant-type protein with the entering cavity blocked due to Ser315Thr mutation. | |
Resistance to INH is a continuing problem in the development of new and effective therapeutic regimes, and this is caused by deletions or point mutations in KatG, which encodes mtCP. Ser315 has been reported to be mutated to a number of amino acid residues, which results in up to a 200 fold increase in the minimum inhibitory concentration for the drug.68 Ser315 to Threonine (S315T) is one of the most common mutations, wherein Ser315 forms hydrogen bonds to one of the heme propionate groups and its modification to threonine modifies the heme pocket, thereby altering the binding site for the hydrazinyl moiety of INH and affecting charge transfer to the heme group. This mutation reduces the affinity for drug binding by increasing the steric effect and reducing the access of INH to the binding site by blocking the entering pathway, as observed in Fig. 7b and d.
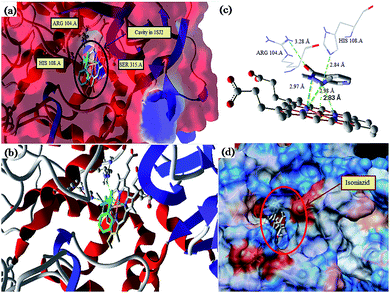 |
| Fig. 6 (a) The secondary structure of INH docked onto wild-type mtCP (1SJ2) protein, (b) closer view of the probable interactions of INH (shown in green) with Ser315, His108, and Arg104 residues, (c) the H-bonded interactions of INH with porphyrin ring, Arg104, and His108 residues, (d) the docked surface of INH within wild-type mtCP protein. | |
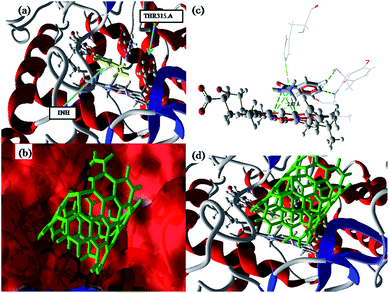 |
| Fig. 7 (a) The secondary structure of INH docked onto mutant-type mtCP (2CCD) protein in INH/Si doped (5,5) SWNT. The SWNT has not been shown for clarity, (b) hydrophobic surface with the nanotube docked at the entering cavity, (c) the H-bonded distance between INH and the porphyrin ring, along with His108, Val230, and Tyr229 residues, (d) docked conformation of SWNT and INH along the protein. The INH drug is shown in yellow and the (5,5) SWNT is shown in green. | |
Docking of INH drug. The docked conformations of INH onto wild-type mtCP are depicted in Fig. 6a and b (INH drug shown in green) and the MolDock and re-rank scores are summarized in Table S2.† The hydrogen bonding distance between INH and the protoporphyrin ring with wild-type mtCP protein (see Fig. 6c) is calculated as 2.83 Å. The INH forms intermolecular hydrogen bonds with two of the active amino acids, Arg104 and His108, at 3.28 Å and 2.84 Å, respectively (Fig. 6c). The hydrophobic surface for INH binding onto 1SJ2 shows the drug molecule in close proximity to the porphyrin ring within the binding cavity (Fig. 6d) and illustrates the interaction of INH with the active residues and porphyrin ring of mtCP.The ESI, Fig. S10a and b† illustrate the INH binding onto the active site of mutant-type mtCP (S315T). The H-bond distance between the protoporphyrin ring and INH is 3.06 Å, which has been significantly increased in comparison to the INH/wild-type mtCP (Fig. 6c). INH also forms hydrogen bonds with His108 and Tyr229 at 2.68 and 3.10 Å, respectively (Fig. S10c†). The binding cavity of mutant mtCP is blocked by the threonine, which hinders the transfusion of INH, as depicted on the hydrophobic surface (Fig. S10d†). This shows that mutation blocks the entering pathway due to steric hindrance, and INH cannot bind directly to the porphyrin ring.
Docking of INH/SWNTs. Table S2 of the ESI† provides the best docked pose in the INH/(5,5) SWNT onto wild and mutant mtCP. For the INH/(5,5) SWNT docked onto wild-type mtCP (1SJ2) (Fig. S11a†), INH is grooved within the binding cavity and the nanotube remains further away from the porphyrin ring and active residues (Fig. S11b of ESI†). Unlike the bare INH docking, in the presence of the SWNT, the amine group lies in close proximity to the porphyrin ring, with the aromatic ring directed away, as observed in Fig. S11c.† In the presence of the SWNT, considering that the amine group is in relatively close proximity to the porphyrin ring, this will facilitate less steric hindrance and the possibility of maximum interaction through H-bonding with the adjoining oxygen atoms along the porphyrin ring and the neighboring amino acids of the protein. The H-bond distance between INH and protoporphyrin ring is 2.52 Å, which is comparatively lower than INH. Likewise, a hydrogen bond is formed between INH and His108 at 3.18 Å.For the INH/(5,5) SWNT docked with mutant-type mtCP (2CCD) (Fig. S12a of ESI†), the H-bond distance between the amine and the protoporphyrin ring is 2.39 Å, which is significantly lower compared to INH in the mutant-type protein. Thus in the mutant protein, the SWNT enhances the interaction between INH and the porphyrin ring, thereby aiding INH administration, along with opening the pathway for the INH drug.
Docking of INH/Si-doped (5,5) SWNT. For the INH/Si-doped SWCNT docked onto mutant-type mtCP (2CCD) (Fig. 7a), the H-bond distance between INH and the protoporphyrin ring is 2.87 Å. The H-bond distance of INH with the amino acids His108, Val230, and Tyr229 are calculated as 2.82, 3.07, and 3.15 Å, respectively (Fig. 7c). The Si-doped (5,5) SWNT is docked within the mutant protein, enhancing the interaction between the drug and binding residues of the protein.Thus, compared to the docking of INH, the presence of the nanotube facilitates the interaction of INH with the protein, as observed from the increased H-bonded interactions, and doped nanotubes are better docked onto the protein, compared to perfect nanotubes.
Conclusion
We performed density functional calculations on the role of Si dopant atom on the structure and energetics of (5,5) and (9,0) SWNTs towards the adsorption of the antitubercular drug, INH. Si doping leads to localized deformation of the nanotube, with the dopant atom protruding out of the molecular plane. Noncovalent functionalization does not drastically perturb the electronic properties of the nanotube as observed from DOS, global reactivity parameters, and frontier orbital distribution, prior to and after functionalization. Covalent functionalization results in significant perturbation of the electronic properties of the nanotubes with enhanced adsorption energy and charge transfer. Compared to perpendicular adsorption of INH onto the nanotubes, parallel orientation is preferred, due to enhanced π-stacking interactions. Global reactivity descriptors suggest a significant increase in the reactivity and electrophilicity of the nanotubes upon Si doping, towards the interaction with the INH. Mulliken charge analysis shows charge transfer from INH to the Si-doped nanotube, which is justified from the increase in the electrophilicity of the two nanotubes with Si doping. The vdW dispersion correction is crucial for explaining weak noncovalent adsorption of INH, and can provide better correlation towards the energetics of interaction for weakly bound conjugates through noncovalent over covalent functionalization.
Finally, docking studies onto wild and mutant-type mtCP KatG proteins helps to envisage the role of the nanotube in enhancing the interaction of INH with the porphyrin ring, compared to when the drug is administered in its bare form. The presence of the nanotube support facilitates the interaction and, in the case of the mutant forms, significant interactions are observed. Si-Doped nanotubes undergo better interaction with the protein compared to pristine nanotubes. Our preliminary finding, supported by future experimental studies in this direction, can add tremendous impetus to this direction of research.
Acknowledgements
The authors acknowledge the Department of Science and Technology (DST), New Delhi, India for funding the project and grant-in-aid. NS acknowledges CSIR, New Delhi, India for the SRF fellowship and SR acknowledges DST for the INSPIRE fellowship.
Notes and references
- W. A. de Heer, A. Chaelain and D. Ugarte, A carbon nanotube field-emission electron source, Science, 1995, 270, 1179–1180 CAS.
- M. Terrones, P. M. Ajayan, F. Banhart, X. Blase, D. L. Carroll, J. C. Charlier, R. Czerw, B. Foley, N. Grobert, R. Kamalakaran, P. Kohler-Redlich, M. Ruhle, T. Seeger and H. Terrones, N-Doping and coalescence of carbon nanotubes: synthesis and electronic properties, Appl. Phys. A: Mater. Sci. Process., 2002, 74, 355–361 CrossRef CAS.
- J. H. Cho, S. J. Yang, K. Lee and C. R. Park, Si-Doping effect on the enhanced hydrogen storage of single walled carbon nanotubes and graphene, Int. J. Hydrogen Energy, 2011, 36, 12286–12295 CrossRef CAS.
- P. Ayala, R. Arenal, M. Rümmeli, A. Rubio and T. Pichler, The doping of carbon nanotubes with nitrogen and their potential applications, Carbon, 2010, 48, 575–586 CrossRef CAS.
- E. Katz and I. Willner, Biomolecule-functionalized carbon nanotubes: applications in nanobioelectronics, ChemPhysChem, 2004, 5, 1084–1104 CrossRef CAS PubMed.
- M. Zheng, A. Jagota, M. S. Strano, A. P. Santos, P. Barone, S. G. Chou, B. A. Diner, M. S. Dresselhaus, R. S. Mclean, G. B. Onoa, G. G. Samsonidze, E. D. Semke, M. Usrey and D. J. Walls, Structure-based carbon nanotube sorting by sequence-dependent DNA assembly, Science, 2003, 302, 1545–1548 CrossRef CAS PubMed.
- K. A. Williams, P. T. M. Veenhuizen, B. G. Torre, R. Eritja and C. Dekker, Nanotechnology: carbon nanotubes with DNA recognition, Nature, 2002, 420, 761 CrossRef CAS PubMed.
- G. Lu, P. Maragakis and E. Kaxiras, Carbon nanotube interaction with DNA, Nano Lett., 2005, 5, 897–900 CrossRef CAS PubMed.
- D. Tasis, N. Tagmatarchis, V. Georgakilas and M. Prato, Soluble Carbon Nanotubes, Chem.–Eur. J., 2003, 9, 4000–4008 CrossRef CAS PubMed.
- F. Tournus, S. Latil, M. I. Heggie and J.-C. Charlier, π-Stacking interaction between carbon nanotubes and organic molecules, Phys. Rev. B: Condens. Matter Mater. Phys., 2005, 72, 075431 CrossRef.
- V. M. de Menezes, S. B. Fagan, I. Zanella and R. Mota, Carbon nanotubes interacting with vitamins: first principles calculations, Microelectron. J., 2009, 40, 877–879 CrossRef CAS.
- D. Baskaran, J. W. Mays and M. S. Bratche, Noncovalent and Nonspecific Molecular Interactions of Polymers with Multiwalled Carbon Nanotubes, Chem. Mater., 2005, 17, 3389–3397 CrossRef CAS.
- G. M. A. Rahman, D. M. Guldi, S. Campidelli and M. Prato, Electronically interacting single wall carbon nanotube–porphyrin nanohybrids, J. Mater. Chem., 2006, 16, 62–65 RSC.
- E. L. Sceats and J. C. Green, Noncovalent interactions between organometallic metallocene complexes and single-walled carbon nanotubes, J. Chem. Phys., 2006, 125, 154704 CrossRef PubMed.
- L.-J. Li, A. N. Khlobystov, J. G. Wiltshire, G. A. D. Briggs and R. J. Nicholas, Diameter-selective encapsulation of metallocenes in single-walled carbon nanotubes, Nat. Mater., 2005, 4, 481–485 CrossRef CAS PubMed.
- J. Zhao, H. Park, J. Han and J. P. Lu, Electronic properties of carbon nanotubes with covalent sidewall functionalization, J. Phys. Chem. B, 2004, 108, 4227–4230 CrossRef CAS.
- F. Li, Y. Xia, M. Zhao, X. Liu, B. Huang, Z. Tan and Y. Ji, Selectable functionalization of single-walled carbon nanotubes resulting from CHn (n = 1–3) adsorption, Phys.
Rev. B: Condens. Matter Mater. Phys., 2004, 69, 165415 CrossRef.
- S.-S. Yu and W.-T. Zheng, Effect of N/B doping on the electronic and field emission properties for carbon nanotubes, carbon nanocones, and graphene nanoribbons, Nanoscale, 2010, 2, 1069–1082 RSC.
- Z. Zhou, X. Gao, J. Yan and D. Song, Doping effects of B and N on hydrogen adsorption in single-walled carbon nanotubes through density functional calculations, Carbon, 2006, 44, 939–947 CrossRef CAS.
- N. Saikia and R. C. Deka, First principles study on the boron–nitrogen domains segregated within (5,5) and (8,0) single-wall carbon nanotubes: formation energy, electronic structure and reactivity, Comput. Theor. Chem., 2012, 996, 11–20 CrossRef CAS.
- S. Roosta, S. J. Nikkhah, M. Sabzali and S. M. Hashemianzadeh, Molecular dynamics simulation study of boron-nitride nanotubes as a drug carrier: from encapsulation to releasing, RSC Adv., 2016, 6, 9344–9351 RSC.
- Y.-T. Li and T.-C. Chen, Effect of B/N co-doping on the stability and electronic structure of single-walled carbon nanotubes by first-principles theory, Nanotechnology, 2009, 20, 375705 CrossRef PubMed.
- Z. Junzhe and W. Chongyu, First-principles study of the effects of Si doping on geometric and electronic structure of closed carbon nanotube, Chin. Sci. Bull., 2005, 50, 1823–1828 CrossRef.
- J. L. Fye and M. F. Jarrold, Structures of Silicon-Doped Carbon Clusters, J. Phys. Chem. A, 1997, 101, 1836–1840 CrossRef CAS.
- C. Ray, M. Pellarin, J. L. Lerme, J. L. Vialle, M. Broyer, X. Blase, P. Mélinon, P. Kéghélian and A. Perez, Synthesis and Structure of Silicon-doped Heterofullerenes, Phys. Rev. Lett., 1998, 80, 5365–5368 CrossRef CAS.
- C. Song, Y. Xia, M. Zhao, X. Liu, F. Li, B. Huang, H. Zhang and B. Zhang, Functionalization of silicon-doped single walled carbon nanotubes at the doping site: an ab initio study, Phys. Lett. A, 2006, 358, 166–170 CrossRef CAS.
- R. Wang, D. Zhang, Y. Liu and C. Liu, A theoretical study of silicon-doped boron nitride nanotubes serving as a potential chemical sensor for hydrogen cyanide, Nanotechnology, 2009, 20, 505704 CrossRef PubMed.
- H. Jiang, D. Zhang and R. Wang, Silicon-doped carbon nanotubes: a potential resource for the detection of chlorophenols/chlorophenoxy radicals, Nanotechnology, 2009, 20, 145501 CrossRef PubMed.
- G. Guo, F. Wang, H. Sun and D. Zhang, Reactivity of silicon-doped carbon nanotubes toward small gaseous molecules in the atmosphere, Int. J. Quantum Chem., 2008, 108, 203–209 CrossRef CAS.
- A. Mavrandonakis, G. E. Froudakis, M. Schnell and M. Muhlhäuser, From Pure Carbon to Silicon–Carbon Nanotubes: An Ab initio Study, Nano Lett., 2003, 3, 1481–1484 CrossRef CAS.
- J. Li, S. Q. Yap, C. F. Chin, Q. Tian, S. L. Yoong, G. Pastorin and W. H. Ang, Platinum(IV) prodrugs entrapped within multiwalled carbon nanotubes: selective release by chemical reduction and hydrophobicity reversal, Chem. Sci., 2012, 3, 2083–2087 RSC.
- M. Adeli, F. Hakimpoor, M. Ashiri, R. Kabiri and M. Bavadi, Anticancer drug delivery systems based on noncovalent interactions between carbon nanotubes and linear-dendritic copolymers, Soft Matter, 2011, 7, 4062–4070 RSC.
- A. A. Bhirde, S. Patel, S. Patel, A. A. Sousa, V. Patel, A. A. Molinolo, Y. Ji, R. D. Leapman, J. S. Gutkind and J. F. Rusling, Distribution and clearance of PEG-single-walled carbon nanotube cancer drug delivery vehicles in mice, Nanomedicine, 2010, 5, 1535–1546 CrossRef CAS PubMed.
- W. Zhang, Z. Zhang and Y. Zhang, The application of carbon nanotubes in target drug delivery systems for cancer therapies, Nanoscale Res. Lett., 2011, 6, 555 CrossRef PubMed.
- N. Saikia and R. C. Deka, Density functional study on the adsorption of the drug isoniazid onto pristine and B-doped single wall carbon nanotubes, J. Mol. Model., 2013, 19, 215–226 CrossRef CAS PubMed.
- N. Saikia, S. K. Pati and R. C. Deka, First principles calculation on the structure and electronic properties of BNNTs functionalized with isoniazid drug molecule, Appl. Nanosci., 2012, 2, 389–400 CrossRef CAS.
- N. Saikia and R. C. Deka, A Comparison of the Effect of Nanotube Chirality and Electronic Properties on the π–π Interaction of Single-Wall Carbon Nanotubes with Pyrazinamide Antitubercular Drug, Int. J. Quantum Chem., 2012, 113, 1272–1284 CrossRef.
- N. Saikia and R. C. Deka, Density functional calculations on adsorption of 2-methylheptylisonicotinate antitubercular drug onto functionalized carbon nanotube, Comput. Theor. Chem., 2011, 964, 257–261 CrossRef CAS.
- Tuberculosis and the tubercle bacillus, ed. S. T. Cole, K. D. Eisenach, D. N. McMurray and W. R. Jacobs Jr, ASM Press, Washington, 2005 Search PubMed.
- World Health Organization, Global Tuberculosis Control, 2011, ISBN 978 92 4 156438 0 Search PubMed.
- E. Vavríková, S. Polanc, M. K. Cevar, J. K. Smrlj, K. Horváti, S. Bosze, J. S. ríková, A. s. Imramovský and J. V. sová, New series of isoniazid hydrazones linked with electron-withdrawing substituents, Eur. J. Med. Chem., 2011, 46, 5902–5909 CrossRef PubMed.
- D. Maher, P. Chaulet, S. Spinaci and A. Harries, Treatment of tuberculosis: Guidelines for national programmes, World Health Organization, Geneva, 1997 Search PubMed.
- K. Johnsson and P. G. Schultz, Mechanistic studies of the oxidation of isoniazid by the catalase-peroxidase from Mycobacterium tuberculosis, J. Am. Chem. Soc., 1994, 116, 7425–7426 CrossRef CAS.
- Y. Zhang, B. Heym, B. Allen, D. Young and S. Cole, The catalase peroxidase gene and isoniazid resistance of Mycobacterium tuberculosis, Nature, 1992, 358, 591–593 CrossRef CAS PubMed.
- R. S. Magliozzo and J. A. Marcinkeviciene, The role of Mn(II)-peroxidase activity of mycobacterial catalase-peroxidase in activation of the antibiotic isoniazid, J. Biol. Chem., 1997, 272, 8867–8870 CrossRef CAS PubMed.
- I. Zanella, S. B. Fagan, R. Mota and A. Fazzio, Ab initio study of pristine and Si-doped capped carbon nanotubes interacting with nimesulide molecules, Chem. Phys. Lett., 2007, 439, 348–353 CrossRef CAS.
- H. J. Monkhorst and J. D. Pack, On Special Points for Brillouin Zone Integrations, Phys. Rev. B: Solid State, 1976, 13, 5188–5192 CrossRef.
- J. P. Perdew and Y. Wang, Accurate and simple analytic representation of the electron–gas correlation energy, Phys. Rev. B: Condens. Matter Mater. Phys., 1992, 45, 13244–13249 CrossRef.
- S. Kristyan and P. Pulay, Can (Semi) Local Density Functional Theory Account for the London Dispersion Forces?, Chem. Phys. Lett., 1994, 229, 175–180 CrossRef CAS.
- L. Goerigkab and S. Grimme, A thorough benchmark of density functional methods for general main group thermochemistry, kinetics, and noncovalent interactions, Phys. Chem. Chem. Phys., 2011, 13, 6670–6688 RSC.
- S. Grimme, Semiempirical GGA-type density functional constructed with a long-range dispersion correction, J. Comput. Chem., 2006, 27, 1787–1799 CrossRef CAS PubMed.
- S. Grimme, J. Antony, S. Ehrlich and H. Krieg, A consistent and accurate ab initio parametrization of density functional dispersion correction (DFT-D) for the 94 elements H-Pu, J. Chem. Phys., 2010, 132, 154104 CrossRef PubMed.
- M. J. Frisch, G. W. Trucks, H. B. Schlegel, G. E. Scuseria, A. M. Robb, J. R. Cheeseman, G. Scalmani, V. Barone, B. Mennucci and G. A. Petersson, et al., Gaussian 09, Gaussian, Inc., Wallingford, CT, 2009 Search PubMed.
- R. S. Mulliken, Electronic Structures of Molecules. XI. Electroaffinity, Molecular Orbitals and Dipole Moments, J. Chem. Phys., 1934, 2, 782–793 CrossRef CAS.
- R. G. Parr, L. von Szentpály and S. Liu, Electrophilicity Index, J. Am. Chem. Soc., 1999, 121, 1922–1924 CrossRef CAS.
- R. Storn and K. Price, Differential Evolution – A Simple and Efficient Adaptive Scheme for Global Optimization over Continuous Spaces, Technical Report, International Computer Science Institute, Berkley, CA, 1995 Search PubMed.
- J.-M. Yang and C.-C. Chen, GEMDOCK: a generic evolutionary method for molecular docking, Proteins, 2004, 55, 288–304 CrossRef CAS PubMed.
- G. M. Morris, D. S. Goodsell, R. S. Halliday, R. Huey, W. E. Hart, R. K. Belew and A. J. J. Olson, Comput. Chem., 1998, 19, 1639–1662 CrossRef CAS.
- L. H. Jensen, The Crystal Structure of Isonicotinic Acid Hydrazide, J. Am. Chem. Soc., 1954, 76, 4663–4672 CrossRef CAS.
- R. Saito, G. Dresselhaus and M. S. Dresselhaus, Physical Properties of Carbon Nanotubes, Imperial College Press, London, UK, 2001, p. 272 Search PubMed.
- N. Yuan, H. Bai, Y. Ma and Y. Ji, First-principle simulations on silicon-doped armchair single-walled carbon nanotubes of various diameters, Physica E, 2014, 64, 195–203 CrossRef CAS.
- R. Bian, J. Zhao and H. Fu, Silicon-doping in carbon nanotubes: formation energies, electronic structures, and chemical reactivity, J. Mol. Model., 2013, 19, 1667–1675 CrossRef CAS PubMed.
- A. K. Pandey, A. Bajpai, V. Baboo and A. Dwivedi, Structural, Electronic, and Vibrational Properties of Isoniazid and Its Derivative N-Cyclopentylidenepyridine-4-carbohydrazide: A Quantum Chemical Study, J. Theor. Chem., 2014, 894175, 1–15 Search PubMed.
- M. Ouyang, J.-L. Huang, C. L. Cheung and C. M. Lieber, Energy Gaps in “Metallic” Single-Walled Carbon Nanotubes, Science, 2001, 292, 702–705 CrossRef CAS PubMed.
- Z. Zhou, M. Steigerwald, M. Hybertsen, L. Brus and R. A. Friesner, Electronic Structure of Tubular Aromatic Molecules Derived from the Metallic (5,5) Armchair Single Wall Carbon Nanotube, J. Am. Chem. Soc., 2004, 126, 3597–3607 CrossRef CAS PubMed.
- A. A. EL-Barbary, K. M. Eid, M. A. Kamel, H. M. Osman and G. H. Ismail, Effect of Tubular Chiralities and Diameters of Single Carbon Nanotubes on Gas Sensing Behavior: A DFT Analysis, J. Surf. Eng. Mater. Adv. Technol., 2014, 4, 66–74 Search PubMed.
- T. Bertrand, N. A. J. Eady, J. N. Jones, J. M. Jesmin Nagy, B. Jamart-Gregoire, E. L. Raven and K. A. Brown, Crystal structure of Mycobacterium tuberculosis catalase-peroxidase, J. Biol. Chem., 2004, 279, 38991–38999 CrossRef CAS PubMed.
- D. van Soolingen, P. E. de Haas, H. R. van Doorn, E. Kuijper, H. Rinder and M. W. Borgdorff, Mutations at amino acid position 315 of the KatG gene are associated with high-level resistance to isoniazid, other drug resistance, and successful transmission of Mycobacterium tuberculosis in the Netherlands, J. Infect. Dis., 2000, 182, 1788–1790 CrossRef CAS PubMed.
Footnote |
† Electronic supplementary information (ESI) available: Noncovalent adsorption of INH onto (5,5) and (9,0) SWNTs, DOS and PDOS plots, frontier orbitals, covalent functionalization of INH onto Si-doped (5,5) and (9,0) SWNTs, INH docked onto mutant-type mtCP (2CCD) protein. See DOI: 10.1039/c6ra16020d |
|
This journal is © The Royal Society of Chemistry 2016 |