DOI:
10.1039/C6RA20397C
(Paper)
RSC Adv., 2016,
6, 109786-109792
Polarizable polysiloxane stationary phase containing a cyano unit attached to an aromatic side group for highly selective separation of H-bonding and aromatic analytes
Received
12th August 2016
, Accepted 11th November 2016
First published on 11th November 2016
Abstract
A new polarizable polysiloxane stationary phase containing a cyano unit attached to an aromatic side group, called CPPP [14.6% 3,4-bis(4-cyanophenyl)-2,5-diphenyl phenyl polysiloxane], was synthesized and used as a stationary phase for capillary gas chromatography (GC). The chemical components of CPPP were characterized by 1H nuclear magnetic resonance spectroscopy. The stationary phase exhibited excellent film-forming ability and high column efficiency (3852 plates per m) for naphthalene at 120 °C. The thermal stability of CPPP was evaluated by thermogravimetric analysis and column bleeding. Results revealed that CPPP could endure high temperatures up to 370 °C. Moreover, the selectivity of the CPPP stationary phase for H-bonding and aromatic analytes, including Grob test mixtures, fatty alcohols and substituted benzenes, was evaluated. Relying on sensitive dipole–induced dipole, H-bonding and π–π interactions with specific solutes, CPPP showed advantages over current stationary phases for separations of polycyclic aromatic hydrocarbons and aromatic aldehydes. In conclusion, the new CPPP stationary phase with high thermal stability and unique selectivity exhibits great potential for GC separations.
1 Introduction
Numerous polysiloxanes containing phenyl or diphenyl substituents are widely used as commercial stationary phases for capillary gas chromatography (GC) because of their high column efficiency, good thermal stability, and high polarity compared with dimethyl polysiloxanes. Among these phenyl/diphenyl substituted polysiloxanes, the content of the diphenyl group reaches 75%,1,2 resulting in increased selectivity for polar compounds. However, polysiloxanes with high phenyl/diphenyl content are crystalline solids, instead of gums or highly viscous liquids, and thus exhibit weakened coating ability during column preparation.
The polarity and selectivity of phenyl/diphenyl substituted polysiloxanes should be increased while maintaining their forms as gums or liquids. This goal can be achieved by introducing polar substituent groups, such as methoxyl and cyano group, to the phenyl ring.3 The content of phenyl/diphenyl group should also be decreased to prevent polysiloxanes from forming solid. Based on the enhanced dipole–dipole interaction with solutes, the modified stationary phase is expected to exert improved selectivity for polar compounds.
Dipole–induced dipole interaction between the stationary phase and solutes is “softer” and more sensitive to slight molecular differences in similar structures compared with dipole–dipole interaction. For instance, Lee et al.4,5 developed polysiloxanes with improved polarizability by using biphenyl, dicyanobiphenyl, and naphthyl. The crosslinked stationary phases exhibit stronger dipole–induced dipole interaction than phenyl/diphenyl substituted polysiloxanes and possess moderate thermal stabilities, improved polarity, and unique selectivity for structural isomers, such as polycyclic aromatic amines.
Increasing the number of rings of polycyclic aromatic hydrocarbons (PAHs) grafted to the backbone of polysiloxanes can enhance the average molecular polarizability. According to ref. 6, the average molecular polarizability values of benzene, biphenyl, trifluoro-substituted tetraphenyl-phenyl and cyano-substituted tetraphenyl-phenyl are 10.40, 20.05, 46.90, and 64.91 Å3, respectively. Increased polarizability can strengthen the dipole–induced dipole interaction between the grafted polysiloxanes and polar compounds, thereby improving the selectivity for isomers.7,8 Moreover, the new 3,4-bis(4-cyanophenyl)-2,5-diphenyl phenyl polysiloxane (CPPP) phase exhibits blends of polarizable as well as polar characteristics that make CPPP have excellent separation performances for analytes having conjugated π-electrons. The participant of cyanophenyl-substituted functional group can produce various of interactions (e.g., dispersive, acid–base, and dipole–induced dipole), therefore, CPPP can be applied to separate samples covering a broad polarity range.
Based on above points, a new polarizable stationary phase with 14.6% cyano-substituted tetraphenyl-phenyl functional groups was synthesized. The details of the method for synthesis of CPPP are presented in Fig. 1. Chromatographic properties, such as column efficiency, polarity, thermal stability, repeatability, and selectivity of CPPP-coated fused silica capillary columns, were systematically investigated. The fabricated capillary columns provided excellent selectivity and high separation efficiency for solutes with similar chemical structures, such as substituted benzenes, PAHs, and aromatic aldehydes.
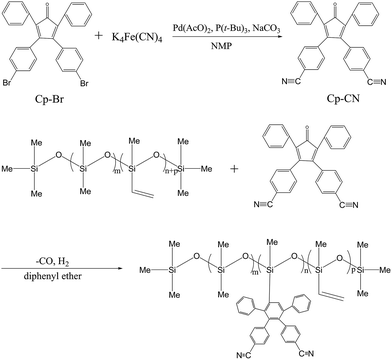 |
| Fig. 1 Reaction scheme for preparation of CPPP. | |
2 Experiment
2.1 Reagents and apparatus
Measurements were conducted on GC-2014C (Shimadzu, Tokyo, Japan) equipped with a capillary split injection system and a flame-ionization detector. Thermogravimetric analysis (TGA) was employed on an LCT-2 TGA instrument (Beijing Optical Instrument Factory, Beijing, China). 1H nuclear magnetic resonance (NMR) spectra were recorded on a DPX 300 spectrometer (Bruker Analytische Messtechnik, Bremen, Germany). High-resolution mass spectrometry (HRMS) was obtained with a magnetic sector type analyzer using EI method. Fused silica capillary tubes were produced in our laboratory from fiber-level (SiO2; 99.999% purity) raw tubes by using a self-made drawing machine. SiO2 (99.999% purity) was purchased from Beijing Shengbogaotai Optical Technology Co., Ltd. The external coating of the fused silica columns was polyamide. All GC separations in this work were performed on column I (30 m × 0.25 mm i.d.; film thickness, 0.50 μm), except the separation of polyethylene pyrolysis products, which was performed on column II (10 m × 0.25 mm i.d.; film thickness, 0.50 μm). Commercial DB-5 and DB-17 capillary columns (30 m × 0.25 mm i.d.; film thickness, 0.50 μm) were purchased from Agilent Technologies.
All reagents and other testing compounds, except aromatic aldehydes (industrial grade), are of analytical grade. Polyethylene pyrolysis products were prepared as described in ref. 9. The PAHs and aromatic aldehydes were prepared by mixing various chemically pure reagents together.
2.2 Synthesis of 3,4-bis(4-bromophenyl)-2,5-diphenylcyclopenta-2,4-dien-1-one (Cp-Br)10
3,4-Bis(4-bromophenyl)-2,5-diphenylcyclopenta-2,4-dien-1-one was synthesized through the cyclization reaction between 1,2-bis(4-bromophenyl)ethane-1,2-dione (12.1 g, 0.033 mol) and dibenzyl ketone (6.9 g, 0.033 mol) in 50.0 mL of absolute ethanol under nitrogen atmosphere. When the solution was heated at approximately 75 °C, KOH (1.0 g) in 5.0 mL of ethanol was added dropwise into the solution for more than 1 h under stirring at 78 °C. The reaction was allowed to continue for 30 min, and the mixture was filtered after cooling. The crude product was re-crystallized from ethanol/toluene (v/v = 1
:
1) to obtain pure 3,4-bis(4-bromophenyl)-2,5-diphenylcyclopenta-2,4-dien-1-one (14.3 g, 80.0%) as bright black crystals.
2.3 Synthesis of 3,4-bis(4-cyanophenyl)-2,5-diphenylcyclopenta-2,4-dien-1-one (Cp-CN)
The compound Cp-CN was synthesized according to ref. 9. Briefly, compound Cp-Br (2.00 g, 3.68 mmol), K4Fe(CN)6 (0.62 g, 1.48 mmol), Pd(OAc)2 (0.008 g, 0.04 mmol), P(t-Bu)3 (0.32 mL, 0.12 mmol) and Na2CO3 (0.16 mg, 1.48 mmol) were dissolved in 40.0 mL of N-methyl pyrrolidone under N2 atmosphere. The mixture was stirring for 8 hours at 140 °C. After removing the solvent under reduced pressure, the crude product was obtained. Then the crude product was purified by column chromatography on silica gel to obtain compound Cp-CN as dark crystals (0.77 g, 48.1%). 1H NMR (400 MHz, CDCl3, δppm): 7.09 (d, 4H); 7.12–7.20 (m, 4H); 7.34–7.42 (m, 6H); 7.62 (d, 4H). HRMS (EI) calcd for C31H18N2O [M+], 434.1419; found, 434.1428.
2.4 Procedure for preparation of CPPP
Methyl vinyl polysiloxanes with 18% vinyl group were prepared using the procedure reported by Feng et al.11 CPPP was synthesized through the Diels–Alder reaction of polymethylvinylsiloxane and Cp-CN. Sufficient amounts of Cp-CN (3.9 g, 9.0 mmol) and methyl vinyl polysiloxane (4.1 g, containing 8.8 mmol vinyl) were dissolved in 30.0 mL of diphenyl ether and heated at 220 °C for 48 h under a continuous stream of nitrogen. The color of the solution changed from dark brown to yellow with a large number of bubbles. Polymerization reaction was terminated when no bubble was observed. The solution was divided into two layers after cooling, and the upper diphenyl ether layer was removed to obtain a high viscosity polymer. For purification, the polymer was dissolved in toluene, precipitated with methanol three times, and then vacuum dried at 50 °C to obtain a clear, pale yellowish gum. The yield was about 50.4%. 1H NMR (400 MHz, CDCl3, δppm): 6.92 (m, 4H); 7.06 (t, 2H); 7.20 (dd, 4H); 7.39 (m, 4H); 7.42 (m, 4H); 7.61 (s, 1H). Peaks at 5.89 and 0.079 ppm were respectively attributed to vinyl and C–H on Si atoms in the skeleton of polysiloxane.
2.5 Column preparation
Before coating, the fused silica capillary tubes were rinsed with 5 mL of dichloromethane and purged with nitrogen at 250 °C for 3 h.12,13 The column was statically coated with 0.80% solution (w/v) of CPPP in dichloromethane [containing dicumylperoxide at about 4% (w/w) of the weight of the stationary phase] as a free radical initiator14 with 0.50 μm-thick film.15 After coating, the column was conditioned under nitrogen flow at 50 °C for 0.5 h, temperature-programmed at a rate of 1 °C min−1 to 160 °C (maintained for 3 h to facilitate cross-linking), and then to 370 °C (maintained for 24 h) at 2 °C min−1.
2.6 Column evaluation
High purity nitrogen was used as carrier gas, with an injection split ratio of 30
:
1. The efficiency of the column was evaluated by measuring the number of plates per meter at 120 °C for naphthalene. The polarity of the stationary phase was characterized using McReynolds constants; the Abraham solvation parameter model was used to quantitatively evaluate individual inter-molecular interactions between CPPP and solutes.16–18
3 Results and discussion
3.1 Characterization of the stationary phase by NMR spectroscopy
Chemical composition of 14.6% cyano-substituted tetraphenyl-phenyl group in CPPP was estimated from the signal integrals of the 1H NMR spectrum. The content of the remaining vinyl groups was 3.3%. During CPPP synthesis, 3,4-bis(4-cyanophenyl)-2,5-diphenylcyclopenta-2,4-dien-1-one with huge steric hindrance did not react completely with vinyl groups in methyl vinyl polysiloxane, leading to a small percent of vinyl retained in each polymer mixture for crosslinking.
3.2 Column efficiency
The efficiency of the column was evaluated using the number of plates per meter at 120 °C for naphthalene at a liner velocity of 8 cm s−1. The column efficiency was 3852 plates per meter and exhibited an coating efficiency of 79.7%. Thus, this new stationary phase exerts good film-forming ability and coating performance. We also synthesized other polysiloxane containing 18.0% cyano-substituted tetraphenyl-phenyl group, increasing the content of tetraphenyl-phenyl group to 18.0% in the polymer generated a solid, instead of a gummy product. This result is consistent with those previously reported by Lee et al.4 Therefore, similar to biphenyl polymers, the column cannot be used at low temperature; as such, the CPPP stationary phase with 14.6% functional groups was further studied.
3.3 McReynolds constants and Abraham system constants
The polarity and molecular interactions with solutes of CPPP are represented by McReynolds constants19 and then compared with DB-17 (50%-phenyl, 50%-methyl polysiloxanes)20 (Table 1). CPPP exhibited medium polarity. By contrast, the overall polarity of the CPPP stationary phase was lower than that of DB-17 but similar to that of 42%-diphenyl, 58%-dimethyl polysiloxanes according to the reference graph (CP-index versus diphenyl content of dimethyl diphenyl-polysiloxanes).9 Based on the structure of CPPP, the content of functional group was about 14.6% in the polymer, thereby indicating that a tetraphenyl-phenyl group acts as more than two diphenyl groups in chromatographic separation. Analysis of the specific values of McReynolds constants indicated that CPPP exhibited high Z′, U′, and S′ values. This finding suggested the strong dipole–induced dipole, and H-bond donor/acceptor interactions of CPPP with solutes. Therefore, the CPPP stationary phase is suitable for separation of polar and easily polarizable compounds.
Table 1 McReynolds constants and the CP-index of CPPP and DB-17
Column |
X′ |
Y′ |
Z′ |
U′ |
S′ |
Sum |
CP-indexa |
CP-index which was calculated from the sum of the first five Rohrschneider–McReynolds constants divided by the sum of the Rohrschneider–McReynolds constants of OV-275 multiplied by 100. |
CPPP |
111 |
171 |
157 |
226 |
169 |
834 |
19.8 |
DB-17 (ref. 20) |
119 |
158 |
162 |
243 |
174 |
856 |
20.3 |
The solvation parameter model developed by Abraham et al. was used to characterize the intermolecular interactions between CPPP and solutes.21,22 Table 2 lists the Abraham system constants of the CPPP stationary phase at 80 °C, 100 °C, and 120 °C. Data indicated that CPPP showed strong intermolecular interactions with solutes via dipole–induced dipole interaction (s), dispersive interactions (l), and H-bond basicity (a).23 This result is in good agreement with that of McReynolds constants. As such, we used this new stationary phase to separate substituted benzene isomers, PAHs, and aromatic aldehydes in following sections. The final separation results exceeded our expectations.
Table 2 Abraham system constants of the CPPP stationary phasea
T (°C) |
c |
e |
s |
a |
b |
l |
n |
R2 |
e, s, a, b, l, n, and R2 stand for π–π/n–π interactions, dipole/polarizable interactions, H-bond basicity, H-bond acidity, dispersive interactions, number of solutes used in multiple linear regression, and coefficient of determination, respectively. |
80 |
−3.046 |
−0.004 |
0.799 |
0.730 |
0 |
0.692 |
38 |
0.99 |
100 |
−3.315 |
0.021 |
0.721 |
0.642 |
0 |
0.665 |
38 |
0.99 |
120 |
−3.482 |
0.048 |
0.672 |
0.528 |
0 |
0.629 |
38 |
0.99 |
3.4 Selectivity for Grob test mixtures
The prepared stationary phase exhibited good wettability on the inner surface of the capillary tube as shown in the chromatogram of the Grob test mixtures24 (Fig. 2). The mixtures were well resolved and showed symmetrical peaks except 2,3-butanediol (peak 1). The tailing factor of 2,3-butanediol on the CPPP column was close to 1.09, indicating slight reversible adsorption. The elution order of Grob test mixtures remained mostly unaltered compared with that of SOP-50 (50%-diphenyl, 50%-dimethyl polysiloxanes).25 Irreversible acid–base adsorption was indicated by the reduced peak areas of the acid (peak 6) and amine (peak 11) on the chromatographic column. Notably, 2,6-dimethylphenol (peak 8) was eluted after 2,6-dimethyl aniline (peak 7), thereby indicating that the new column more strongly retained acidic analytes than alkaline ones, which was quite different from the polarizable stationary phases studied in our previous works.8 Moreover, the three homologous fatty acid methyl esters (methyl decanoate, methyl undecanoate and methyl dodecanoate) were baseline separated on the CPPP column, TZE1 and TZE2 were calculate to be 38.5 and 36.4 respectively, demonstrating the good separation efficiency of the CPPP column.
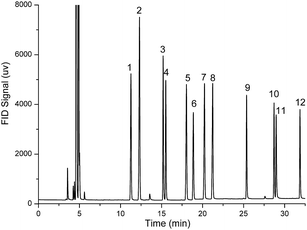 |
| Fig. 2 Chromatogram of Grob test mixtures on the CPPP column. Peaks: (1) 2,3-butanediol; (2) decane; (3) undecane; (4) 1-octanol; (5) nonanal; (6) 2-ethylhexanoic acid; (7) 2,6-dimethylaniline; (8) 2,6-dimethylphenol; (9) methyl decanoate; (10) methyl undecanoate; (11) dicyclohexylamine; and (12) methyl dodecanoate. Conditions: temperature was increased from 40 °C to 160 °C at 3 °C min−1. Carrier gas velocity: 13.5 cm s−1. The injection and detector temperatures were both 220 °C. | |
3.5 Column bleeding and operating range
The thermal stability of CPPP polymer was analyzed via TGA from 100 °C to 700 °C at 10 °C min−1 under helium. The TGA curve for CPPP was shown in Fig. 3. The onset of thermal decomposition of CPPP was about 400 °C and the polymer then significantly degraded at 410 °C. The thermal stability of the CPPP column was further evaluated by GC separation of the polyethylene pyrolysis products after the column was conditioned at 370 °C for 24 h. As shown in Fig. 4, no obvious baseline drift was observed on the CPPP column and it still exhibited excellent separation performance for the analytes until 370 °C. The good thermal stability of the CPPP phase demonstrated its potential for practical applications.
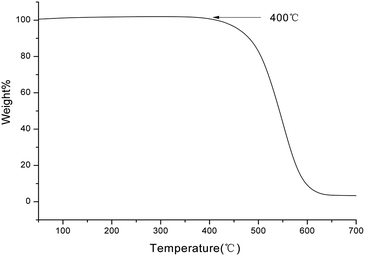 |
| Fig. 3 TGA analysis for CPPP. | |
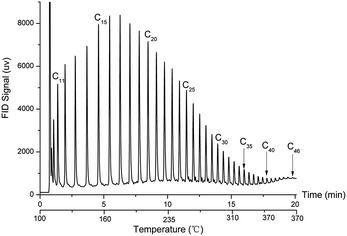 |
| Fig. 4 Chromatograms of the polyethylene pyrolysis products on the CPPP column (10 m × 0.25 mm i.d.). Conditions: temperature was increased from 100 °C (maintained for 1 min) to 370 °C (maintain for 5 min) at 15 °C min−1. Carrier gas velocity: 15 cm s−1. The injection and detector temperatures were both 400 °C. | |
3.6 Separation performance and retention behaviour
3.6.1 Separation performance of CPPP for H-bonding analytes. Based on the obtained data in Section 3.3, a mixture of alcohols and n-alkanes was separated on CPPP to investigate its separation performance for H-bonding analytes. A commercial DB-5 column was also provided for reference. As shown in Fig. 5, all components were baseline resolved with nice peak shapes on both columns. However, the retention behaviour of CPPP was differ greatly from the DB-5 stationary phase. On the DB-5 column, the components were eluted according to their increasing boiling points. While on CPPP, the alcohols were eluted after corresponding alkanes though the alcohol has a lower boiling point in each pair. This finding suggested that the H-bonding interaction between CPPP and H-bonding analytes played a significant function in separating polar compounds.
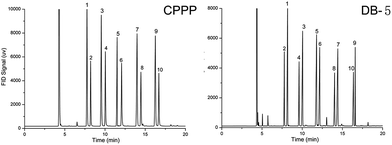 |
| Fig. 5 Chromatograms for separation of the mixture of n-alkanes and alcohols on CPPP and DB-5 columns. Peaks: (1) n-undecane; (2) 1-octanol; (3) n-dodecane; (4) 1-nonanol; (5) n-tridecane; (6) 1-decanol; (7) n-tetradecane; (8) 1-undecanol; (9) n-pentadecane; and (10) 1-dodecanol. Conditions: temperature was increased from 100 °C (maintained for 5 min) to 160 °C at 5 °C min−1. Carrier gas velocity: 12 cm s−1. The injection and detector temperatures were both 220 °C. | |
3.6.2 Separation performance of CPPP for substituted benzenes. The aromatic structure of CPPP is conducive for enhancing π–π interactions among analytes of the π-system. Consequently, CPPP was employed to separate the substituted benzenes. As shown in Fig. 6, the mixture containing 23 different di- and tri-substituted benzenes was effectively separated and sharp symmetrical peaks were obtained on CPPP. Peak pairs, such as 2-chlorotoluene (peak 1; b.p., 158.5 °C)/3-chlorotoluene (peak 2; b.p., 159.8 °C) and 1,3-dichlorobenzene (peak 8; b.p., 173.0 °C)/1,4-dichlorobenzene (peak 9; b.p., 173.4 °C) were baseline resolved on the CPPP column despite minor differences in their boiling points. The above mentioned results demonstrated the excellent selectivity of CPPP for aromatic analytes with subtle differences in their structures.
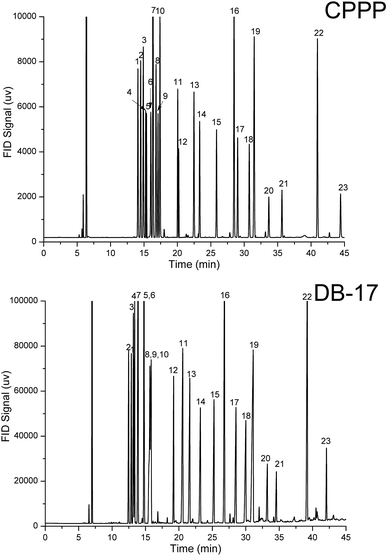 |
| Fig. 6 Chromatograms of substituted benzenes on the CPPP and DB-17 columns. Peaks: (1) 2-chlorotoluene; (2) 3-chlorotoluene; (3) 4-chlorotoluene; (4) 1,3,5-trimethylbenzene; (5) 1,3,5-trimethylbenzene; (6) tert-butylbenzene; (7) mercaptobenzene; (8) 1,3-dichlorobenzene; (9) 1,4-dichlorobenzene; (10) 1,2,3-trimethylbenzene; (11) benzyl cyanide; (12) α,α-dichlorotoluene; (13) p-nitrotoluene; (14) o-nitrochlorobenzene; (15) p-nitrobromobenzene; (16) diphenylmethane; (17) 4-nitrobenzyl chloride; (18) 2,4-dinitrotoluene; (19) 1-chloro-2,4-dinitrobenzene; (20) 1-chloro-3,4-dinitrobenzene; (21) 1-fluoro-2,4-dinitrobenzene; (22) 1-bromo-2,4-dinitrobenzene; (23) triphenylmethane. Conditions: temperature was increased from 60 °C to 320 °C at 6 °C min−1. Carrier gas velocity: 10 cm s−1. The injection and detector temperatures were both 320 °C. | |
3.6.3 Separation performance of CPPP for PAHs. For PAHs, the sensitive dipole–induced dipole interaction and concentrated π–π interaction are extremely important in distinguishing CPPP from the traditional polysiloxane stationary phases in terms of the retention behavior and resolving ability (Fig. 7). The baseline separation of all components was achieved, and good peak shapes were obtained on the CPPP column. On the DB-17 column, the peak pairs of 8-hydroxyquinoline (peak 8; b.p., 267 °C)/acenaphthylene (peak 9; b.p., 268 °C) and phenanthrene (peak 16; b.p., 340 °C)/anthracene (peak 17; b.p., 342 °C) were co-eluted. Phenanthrene and anthracene are known critical pairs in GC analysis because of their relatively similar boiling points.24 However, this pair can be successfully separated on the CPPP column. In terms of the retention behavior, phenanthrenequinone and 1,8-dihydroxyanthracene-9,10-dione exhibited a reverse elution order on the CPPP column compared with the DB-17 column. This phenomenon may be attributed to the polarizable cyano-substituted tetraphenyl-phenyl as appended functional groups to the polysiloxanes. Compared with phenyl and biphenyl, the cyano-substituted tetraphenyl-phenyl used in this study was more polarizable. The increased average molecular polarizability of cyano-substituted tetraphenyl-phenyl can enhance the inductive capability of the stationary phases for polar analytes as well as their dispersive interactions with aromatic analytes. Therefore, based on these results, CPPP can be applied in environmental, water, and petroleum analyses.26,27
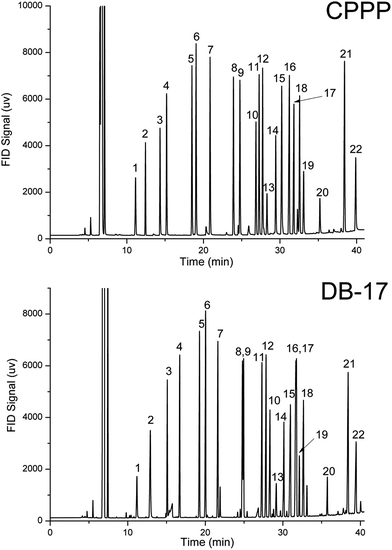 |
| Fig. 7 Separation of polycyclic aromatic hydrocarbons on the CPPP and DB-17 column. Peaks: (1) decahydronaphthalene; (2) tetralin; (3) naphthalene; (4) 2-methylnaphthalene; (5) 1-methylnaphthalene; (6) biphenyl; (7) dibenzyl ether; (8) 8-hydroxyquinoline; (9) acenaphthylene; (10) dihydroacenaphthylene; (11) naphthyl ethyl ether; (12) fluorenone; (13) α-nitronaphthalene; (14) 4-nitrobiphenyl; (15) benzil; (16) phenanthrene; (17) anthracene; (18) phenanthrenequinone; (19) 1,8-dihydroxyanthracene-9,10-dione; (20) pyrene; (21) 1,2-benzanthracene; and (22) chrysene. Conditions: temperature was increased from 80 °C (maintained for 5 min) to 290 °C at 6 °C min−1. Carrier gas velocity: 10 cm s−1. The injection and detector temperatures were both 320 °C. | |
3.6.4 Separation performance of CPPP for aromatic aldehydes. A complex mixture of 39 different aromatic aldehydes was separated on the CPPP column and on DB-17 column under same condition (Fig. 8). The resolution of the dense peaks separated by the CPPP column was mostly superior to that of the DB-17 column because of the sensitive dipole–induced dipole and π–π interaction between the CPPP phase and aromatic aldehydes as compared with DB-17. In terms of the elution order, the retention time of several analytes with a linear alkyl substitute, e.g., cis-citral (peak 20; b.p., 117 °C under 2.67 kPa) and trans-citral (peak 23; b.p., 118 °C under 2.67 kPa), were evidently increased on CPPP, thereby suggesting the specific shape selectivity of CPPP and its different interactions with analytes as compared with the conventional phase. Another interesting phenomenon was that the halogenated aromatic aldehydes, e.g., 2,4-dichlorobenzaldehyde (peak 24; b.p., 233 °C) and 3,4-dichlorobenzaldehyde (peak 25; b.p., 247 °C), were retained longer on CPPP. This phenomenon can be explained by the induced polarization of cyano-substituted tetraphenyl-phenyl groups, which contain mobile π-bonding electrons and may acquire dipole moments when exposed to polar compounds, such as halogenated aromatic aldehydes.
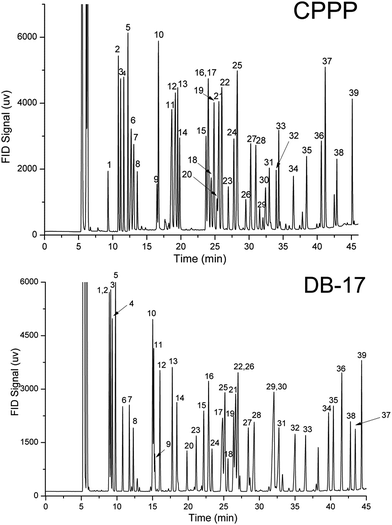 |
| Fig. 8 Separation of aromatic aldehydes on the CPPP and DB-17 columns. Peaks: (1) 2-furaldehyde; (2) 3,5-difluorobenzaldehyde; (3) 2-fluoro-5-trifluoromethylbenzaldehyde; (4) 3,4,5-trifluorobenzaldehyde; (5) benzaldehyde; (6) 4-fluorobenzaldehyde; (7) 2-trifluoromethylbenzaldehyde; (8) phenylacetaldehyde; (9) 2-amino-benzaldehyde; (10) 4-methylbenzaldehyde; (11) 2-hydroxybenzaldehyde; (12) 2-ethylbenzaldehyde; (13) 2-chlorobenzaldehyde; (14) 4-chlorobenzaldehyde; (15) 2-bromobenzaldehyde; (16) 4-bromobenzaldehyde; (17) 2-cyanobenzaldehyde; (18) 1,2-benzenedialdehyde; (19) 1,4-benzenedialdehyde; (20) cis-citral; (21) 3-cyanobenzaldehyde; (22) 4-methoxybenzaldehyde; (23) trans-citral; (24) 2,4-dichlorobenzaldehyde; (25) 3,4-dichlorobenzaldehyde; (26) 2-nitrobenzaldehyde; (27) 3-nitrobenzaldehyde; (28) 4-nitrobenzaldehyde; (29) 4-hydroxybenzaldehyde; (30) 3-phenyl-2-propenal; (31) 3,5-bis(trifluoromethyl)benzaldehyde; (32) 2-chloro-4-fluoro-5-nitrobenzaldehyde; (33) 4-hydroxy-3-methoxybenzaldehyde; (34) 4-chloro-3-nitrobenzaldehyde; (35) 2,4-dimethoxybenzaldehyde; (36) 4-dimethylaminobenzaldehyde; (37) 3,4,5-trimethoxybenzaldehyde; (38) 2,4-dinitrobenzaldehyde; and (39) 4-fluoro-3-phenoxybenzaldehyde. Conditions: temperature was increased from 100 °C (maintained for 5 min) to 170 °C at 4 °C min−1 (maintained for another 5 min) and then to 280 °C at 6 °C min−1. Carrier gas velocity: 10 cm s−1. The injection and detector temperatures were both 320 °C. | |
3.7 Column repeatability and reproducibility
Column repeatability (month-to-month) and reproducibility (column-to-column) of CPPP was evaluated by separation of several analytes of different varieties at 120 °C. The columns have been in use during storage. As obtained in Table 3, with relative standard deviations (RSD%) of retention time below 0.63% for month-to-month and 4.29% for column-to-column, the CPPP column is well repeatable and reproducible.
Table 3 Repeatability of retention times on CPPP column
Analytes |
Month-to-month (n = 4) |
Column-to-column (n = 3) |
Mean |
RSD (%) |
Mean |
RSD (%) |
Heptane |
5.552 |
0.19 |
5.559 |
2.82 |
Butyl acetate |
6.108 |
0.51 |
6.127 |
4.29 |
Chlorobenzene |
6.962 |
0.26 |
6.959 |
2.75 |
Bromobenzene |
7.988 |
0.17 |
7.993 |
2.36 |
Octanol |
9.859 |
0.39 |
9.856 |
4.11 |
p-Cresol |
11.138 |
0.63 |
11.141 |
3.59 |
Acetophenone |
12.051 |
0.32 |
12.120 |
3.14 |
4 Conclusion
This paper reports that the synthesized CPPP stationary phase possesses good film-forming ability, medium polarity, high column efficiency, high thermal stability and good repeatability. The stationary phase also exhibits good resolution and excellent selectivity in separating Grob test mixtures, alcohols, substituted benzenes, PAHs and aromatic aldehydes. The present work demonstrates that CPPP are highly selective GC stationary phases and promises well for high-resolution separation of analytes.
Acknowledgements
This study was supported by the National Natural Science Foundation of China (No. 21275090) and Technology Development Plan of Shandong Province (No. 2014GGX107010).
References
- B. X. Mayer, P. Zöllner and E. Lorbeer, J. Sep. Sci., 2002, 25, 60–66 CrossRef CAS.
- P. A. Peaden, B. W. Wright and M. L. Lee, Chromatographia, 1982, 15, 335–340 CAS.
- Z. Juvancz, M. A. Pulsipher and B. J. Tarbet, J. Microcolumn Sep., 1989, 1, 142–149 CrossRef CAS.
- M. L. Lee, J. C. Kuei and N. W. Adams, J. Chromatogr. A, 1984, 302, 303–318 CrossRef CAS.
- A. Malik, S. L. Reese and S. Morgan, Chromatographia, 1997, 46, 79–84 CAS.
- K. J. Miller and J. Savchik, J. Am. Chem. Soc., 1979, 101, 7206–7213 CrossRef CAS.
- P. C. Zhao, S. Teng, M. Yu, N. Niu, X. X. He and B. Wu, Anal. Methods, 2015, 7, 1333–1338 RSC.
- X. Han, X. X. He, H. Wang, B. Wang and B. Wu, J. Chromatogr. A, 2016, 1449, 118–128 CrossRef CAS PubMed.
- P. C. Zhao, Y. Niu and B. Wu, RSC Adv., 2015, 5, 22399–22404 RSC.
- H. C. Ren, Q. Tao, Z. X. Gao and D. Liu, Dyes Pigm., 2012, 94, 136–142 CrossRef CAS.
- Silicone Polymers and Their Applications, ed. S. Y. Feng, J. Zhang, M. J. Li and Q. Z. Zhu, Chemical Industry Press, Beijing, 2004, pp. 79–80 Search PubMed.
- B. X. Mayer, W. Rauter and G. Rauchbauer, J. Sep. Sci., 2004, 27, 335–342 CrossRef PubMed.
- L. Wang, X. Wang and M. Qi, J. Chromatogr. A, 2014, 1334, 112–117 CrossRef CAS PubMed.
- P. Ma, X. Cai, Y. Zhang, S. Wang, W. Dong, M. Chen and P. J. Lemstra, Polym. Degrad. Stab., 2014, 102, 145–151 CrossRef CAS.
- J. L. Anderson and D. W. Armstrong, Anal. Chem., 2005, 77, 6453 CrossRef CAS PubMed.
- M. H. Abraham, Chem. Soc. Rev., 1993, 22, 73–83 RSC.
- M. H. Abraham, C. F. Poole and S. K. Poole, J. Chromatogr. A, 1999, 842, 79–114 CrossRef CAS.
- C. F. Poole and S. K. Poole, J. Chromatogr. A, 2008, 1184, 254–280 CrossRef CAS PubMed.
- W. O. McReynolds, J. Chromatogr. Sci., 1970, 8, 685–691 CrossRef CAS.
- E. Hedaya, J. H. Kawakami, P. W. Kopt, G. T. Kwiatkowski, D. W. Mcneil, D. A. Owen, E. N. Peters and R. W. Tulis, J. Polym. Sci., Polym. Chem. Ed., 1977, 15, 2229–2238 CrossRef CAS.
- M. H. Abraham, G. S. Whiting, R. M. Doherty and W. J. Shuely, J. Chromatogr. A, 1991, 587, 213–228 CrossRef CAS.
- M. H. Abraham, A. Ibrahim and A. M. Zissimos, J. Chromatogr. A, 2004, 1037, 29–47 CrossRef CAS PubMed.
- X. Wang, M. Qi and R. Fu, J. Chromatogr. A, 2014, 1371, 237–243 CrossRef CAS PubMed.
- J. Fan, Z. Wang and M. Qi, J. Chromatogr. A, 2014, 1362, 231–240 CrossRef CAS PubMed.
- B. X. Mayer and E. Lorbeer, J. High Resolut. Chromatogr., 1995, 18, 504–506 CrossRef CAS.
- A. J. King, J. W. Readman and J. L. Zhou, Anal. Chim. Acta, 2004, 523, 259–267 CrossRef CAS.
- S. Sremac, A. Popović and Ž. Todorović, Talanta, 2008, 76, 66–71 CrossRef CAS PubMed.
|
This journal is © The Royal Society of Chemistry 2016 |