DOI:
10.1039/C6RA17377B
(Paper)
RSC Adv., 2016,
6, 85787-85794
Detection of cyanide by a novel probe with a V-shaped structure based on aggregation of the probe adduct†
Received
7th July 2016
, Accepted 25th August 2016
First published on 25th August 2016
Abstract
2-[2-[4-(Dimethylamino)phenyl]phenylethenyl]-1,3,3-trimethyl-3H-indolium iodide, a turn-on fluorescent probe for detection of the cyanide anion in aqueous solution with a novel V-shaped structure, is synthesized and characterized. Due to the considerably localized HOMO and LUMO on different “arms” of the probe, its fluorescence is completely quenched in aqueous solution caused by a strong ICT effect and intramolecular rotation of dimethylaminophenyl. However, the emission is dramatically intensified over 500 times when the probe is exposed to cyanide anions and the turn-on fluorescence should be ascribed to the aggregation of the probe adduct in aqueous solution. The probe, which displays a high selectivity and sensitivity for CN− against other analytes, can finish the response within 20 s and the detection limit is measured to be as low as 55 nM. These merits make our cyanide probe one of the most effective ones ever reported.
1 Introduction
It is well-known that the cyanide anion (CN−) is a highly toxic species, whose fatal dosage for human beings is reported to be as low as 0.5–3.5 mg per kilogram of body weight.1 According to the provision of the World Health Organization (WHO), water only with a CN− concentration lower than 1.9 μM is safe for drinking.2 However, due to high nucleophilic reactivity and special properties, cyanides are widely used in electroplating, metallurgy, synthetic fibres, resins, production of pharmaceutical intermediates as well as gold mining. The multiple channels and large amount of cyanides used make our living environment exposed to the risk of their pollution. Therefore, probes with the advantages of rapid response, low detection limit, excellent selectivity and sensitivity, ready accessibility of starting materials, convenient preparation and outstanding compatibility to aqueous solution, are still highly required. Compared with some reported CN− probes with turn-off fluorescence,3 those with turn-on4 or ratiometric fluorescence5 have the merits of weakened photo-bleaching and diminished interference of background emission. Among such CN− probes with turn-on fluorescence, however, very limited cases based on aggregation-induced emission (AIE) mechanism have been reported.6
Recently, AIE-active molecules have been turned up as the focus in fields of chemical, biological, materials science and other ever expanding multidisciplinary arenas. At the single molecular level, the most popular AIE mechanism is explained by the restriction from intramolecular rotation (RIR) of non-conjugated σ-bonds in condensed phase.7 Other mechanisms such as formation of hydrogen bond,8 restriction of twisted intramolecular charge transfer (TICT)9 or the flipping of flexible alicyclic components10 should also account for some AIE phenomena. At the supramolecular level, AIE effect is closely related to the molecular packing patterns. Some specific types of molecular packing such as J-aggregation,11 cross packing,12 herringbone stacking13 and dimer/excimer stacking14 are also favourable for generating AIE-active compounds.
V-shaped molecules, which can be considered as half-cut X-shaped cruciforms, display the inherent photophysical features similar to their cruciform parents.15 In case where an electron-donating and an electron-withdrawing group are respectively imposed on the different arms of a V-shaped molecule, considerably localized distribution of electrons on HOMO and LUMO will result in strong intramolecular charge transfer (ICT) effect, just as that commonly occurs on its X-shaped cruciform parents. Moreover, highly twisted conformation caused by spatially repulsed arms on a V-shaped molecule hardly results in packing with strong and dense π–π interactions, which also resembles to that taking place on its cruciform parents. Hence, V-shaped molecules are still intensely emissive in aggregation state.
Thanks to long emission wavelength, specific binding site and satisfactory hydrophilicity, a positively charged indolium or benzo[e]indolium moiety is frequently used to construct ICT-active probes,16 whose emission intensity is usually dramatically reduced in a highly polar solvent. Herein we describe a novel V-shaped CN− probe 2-[2-[4-(dimethylamino)phenyl]phenylethenyl]-1,3,3-trimethyl-3H-indolium iodide, whose the electron-rich dimethyl-aminophenyl and the electron-deficient benzo[e]indolium are respectively introduced on the two arms of the probe. Due to the strong ICT effect along with the intramolecular rotation of dimethylaminophenyl, this probe is completely non-emissive in aqueous solution, but the fluorescence is drastically turned on when it is exposed to cyanide anion. It is verified that the turn-on emission should be attributed to the aggregation of the probe adduct in aqueous solution. Moreover, the excellent sensitivity and selectivity, very short response time and extremely low detection limit to cyanide anion also make our probe greatly attractive.
2 Results and discussion
2.1 Synthesis
The preparation of the probe can be readily fulfilled via a two-step procedure involving Suzuki coupling and Knoevenagel reaction (Scheme 1). Under the common conditions of Suzuki coupling, 4′-(dimethylamino)-[1,1′-biphenyl]-2-carbaldehyde (1) can be obtained in 83% yield by the reaction of 2-bromo-benzaldehyde and N,N-dimethyl-4-(4,4,5,5-tetramethyl-1,3,2-dioxa-borolan-2-yl)aniline with 5% mol Pd(PPh3)4 as the catalyst and Cs2CO3 as the base. The following Knoevenagel condensation between 1 and 1,2,3,3-tetramethylindolium iodide can be smoothly carried out without any catalyst, providing the probe as a dark red microcrystal which is entirely non-emissive under UV light (Fig. 1D). The purified 1 and probe are characterized by 1H, 13C-NMR and EI-MS spectroscopy and the satisfactory data are obtained (ESI†).
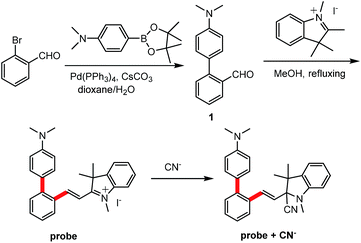 |
| Scheme 1 Synthetic route to probe and its reaction with cyanide. | |
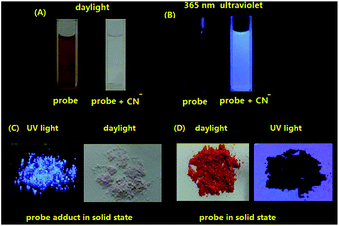 |
| Fig. 1 Photos of probe (50 μM) in water in absence (left) or presence (right) of CN− solution (150 μM) under daylight (A) and UV light (B); photo of probe adduct in solid state under UV light (C); photos of probe in solid state under UV and day light (D). | |
2.2 Study on the reaction of probe with CN−
Under daylight and observed by the naked eyes, the aqueous solution of the probe is brownish yellow and it rapidly fades into colourless when exposed to CN− solution (Fig. 1A). However, the aqueous solution of the probe which is entirely non-emissive under UV light gives out intensely blue light in the presence of cyanide anion (Fig. 1B). When the probe solution exposed to CN− is irradiated by a laser beam, a bright light path across a cell turns up (Tyndall effect), which indicates that a nanoparticle system is generated for the probe adduct in aqueous solution. In contrast, Tyndall phenomenon does not appear in a cell containing the probe solution in absence of CN− (Fig. 2). Dynamic light scattering (DLS) measurement confirms that nanoparticles with averaged diameter in 310.3 nm are formed for the probe adduct derived from probe (20 μM) and cyanide anion (60 μM) in aqueous solution (Fig. 3). Hence, the intense emission in aqueous solution should be undoubtedly ascribed to the aggregation of the probe adduct.
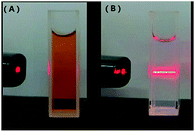 |
| Fig. 2 Photos of probe (20 μM) in absence (A, without Tyndall effect) or presence (B, Tyndall effect) of cyanide solution (60 μM) under laser irradiation. | |
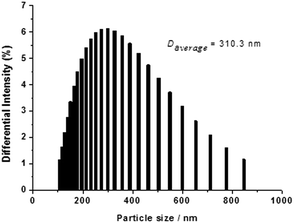 |
| Fig. 3 Size distribution by DLS measurement of probe (20 μM) exposed to cyanide anion (60 μM) in water. | |
2.2.1 Kinetic behaviour. In order to determine the response time of the probe to cyanide anion in aqueous solution, a curve of fluorescence intensity at 435 nm versus reaction time in a 0.06 s acquisition interval is plotted (Fig. 4). It is indicated that the reaction rate between probe (20 μM) and CN− (60 μM) is so fast that it just takes 15 s for the curve to reach a plateau, which suggests that the reaction can be finished within 15 s. Hence, the time is prolonged to 1 min in the following measurements to ensure the reaction is fully completed. Compared with many other reported CN− probes,4a,b,5a,17 the response time of our V-shaped probe is extremely short. However, a first-order kinetic equation is unable to be fitted according to the presented curve in Fig. 4.
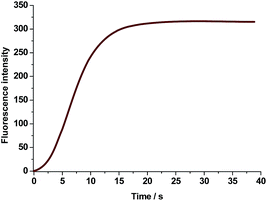 |
| Fig. 4 Fluorescence intensity (at 435 nm) of probe (20 μM) in presence of cyanide solution (60 μM) with varied reaction time (acquisition interval: 0.06 s). | |
2.2.2 Spectral titration of probe. Fig. 5 illustrates the UV-Vis spectra of the probe (20 μM) exposed to various amounts of CN− in aqueous solution (0–100 μM). When the CN− concentration is less than 60 μM, the absorption peak of the probe at 380 nm is gradually reduced while the peak of the probe adduct at 315 nm continuously rises. When the CN− concentration is more than 60 μM, the absorption of the probe is fully vanished while the absorption of the probe adduct does not make increase any more. This result suggests that 3 eq. cyanide anion is the enough amounts for the reaction to be finished. A well-defined isobestic point at 353 nm is clearly turned up on the UV-Vis spectra. Notably, as the CN− concentration is progressively increased, the level-off tails at long-wavelength region can be readily observed, which should be attributed to the well-known Mie effect caused by the scattering of nanoparticles.18 Hence, a stable nanoparticle system of the probe adduct is confirmed to occur in aqueous solution.
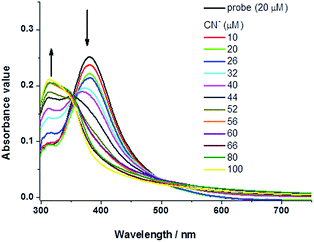 |
| Fig. 5 Absorption spectra of probe (20 μM) in water upon addition of cyanide aqueous solution at varied concentration. | |
Similarly, the fluorescence spectra of the probe (20 μM) in the presence of CN− aqueous solution (0–180 μM) are depicted in Fig. 6. It is shown that the emission intensity of the probe adduct at 435 nm is largely boosted when the CN− concentration is less than 60 μM, but it does not make any obvious increase when the CN− concentration is more than 60 μM.
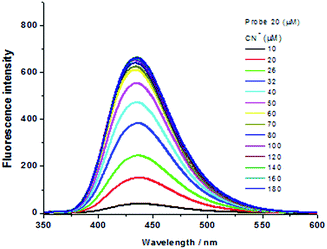 |
| Fig. 6 Emission spectra (λex = 315 nm) of probe (20 μM) in water upon addition of cyanide solution at varied concentration. | |
Consequently, a curve of fluorescence intensity at 435 nm versus cyanide concentration is plotted in Fig. 7. It is evidently shown that the probe consumes no more than 3 eq. CN− to complete the reaction and such amount is in accordance with that determined by UV-Vis spectra. Due to the entirely quenched background fluorescence of the probe in aqueous solution, the emission intensity is increased over 500 times when it is exposed to 3 eq. cyanide anion. Moreover, the emission intensity of probe adduct at 435 nm is in a linear variation at the low concentration range of CN− (Fig. 7, inserted part), and the detection limit (3σ/ρ, where σ is the standard deviation for the measured emission intensity of the aqueous probe solution by 20 times; ρ is the slope of the calibration curve) is estimated to be 55 nM, which is much lower than the maximum level of CN− concentration (1.9 μM) in drinkable water permitted by the WHO. Although there are some reported CN− probes with the detection limit ≤100 nM,4h,5a,15a,17a,e,f,19 the one in association with response time within 20 s is greatly scarce.6d Hence, our novel V-shaped probe is an excellent candidate for rapid and sensitive detection of cyanide anion in aqueous solution.
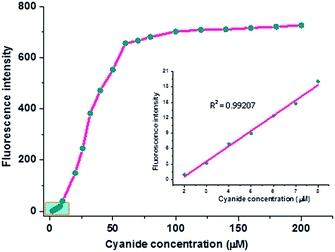 |
| Fig. 7 Fluorescence intensity (at 435 nm) of probe (20 μM) in water upon addition of cyanide aqueous solution at varied concentration [insert: fluorescence intensity of probe (20 μM) in presence of cyanide solution at low concentration]. | |
2.2.3 Selectivity of probe. The experiments involving the selectivity of the probe (20 μM) to other common anions (200 μM) in aqueous solution are carried out and the results are summarized in Fig. 8. Clearly, for CN−, CO32−, PO43−, SCN−, HS−, S2−, SO42−, HSO42−, S2O32−, HPO42−, H2PO4−, F−, Cl−, Br−, I−, N3−, NO2−, NO3−, HCO3−, AcO−, HSO3−, SO32− and ClO4−, only CN− can make the probe produce the drastically enhanced emission intensity. Although the probe can generate the weak fluorescence in response to both CO32− and PO43−, the emission of these two adducts is located at 410 nm, which is easily distinguished from that of CN− adduct at 435 nm (Fig. 9). The competition between cyanide and other anions is also determined. It is verified that the emission intensity of the probe exposed to CN− (60 μM) is not affected by the other coexisting analytes (200 μM) (Fig. 8). Therefore, our probe can be used as a highly selective sensor to CN− in aqueous medium.
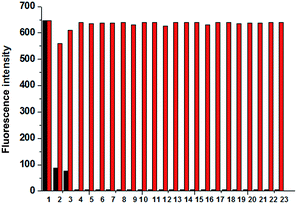 |
| Fig. 8 Fluorescence intensity (λem = 435 nm) of probe (20 μM) in water upon addition of aqueous cyanide solution (60 μM) and other analytes (200 μM). Black bar: probe + each analyte; red bar: probe + each analyte + cyanide. λex = 315 nm. 1–23: CN−, CO32−, PO43−, SCN−, HS−, S2−, SO42−, HSO42−, S2O32−, HPO42−, H2PO4−, F−, Cl−, Br−, I−, N3−, NO2−, NO3−, HCO3−, AcO−, HSO3−, SO32−, ClO4−. | |
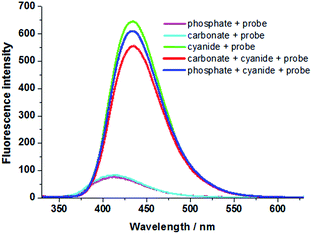 |
| Fig. 9 Emission spectra (λex = 315 nm) of probe (20 μM) in water upon addition of cyanide (60 μM) and other analytes (200 μM). | |
2.2.4 Optical properties of the probe adduct. The probe adduct is white powder which emits brightly blue fluorescence under UV light (Fig. 1C), and its solid-state quantum yield measured by an integral sphere is 0.31. It is demonstrated (Fig. 10) that the absorption spectra of the adduct are inert to the change of solvent polarity, as almost identical λab and spectral profiles are obtained in hexane, dichloromethane and ethanol. Such insensitivity implies that the ground state is a species with a very small dipole moment. In dichloromethane, the molar extinction coefficient is determined to be 2.11 × 104 L mol−1 cm−1. In contrast, the emission spectra of the probe adduct make an obvious red shift as the polarity of the solvents is enhanced (Fig. 10), which indicates that the dipole moment of the excited state is much larger than that of the ground state and ICT effect still takes place in the excited state. Notably, for the probe adduct, the characteristics of both absorption and emission spectra in organic solutions are remarkably distinct from those in aqueous solution, as the optical properties of isolated molecules and aggregated ones are very much different.
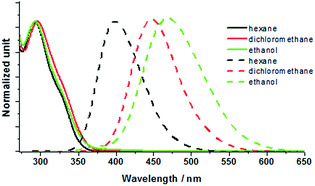 |
| Fig. 10 Absorption (solid lines) and emission spectra (dash lines) of probe adduct in different solvents. | |
2.3 Test on filter paper strips
The photos of our probe exposed to common anion solutions on filter paper strips are presented in Fig. 11. The neutral filter paper strips soaked with an aqueous solution of the probe (50 μM) are initially dried under vacuum and they turn to be pink. Subsequently, the aqueous solutions containing various anions (200 μM) are carefully dropped onto these strips. Under daylight, the colour of the spot exposed to CN− solution on the modified filter paper strip rapidly fades from pink to white. Under UV light, the same spot emits brightly blue fluorescence. However, solutions containing other common anions are unable to make the strips produce the same positive response. Hence, this cheap and use-and-throw method is very convenient for in situ detection of CN− in aqueous solution.
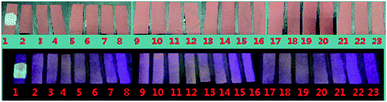 |
| Fig. 11 Photos of filter paper strips soaked with probe solution (50 μM) in response to various analytes (200 μM) under daylight (upper) and UV light (bottom). 1–23: CN−, CO32−, PO43−, SCN−, HS−, S2−, SO42−, HSO42−, S2O32−, HPO42−, H2PO4−, F−, Cl−, Br−, I−, N3−, NO2−, NO3−, HCO3−, AcO−, HSO3−, SO32−, ClO4−. | |
2.4 AIE mechanism
The density functional theory (DFT) calculations based on Gaussian 09W software package20 and its accompanying graphical interface program GaussView 5.0 at B3LYP/6-31G(d,p) level is carried out to explore the reason for the completely quenched fluorescence of the probe in aqueous solution. Like many other cruciforms with D–π–A design,21 our V-shaped probe also exhibits the considerably localized FMOs (Fig. 12A): most of the LUMO density is placed along the electron-deficient indolium-bearing arm, while most of the HOMO density resides along the electron-rich dimethylaminophenyl group. For the two FMOs, the HOMO is a little more localized than the LUMO. In light of the spatially separated FMOs in the probe, excited-state species with a strong ICT effect should occur, by which dramatically decreased quantum yield will be induced in a solvent with high polarity. Moreover, for the isolated molecules of the probe in aqueous solution, the rotation of dimethylaminophenyl group around C2–C3 σ bond facilitates the decay of the excited energy. Hence, the associated two effects make the fluorescence of our V-shaped probe completely quenched in aqueous solution.
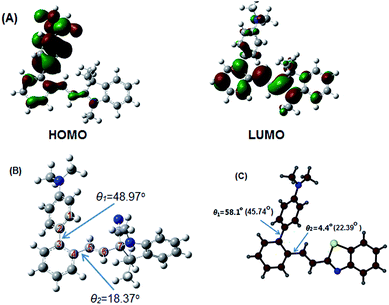 |
| Fig. 12 DFT-calculated electron distribution on FMOs of probe (A), DFT-calculated geometry of probe adduct (B) and X-ray crystallographic geometry of (E)-2′-[2-(benzo[d]thiazol-2-yl)vinyl]-N,N-dimethyl-(1,1′-biphenyl)-4-amine (C). | |
Due to the activation of the double bond by the electron-withdrawing N+ on the indolium moiety, a 1,2-nucleophilic addition with CN− can readily occur at C7 atom, which transforms indolium into indoline. The structure of the probe adduct is characterized by 1H, 13C-NMR and EI-MS (ESI). Hence, the solubility of the probe adduct in aqueous solution becomes very poor due to the disappearance of the hydrophilic indolium moiety, and molecules have to be aggregated in such medium.
In literature, most compounds with intense emission in aggregation state have highly twisted molecular conformations,22 by which strong and dense π–π interactions are not readily formed. For our probe adduct, a single crystal suitable for X-ray diffraction cannot be gained in spite of our most effort. We have to rely on DFT calculations to get its geometry (Fig. 12B). It is illustrated that the torsion angles θ1 and θ2 are respectively 48.97 and 18.37° in gas state.
Although it is well known that the geometry of a molecule in gas state is definitely different from that in solid state due to multiple intermolecular interactions, a good co-planarity between dimethylaminophenyl and phenyl ring on styryl moiety cannot be yet predicted in aggregation state. Recently, we obtained the X-ray crystallography of (E)-2′-[2-(benzo[d]thiazol-2-yl)vinyl]-N,N-dimethyl-(1,1′-biphenyl)-4-amine (CCDC 1480706), a structural analogue to the probe adduct, and made the comparison of its geometry with that provided by DFT calculations (Fig. 12C). It is revealed that θ1 is increased from 45.74° in gas phase to 58.1° in crystalline phase, while θ2 is reduced from 22.39° to 4.4°. This analogue is highly emissive in solid state (Φsolid = 0.58) due to the shortage of π–π overlaps in molecular packing. However, the linear isomer of such analogue (Scheme 2) is completely dark in solid state (Φsolid = 0.05)23 due to the strong π–π interactions caused by the linear arrangement of the electron-donating and electron-withdrawing groups on the phenyl core. Clearly, the highly twisted conformation caused by the V-shaped array of dimethylamino-phenyl and 2-benzo[d]thiazolovinyl on a phenyl core should account for the intensely solid-state emission of the analogue. Hence, it should be safe to deduce that the strong aggregation-induced emission of our probe adduct in aqueous solution and solid state (Fig. 1C) is ascribed to the same V-shaped skeleton as its analogue.
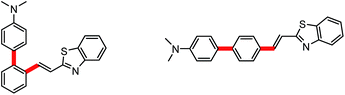 |
| Scheme 2 (E)-2′-[2-(Benzo[d]thiazol-2-yl)vinyl]-N,N-dimethyl-(1,1′-biphenyl)-4-amine (left) and its linear isomer (right). | |
3 Conclusion
Herein we describe a novel CN− probe with V-shaped structure and the turn-on fluorescence should be ascribed to the aggregation of the probe adduct in aqueous solution. Due to the strong ICT effect together with the intramolecular rotation of dimethylaminophenyl, the background emission of the hydrophilic probe is completely quenched in aqueous solution. However, the fluorescence intensity of the probe adduct is impressively enhanced by at least 500 times, which ensures that the detection limit can be as low as 55 nM. It is deduced that the V-shaped array of dimethylaminophenyl and 2-indolinovinyl on a phenyl core is the key for the intense emission of the probe adduct in aggregation state. Additionally, the very short response time within 20 s along with excellent selectivity against other common 22 anions makes our probe much more effective than most of ever reported CN− probes. The application of it on filter paper strips develops a cheap and use-and-throw material for the convenient and rapid in situ detection of cyanides. The further research on its use as a probe for imaging of CN− in living cells will be reported under the due course.
4 Experimental section
4.1 Materials and instruments
All reagents and solvents are purchased from commercial source and used without further purification, if not stated. All reactions are carried out on the magnetic stirrers and their reaction process is monitored on thin layer chromatography (TLC). Stock solutions (10 mM) of cyanide anion and other analysts are prepared by direct dissolution of proper amounts of sodium or potassium salts with deionized water. The probe is initially dissolved in DMSO to be 10 mM and 0.2 mL of the resulted solution is diluted with deionized water until the total volume reaches to 100 mL. The aqueous solution of the probe prepared by such method is 20 μM with 99.8% water fraction, which is used in the aforementioned measurements.
Melting points were determined by an OptiMelt automated melting point system and the thermometer was uncorrected. The 1H NMR (400 MHz) and 13C NMR (100 MHz) spectra were obtained on a Bruker Avance II DMX 400 spectrometer with CDCl3 or DMSO-d6 as the solvent. The absorption spectra were measured on a Shimadzu UV 2501(PC)S UV-Vis spectrometer, and the fluorescence spectra were acquired on a Perkin-Elmer LS55 spectrophotometer, and the solid-state quantum yield of probe adduct was determined by an integral sphere. The mass spectrum was recorded on a HP 1110 mass spectrometer.
4.2 Computational method
The gas-phase geometries of the concerned compounds were optimized without any symmetry restrictions in singlet ground state using the density functional theory (DFT) method at the B3LYP level. The 6-31G(d,p) basis set was selected for all the elements. The vibration frequency calculations were performed to ensure that the optimized geometries represented the global minima on the ground-state potential energy surface. All the calculations were carried out with the Gaussian 09 program package in aid of the GaussView visualization program.
4.3 Preparation of the probe
4.3.1 4′-(Dimethylamino)-[1,1′-biphenyl]-2-carbaldehyde (1). At room temperature and nitrogen atmosphere, N,N-dimethyl-4-(4,4,5,5-tetramethyl-1,3,2-dioxa-borolan-2-yl)aniline (20 mmol, 4.92 g) in dioxane/H2O (20 mL, 4
:
1 v/v) is injected into the mixture of 2-bromo-benzaldehyde (18 mmol, 3.31 g), Cs2CO3 (20 mmol, 3.88 g) and Pd(PPh3)4 (0.9 mmol, 1.07 g) in dioxane/H2O (30 mL, 4
:
1 v/v). The resulted mixture is heated at 90 °C for 16 h and then cooled to room temperature. After filtration, the filtrate is diluted with water (150 mL) and extracted by ethyl acetate (3 × 20 mL). The combined organic layers are dried over anhydrous Na2SO4 and concentrated on a rotating evaporator. The residue is purified on a silica gel column with hexane/ethyl acetate (4
:
1) as the eluent, providing the product in 83% yield as the yellowish powder. Mp 77.4–79.3 °C; 1H NMR (400 MHz, CDCl3) δ 2.93 (s, 6H), 6.71 (d, J = 7.6 Hz, 2H), 7.17 (d, J = 8.0 Hz, 2H), 7.31–7.37 (m, 2H), 7.50 (t, J = 7.6 Hz, 1H), 7.79 (d, J = 8.0 Hz, 1H), 9.94 (s, 1H); 13C NMR (100 MHz, CDCl3) δ 40.58, 112.05, 126.63, 127.54, 130.63, 131.10, 133.42, 133.61, 146.30, 150.26, 193.54; EI-MS (70 eV) m/z (%) 225(M+, 100), 196(45), 182(29), 167(12), 152(49), 141(13), 128(17), 115(17), 69(23), 51 (25), 42(47).
4.3.2 2-[2-[4-(Dimethylamino)phenyl]phenylethenyl]-1,3,3-trimethyl-3H-indolium iodide (probe). At room temperature, the solution of 1,2,3,3-tetramethylindolium iodide (20 mmol, 6.02 g) in methanol (20 mL) is dropped into the solution of 1 (18 mmol, 4.05 g) in methanol (20 mL). The resulted solution is refluxed for 24 h and cooled to room temperature. The precipitate is filtrated and recrystallized with methanol, providing the product in 63% yield as the dark lamellar crystal. Mp 234.2–235.9 °C; 1H NMR (400 MHz, DMSO-d6) δ 1.53 (s, 6H), 3.00 (s, 6H), 4.15 (s, 3H), 6.87 (d, 2H, J = 8.4 Hz), 7.25 (d, 2H, J = 8.8 Hz), 7.52–7.69 (m, 5H), 7.73 (d, 1H, J = 16.4 Hz), 7.81 (d, 1H, J = 7.2 Hz), 7.90 (d, 1H, J = 7.2 Hz), 8.14 (d, 1H, J = 16.4 Hz), 8.38 (d, 1H, J = 7.6 Hz); 13C NMR (100 MHz, DMSO-d6) δ 25.28, 34.62, 51.86, 112.02, 113.44, 115.24, 122.82, 125.88, 127.21, 128.25, 129.01, 129.42, 130.46, 130.77, 131.64, 132.81, 141.75, 143.08, 145.17, 150.22, 151.61, 181.66; EI-MS (70 eV) m/z (%) 382(M+ + 1 − I−, 46), 366(100), 352(23), 246(33), 221(44), 208(23), 178(14), 165(17), 158(68), 144(34), 127(13), 115(11).
4.4 Preparation of the adduct (probe + CN−)
At room temperature, the aqueous solution of KCN (10 mmol, 0.65 g) is dropped into the solution of probe (3 mmol, 1.52 g) in acetonitrile and the resulted solution is stirred for 10 min. After diluted by saturated aqueous FeSO4 solution, the mixture is extracted with ethyl acetate and the organic layer is dried over anhydrous Na2SO4. By removal of the solvent on a rotating evaporator, the residue is purified on a silica gel flash column with hexane as the eluent, providing the adduct in 97% yield as the white powder. Mp 152.7–154.1 °C; 1H NMR (400 MHz, CDCl3) δ 1.18 (s, 3H), 1.51 (s, 3H), 2.78 (s, 3H), 2.98 (s, 6H), 6.09 (d, J = 16 Hz, 1H), 6.57 (d, J = 7.6 Hz, 1H), 6.77 (d, J = 8.4 Hz, 2H), 6.84 (t, J = 7.6 Hz, 1H), 7.03 (d, J = 7.2 Hz, 1H), 7.13–7.36 (m, 7H), 7.57 (d, J = 7.2 Hz, 1H); 13C NMR (100 MHz, CDCl3) δ 23.09, 24.74, 31.84, 40.58, 49.22, 80.18, 109.00, 112.18, 117.30, 120.28, 121.73, 123.42, 126.53, 126.92, 128.21, 128.59, 130.38, 130.57, 133.81, 136.36, 136.63, 141.63, 148.72, 149.87; EI-MS (70 eV) m/z (%) 407 (M+, 71), 380(100), 365(77), 350(20), 221(17), 209(18), 183(21), 144(19).
Acknowledgements
We are grateful for the financial support of Zhejiang Provincial Natural Science Foundation of China (LY14B020016).
References
- W. Kaim and B. Schwederski, Bioinorganic Chemistry: Inorganic Elements in the Chemistry of Life, John Wiley & Sons Ltd., England, 1991 Search PubMed.
- Guidelines for Drinking-Water Quality, World Health Organization, Geneva, 1996 Search PubMed.
-
(a) Y. M. Dong, Y. Peng, M. Dong and Y. W. Wang, J. Org. Chem., 2011, 76, 6962–6966 CrossRef CAS PubMed;
(b) J. Yoshino, N. Kano and T. Kawashima, J. Org. Chem., 2009, 74, 7496–7503 CrossRef CAS PubMed;
(c) Y. K. Yang and J. Tae, Org. Lett., 2006, 8, 5721–5723 CrossRef CAS PubMed;
(d) Z. Ekmekci, M. D. Yilmaz and E. U. Akkaya, Org. Lett., 2008, 10, 461–464 CrossRef CAS PubMed.
-
(a) R. Jackson, R. P. Oda, R. K. Bhandari, S. B. Mahon, M. Brenner, G. A. Rockwood and B. A. Logue, Anal. Chem., 2014, 86, 1845–1852 CrossRef CAS PubMed;
(b) K. H. Jung and K. H. Lee, Anal. Chem., 2015, 87, 9308–9314 CrossRef CAS PubMed;
(c) J. Jo, A. Olasz, C. H. Chen and D. Lee, J. Am. Chem. Soc., 2013, 135, 3620–3632 CrossRef CAS PubMed;
(d) M. Jamkratoke, V. Ruangpornvisuti, G. Tumcharern, T. Tuntulani and B. Tomapatanaget, J. Org. Chem., 2009, 74, 3919–3922 CrossRef CAS PubMed;
(e) M. Dong, Y. Peng, Y. M. Dong, N. Tang and Y. W. Wang, Org. Lett., 2012, 14, 130–133 CrossRef CAS PubMed;
(f) J. H. Lee, A. R. Jeong, I. S. Shin, H. J. Kim and J. I. Hong, Org. Lett., 2010, 12, 764–767 CrossRef CAS PubMed;
(g) T. F. Robbins, H. Qian, X. Su, R. P. Hughes and I. Aprahamian, Org. Lett., 2013, 15, 2386–2389 CrossRef CAS PubMed;
(h) Y. Shiraishi, S. Sumiya, K. Manabe and T. Hirai, ACS Appl. Mater. Interfaces, 2011, 3, 4649–4656 CrossRef CAS PubMed;
(i) L. Long, L. Wang, Y. Wu, A. Gong, Z. Da, C. Zhang and Z. Han, Chem.–Asian J., 2014, 9, 3291–3298 CrossRef CAS PubMed.
-
(a) W. C. Lin, S. K. Fang, J. W. Hu, H. Y. Tsai and K. Y. Chen, Anal. Chem., 2014, 86, 4648–4652 CrossRef CAS PubMed;
(b) L. Yang, X. Li, J. Yang, Y. Qu and J. Hua, ACS Appl. Mater. Interfaces, 2013, 5, 1317–1326 CrossRef CAS PubMed;
(c) S. Khatua, D. Samanta, J. W. Bats and M. Schmittel, Inorg. Chem., 2012, 51, 7075–7086 CrossRef CAS PubMed;
(d) X. Wu, B. Xu, H. Tong and L. Wang, Macromolecules, 2011, 44, 4241–4248 CrossRef CAS;
(e) C. L. Chen, Y. H. Chen, C. Y. Chen and S. S. Sun, Org. Lett., 2006, 8, 5053–5056 CrossRef CAS PubMed;
(f) L. Yuan, W. Lin, Y. Yang, J. Song and J. Wang, Org. Lett., 2011, 13, 3730–3733 CrossRef CAS PubMed;
(g) J. Liu, Y. Liu, Q. Liu, C. Li, L. Sun and F. Li, J. Am. Chem. Soc., 2011, 133, 15276–15279 CrossRef CAS PubMed.
-
(a) X. Huang, X. Gu, G. Zhang and D. Zhang, Chem. Commun., 2012, 48, 12195–12197 RSC;
(b) L. Peng, M. Wang, G. Zhang, D. Zhang and D. Zhu, Org. Lett., 2009, 11, 1943–1946 CrossRef CAS PubMed;
(c) Y. Zhang, D. Li, Y. Li and J. Yu, Chem. Sci., 2014, 5, 2710–2716 RSC;
(d) Y. Sun, Y. Li, X. Ma and L. Duan, Sens. Actuators, B, 2016, 224, 648–653 CrossRef CAS.
-
(a) J. Shi, N. Chang, C. Li, J. Mei, C. Deng, X. Luo, Z. Liu, Z. Bo, Y. Q. Dong and B. Z. Tang, Chem. Commun., 2012, 48, 10675–10677 RSC;
(b) Z. Zhao, S. Chen, J. W. Y. Lam, P. Lu, Y. Zhong, K. S. Wong, H. S. Kwoka and B. Z. Tang, Chem. Commun., 2010, 46, 2221–2223 RSC;
(c) D. Jana and B. K. Ghorai, Tetrahedron Lett., 2012, 53, 6838–6842 CrossRef CAS;
(d) R. Misra, T. Jadhav, B. Dhokale and S. M. Mobin, Chem. Commun., 2014, 50, 9076–9078 RSC;
(e) X. Y. Shen, Y. J. Wang, H. Zhang, A. Qin, J. Z. Sun and B. Z. Tang, Chem. Commun., 2014, 50, 8747–8750 RSC;
(f) S. Odabas, E. Tekin, F. Turksoy and C. Tanyeli, J. Mater. Chem. C, 2013, 1, 7081–7091 RSC.
- R.-H. Chien, C.-T. Lai and J.-L. Hong, J. Phys. Chem. C, 2011, 115, 12358–12366 CAS.
-
(a) Y. Qian, M. M. Cai, L. H. Xie, G. Q. Yang, S. K. Wu and W. Huang, ChemPhysChem, 2011, 12, 397–404 CrossRef CAS PubMed;
(b) M. Cai, Z. Gao, X. Zhou, X. Wang, S. Chen, Y. Zhao, Y. Qian, N. Shi, B. Mi, L. Xie and W. Huang, Phys. Chem. Chem. Phys., 2012, 14, 5289–5296 RSC;
(c) Q. C. Yao, X. L. Lu and M. Xia, New J. Chem., 2014, 38, 2693–2700 RSC.
-
(a) K. Perumal, J. A. Garg, O. Blacque, R. Saiganesh, S. Kabilan, K. K. Balasubramanian and K. Venkatesan, Chem.–Asian J., 2012, 7, 2670–2677 CrossRef CAS PubMed;
(b) N. L. Leung, N. Xie, W. Yuan, Y. Liu, Q. Wu, Q. Peng, Q. Miao, J. W. Y. Lam and B. Z. Tang, Chem.–Eur. J., 2014, 20, 15349–15353 CrossRef CAS PubMed;
(c) S. Kumar, P. Singh, A. Mahajan and S. Kumar, Org. Lett., 2013, 15, 3400–3403 CrossRef CAS PubMed;
(d) T. Nishiuchi, K. Tanaka, Y. Kuwatani, J. Sung, T. Nishinaga, D. Kim and M. Iyoda, Chem.–Eur. J., 2013, 19, 4110–4116 CrossRef CAS PubMed;
(e) C. Yuan, S. Saito, C. Camacho, T. Kowalczyk, S. Irle and S. Yamaguchi, Chem.–Eur. J., 2014, 20, 2193–2200 CrossRef CAS PubMed;
(f) C.-X. Yuan, X.-T. Tao, Y. Ren, Y. Li, J.-X. Yang, W.-T. Yu, L. Wang and M.-H. Jiang, J. Phys. Chem. C, 2007, 111, 12811–12816 CrossRef CAS.
-
(a) B.-K. An, S.-K. Kwon, S.-D. Jung and S. Y. Park, J. Am. Chem. Soc., 2002, 124, 14410–14415 CrossRef CAS PubMed;
(b) T. E. Kaiser, H. Wang, V. Stepanenko and F. Würthner, Angew. Chem., Int. Ed., 2007, 46, 5541–5544 CrossRef CAS PubMed;
(c) S. Choi, J. Bouffard and Y. Kim, Chem. Sci., 2014, 5, 751–755 RSC.
-
(a) Z. Xie, W. Xie, F. Li, L. Liu, H. Wang and Y. Ma, J. Phys. Chem. C, 2008, 112, 9066–9071 CrossRef CAS;
(b) Y. Qian, M. Cai, X. Zhou, Z. Gao, X. Wang, Y. Zhao, X. Yan, W. Wei, L. Xie and W. Huang, J. Phys. Chem. C, 2012, 116, 12187–12195 CrossRef CAS.
-
(a) C. J. Bhongale, C.-W. Chang, C.-S. Lee, E. W.-G. Diau and C.-S. Hsu, J. Phys. Chem. B, 2005, 109, 13472–13482 CrossRef CAS PubMed;
(b) Q. C. Yao, D. E. Wu and M. Xia, J. Mol. Struct., 2013, 1042, 78–85 CrossRef CAS.
-
(a) T. L. Zhou, F. Li, Y. Fan, W. F. Song, X. Y. Mu, H. Y. Zhang and Y. Wang, Chem. Commun., 2009, 3199–3201 RSC;
(b) Y. Liu, X. T. Tao, F. Z. Wang, J. H. Shi, J. L. Sun, W. T. Yu, Y. Ren, D. C. Zou and M. H. Jiang, J. Phys. Chem. C, 2007, 111, 6544–6549 CrossRef CAS.
-
(a) L. Bu, M. Sun, D. Zhang, W. Liu, Y. Wang, M. Zheng, S. Xue and W. Yang, J. Mater. Chem. C, 2013, 1, 2028–2035 RSC;
(b) M. Zheng, D. T. Zhang, M. X. Sun, Y. P. Li, T. L. Liu, S. F. Xue and W. J. Yang, J. Mater. Chem. C, 2014, 2, 1913–1920 RSC;
(c) H. Luo, S. Chen, Z. Liu, C. Zhang, Z. Cai, X. Chen, G. Zhang, Y. Zhao, S. Decurtins, S.-X. Liu and D. Zhang, Adv. Funct. Mater., 2014, 24, 4250–4258 CrossRef CAS;
(d) M. A. Saeed, H. T. M. Le and O. Š. Miljanić, Acc. Chem. Res., 2014, 47, 2074–2083 CrossRef CAS PubMed;
(e) X. Zhang, Z. Chi, J. Zhang, H. Li, B. Xu, X. Li, S. Liu, Y. Zhang and J. Xu, J. Phys. Chem. B, 2011, 115, 7606–7611 CrossRef CAS PubMed.
-
(a) Y. Sun, S. Fan, D. Zhao, L. Duan and R. Li, Sens. Actuators, B, 2013, 185, 638–643 CrossRef CAS;
(b) Y. Chen, C. Zhu, Z. Yang, J. Chen, Y. He, Y. Jiao, W. He, L. Qiu, J. Cen and Z. Guo, Angew. Chem., Int. Ed., 2013, 52, 1688–1691 CrossRef CAS PubMed;
(c) L. Zhu, J. Xu, Z. Sun, B. Fu, C. Qin, L. Zeng and X. Hu, Chem. Commun., 2015, 51, 1154–1156 RSC;
(d) F. Huo, J. Kang, C. Yin, J. Chao and Y. Zhang, Sens. Actuators, B, 2015, 215, 93–98 CrossRef CAS.
-
(a) S. Pramanik, V. Bhalla and M. Kumar, ACS Appl. Mater. Interfaces, 2014, 6, 5930–5939 CrossRef CAS PubMed;
(b) L. Wang, J. Zheng, S. Yang, C. Wu, C. Liu, Y. Xiao and Y. Li, ACS Appl. Mater. Interfaces, 2015, 7, 19509–19515 CrossRef CAS PubMed;
(c) H. S. Jung, J. H. Han, Z. H. Kim, C. Kang and J. S. Kim, Org. Lett., 2011, 13, 5056–5059 CrossRef CAS PubMed;
(d) J. Jo and D. Lee, J. Am. Chem. Soc., 2009, 131, 16283–16291 CrossRef CAS PubMed;
(e) Y. Sun, S. Fan, D. Zhao, L. Duan and R. Li, J. Fluoresc., 2013, 23, 1255–1261 CrossRef CAS PubMed;
(f) F. Huo, J. Kang, C. Yin, J. Chao and Y. Zhang, Sens. Actuators, B, 2015, 215, 93–98 CrossRef CAS;
(g) Y. Zhang, D. Li, Y. Li and J. Yu, Chem. Sci., 2014, 5, 2710–2716 RSC.
-
(a) Q. Zhao, J. Zhou, F. L. Zhang and D. Lippens, Mater. Today, 2009, 12, 60–69 CrossRef CAS;
(b) Absorption and scattering of light by small particles, ed. C. F. Bohren and D. R. Huffmann, Wiley-Interscience, New York, 2010 Search PubMed;
(c) H. Xu, X. Chen, S. Ouyang, T. Kako and J. Ye, J. Phys. Chem. C, 2012, 116, 3833–3839 CrossRef CAS.
-
(a) C. Zhou, M. Sun, C. Yan, Q. Yang, Y. Li and Y. Song, Sens. Actuators, B, 2014, 203, 382–387 CrossRef CAS;
(b) K. D. Prasad, N. Venkataramaiah and T. N. G. Row, Cryst. Growth Des., 2014, 14, 2118–2122 CrossRef.
- M. J. Frisch, G. W. Trucks, H. B. Schlegel, G. E. Scuseria, M. A. Robb, J. R. Cheeseman, G. Scalmani, V. Barone, B. Mennucci, G. A. Petersson, H. Nakatsuji, M. Caricato, X. Li, H. P. Hratchian, A. F. Izmaylov, J. Bloino, G. Zheng, J. L. Sonnenberg, M. Hada, M. Ehara, K. Toyota, R. Fukuda, J. Hasegawa, M. Ishida, T. Nakajima, Y. Honda, O. Kitao, H. Nakai, T. Vreven, J. A. Montgomery Jr, J. E. Peralta, F. Ogliaro, M. Bearpark, J. J. Heyd, E. Brothers, K. N. Kudin, V. N. Staroverov, R. Kobayashi, J. Normand, K. Raghavachari, A. Rendell, J. C. Burant, S. S. Iyengar, J. Tomasi, M. Cossi, N. Rega, J. M. Millam, M. Klene, J. E. Knox, J. B. Cross, V. Bakken, C. Adamo, J. Jaramillo, R. Gomperts, R. E. Stratmann, O. Yazyev, A. J. Austin, R. Cammi, C. Pomelli, J. W. Ochterski, R. L. Martin, K. Morokuma, V. G. Zakrzewski, G. A. Voth, P. Salvador, J. J. Dannenberg, S. Dapprich, A. D. Daniels, O. Farkas, J. B. Foresman, J. V. Ortiz, J. Cioslowski and D. J. Fox, Gaussian 09, Revision A.02, Gaussian, Inc., Wallingford CT, 2009 Search PubMed.
-
(a) J. Sun, Y. Dai, M. Ouyang, Y. Zhang, L. Zhan and C. Zhang, J. Mater. Chem. C, 2015, 3, 3356–3363 RSC;
(b) D. Cao, P. Zhang, P. Liu, S. Cui and N. Sun, J. Mol. Struct., 2014, 1076, 396–402 CrossRef CAS;
(c) M. A. Saeed, H. T. M. Le and O. Š. Miljanič, Acc. Chem. Res., 2014, 47, 2074–2083 CrossRef CAS PubMed;
(d) J. Sun, X. Lv, P. Wang, Y. Zhang, Y. Dai, Q. Wu, M. Ouyang and C. Zhang, J. Mater. Chem. C, 2014, 2, 5365–5371 RSC;
(e) A. J. Zucchero, P. L. McGrier and U. H. F. Bunz, Acc. Chem. Res., 2010, 43, 397–408 CrossRef CAS PubMed;
(f) H. Luo, S. Chen, Z. Liu, C. Zhang, Z. Cai, X. Chen, G. Zhang, Y. Zhao, S. Decurtins, S. X. Liu and D. Zhang, Adv. Funct. Mater., 2014, 24, 4250–4258 CrossRef CAS.
-
(a) Y. Hong, J. W. Y. Lama and B. Z. Tang, Chem. Commun., 2009, 4332–4353 RSC;
(b) Y. Q. Dong, J. W. Y. Lama and B. Z. Tang, J. Phys. Chem. Lett., 2015, 6, 3429–3436 CrossRef CAS PubMed;
(c) Y. Hong, J. W. Y. Lama and B. Z. Tang, Chem. Soc. Rev., 2011, 40, 5361–5388 RSC.
- The manuscript including the results of the different solid-state optical features between the V-shaped analogue and its linear isomer is submitted to J. Mater. Chem. C (ID: TC-ART-06-2016-002618).
Footnote |
† Electronic supplementary information (ESI) available. See DOI: 10.1039/c6ra17377b |
|
This journal is © The Royal Society of Chemistry 2016 |