DOI:
10.1039/C6RA14420A
(Paper)
RSC Adv., 2016,
6, 85795-85801
Turn-off–on fluorescence probe based on 3-mercaptopropionic acid-capped CdS quantum dots for selective and sensitive lysozyme detection
Received
3rd June 2016
, Accepted 13th July 2016
First published on 12th August 2016
Abstract
In this work, we presented a novel biosensor for rapid detection of lysozyme. The novel biosensor was based on the fluorescent “turn off–on” of 3-mercaptopropionic acid-capped CdS quantum dots (MPA-CdS QDs). Positively charged hemoglobin (Hb) could bind to MPA-CdS QDs via electrostatic interaction, which leads to the fluorescence of MPA-CdS QDs to “turn off”. Lysozyme is also positively charged, and it can replace Hb on the surface of MPA-CdS QDs. In the presence of lysozyme, the fluorescence of MPA-CdS QDs could be restored due to the displacement of Hb by lysozyme. The relative fluorescence intensity ratio F/F0 (F and F0 is the intensity of the MPA-CdS QDs–Hb system in the presence and absence of lysozyme respectively) showed a good linear relationship with the lysozyme concentration in the range of 15–600 nmol L−1 with a detection limit of 6.3 nmol L−1, the method exhibited remarkable selectivity for lysozyme in the presence of several other proteins. The presented method was cost-effective, convenient, and does not require any complicated synthetic procedures.
Introduction
As a ubiquitous protein, lysozyme is widely distributed in several human body secretions and tissues.1,2 Its primary sequence contains 129 amino acid residues with a molecular weight of approximately 14.4 kD.3 Lysozyme is positively charged at neutral pH, which may lead viruses to lose activity by coupling directly to the negatively charged viral proteins.4 Lysozyme can also lyse certain bacteria by destroying the bacterial cell wall.5,6 The concentrations of lysozyme are about 1.7 μg mL−1 and 0.18 μg mL−1 in serum and urine samples in healthy human adults respectively. However, serum and urine lysozyme levels can significantly elevate to more than 15 μg mL−1 or even 100 μg mL−1 in patients who suffer from monocytic and myelomonocytic leukemia, renal disease, inflammatory bowel disease and sarcoidosis.7–12 The lysozyme level in serum and urine could be adopted as clinical index for these diseases. Therefore, highly sensitive and specific detection of lysozyme is of great significance in diagnosis, treatment and monitoring of various diseases.
Until now, many analytical techniques have been explored for lysozyme detection, including fluorescent detection,13 electrochemical detection,14,15 luminescent detection,16 colorimetric detection,17,18 RLS detection,6 ECL detection,19 impediment detection20 and etc. However, some disadvantages have been found for these methods, such as time consuming manipulations, large-scale and fragile instrumentation and high detection limits.
In the past few years, fluorescence analysis for some biological molecules has shown several unique advantages such as high outstanding sensitivity, less time requiring in preparation and amenability to high-throughput screening.21–23 In the past decade, with the development of nanotechnology, semiconductor quantum dots (QDs), used as fluorescent materials, have excited marvelous interest in the fields of physics, chemistry and biology owing to their ideal optical properties, such as broad absorption spectra coupled with narrow photoluminescent emission spectra, high photostability and large extinction coefficients.24 As a class of fluorescence probe, QDs with variable surface capping ligands have been extensively used for cell labeling, tumor imaging and clinical diagnosis in biology and pharmacy.25 According to the unique properties of QDs, several strategies based on QDs for lysozyme recognition and detection have been developed. For example, water-soluble cysteamine capped CdTe QDs conjugated with lysozyme binding aptamer was constructed for luminescent sensing of lysozyme by forming a ternary self-assembly complex.26 Wu et al. reported a protein-templated synthesis of Mn-doped ZnS QDs by exploring bovine serum albumin as the template. Lysozyme can assemble with BSA to lead to aggregation of QDs and enhance RLS intensity.27
So far, only a few QDs based sensors showed high sensitivity for lysozyme detection. Furthermore, the traditional ways for QDs preparation are time-consuming and strenuous. In this paper, we adopted a convenient and inexpensive approach to synthesize MPA-CdS QDs, whose fluorescence was effectively quenched by hemoglobin (Hb) via electronic transferring. The Hb, which is bound to MPA-CdS QDs, would be replaced by lysozyme. Thus the fluorescence of the system could be recovered after the addition of lysozyme. This detection was based on the strongly electrostatic interaction between positively charged lysozyme and negatively charged MPA-CdS QDs.
Experimental section
Materials
All reagents were of at least analytical grade. The water used in all experiments had a resistivity higher than 18 MΩ cm−1. Cadmium(II) chloride (CdCl2), sodium hydroxide (NaOH), sodium sulfide nonahydrate (Na2S·9H2O), trihydroxymethyl aminomethane (Tris) and hydrochloric acid were purchased from Shanghai Qingxi Technology Co., Ltd. Lysozyme and Hb were purchased from Sangon Biotechnology Co., Ltd. Mercaptosuccinic acid (99%) was purchased from J & K Chemical Co., Ltd. The 0.1 mol L−1 Tris–HCl buffered solution (pH 8.2) was used as the medium for detection process.
Apparatus
The fluorescence spectra were obtained by using a Shimadzu RF-5301 PC spectrofluorophotometer equipped with a xenon lamp using right-angle geometry. UV-vis absorption spectra were obtained by a Varian GBC Cintra 10e UV-vis spectrometer. In both experiments, a 1 cm path-length quartz cuvette was used. FT-IR spectra were recorded by a Bruker IFS66V FT-IR spectrometer equipped with a DGTS detector. All pH measurements were made with a PHS-3C pH meter (Tuopu Co., Hangzhou, China).
Preparation of MPA-CdS QDs
MPA-CdS QDs were synthesized in aqueous solution: 300 μL 2.4 mmol L−1 MPA, 120 μL 50 mmol L−1 CdCl2 and 0.1 mol L−1 Tris–HCl buffer (pH 8.2, 150 μL) were added into 2 mL calibrated test tube, shaken thoroughly for 10 minutes. After that, 90 μL 30 mmol L−1 Na2S was added into the test tube and diluted to 1500 μL with deionized water followed by thoroughly shaking and equilibrated for 15 min. 100 μL MPA-CdS QDs was diluted to 1500 μL by deionized water. The fluorescence spectra were recorded from 405 nm to 650 nm with the excitation wavelength of 340 nm. The slit widths of excitation and emission were both 10 nm.
Quenching effect of Hb on MPA-CdS QDs
The obtained MPA-CdS QDs solution (100 μL), 0.1 mol L−1 Tris–HCl buffer (pH 6.6, 150 μL), and different amount of Hb was added into a 2.0 mL calibrated test tube. Then, the solution was diluted to 1500 μL by deionized water followed by thoroughly shaking and equilibrated for 40 s until the solution was fully mixed. The fluorescence spectra were recorded from 405 nm to 650 nm with the excitation wavelength of 340 nm. The slit widths of excitation and emission were both 10 nm.
Lysozyme detection
15 μmol L−1 Hb was added to a 2 mL test tube containing 100 μL MPA-CdS QDs solution and 10 mmol pH 6.6 Tris–HCl buffer, and the mixture was incubated for 2.5 min. Different concentrations of lysozyme were successively added into the calibrated test tube and diluted to 1.5 mL by deionized water followed by the thoroughly shaking. The fluorescence spectra were recorded from 405 nm to 650 nm with the excitation wavelength of 340 nm. The slit widths of excitation and emission were both 10 nm. The fluorescence (FL) intensity of the maximum emission peak was used for the quantitative analysis of the target molecules.
Real sample assay
For serum samples detection, drug-free human blood samples were collected from healthy volunteer at the Hospital of Changchun China–Japan Union Hospital. All experiments were performed in compliance with the relevant laws and institutional guidelines. All the blood samples were obtained through venipuncture and centrifuged at 10
000 rpm for 10 min after standing for 2 h at room temperature. The serum samples were diluted 30-fold times with PBS solution before detection. Different concentrations of lysozyme were added to the serum samples to make the spiked samples. Detection processes were carried out under the optimized conditions. All experiments were performed in compliance with the relevant laws and institutional guidelines and were approved by the Animal Care and Use Committee of Jilin University, and the informed consent was obtained from all human subjects.
Results and discussion
Mechanism of the assay
The sensing strategy for fluorescence detection of lysozyme is demonstrated in Scheme 1. Negatively charged reactive functional group COO− on the surface of MPA-CdS QDs can bind to the positively charged Hb via electrostatic interaction, which results in the fluorescence quenching by the electronic transfer process between MPA-CdS QDs and Hb. After the addition of positively charged lysozyme, the system fluorescent recovered. That is because the lysozyme (pI ≈ 11) has a stronger electrostatic interaction with negatively charged MPA-CdS QDs than Hb (pI ≈ 7.4) does. So lysozyme could replace the Hb, which is already on the surface of MPA-CdS QDs. Thus the fluorescence of the system would be recovered by the addition of lysozyme.
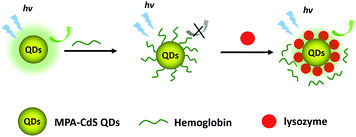 |
| Scheme 1 The schematic representation of the fluorescence detection of lysozyme. | |
Spectral characterization of MPA-CdS QDs
Fig. 1 was the TEM image of the as-prepared CdS QDs. From Fig. 1, it can be seen that the as-prepared CdS QDs were spherical particles with good monodispersity, and the average size was about 6 nm. Fig. 2 was zeta potential of CdS QDs and CdS QDs with hemoglobin. Based on previous reports, solutions with zeta potential above +20 mV and below 20 mV are considered stable.28 As shown in Fig. 2, the zeta potential of MPA capped-CdS QDs is −34.9 mV. After the addition of hemoglobin, the zeta potential of QDs was increased to be −26.5 mV. These results proved the static interaction between positively charged hemoglobin and negatively charged MPA capped-CdS QDs. Fig. 3 showed the UV-Vis absorption and fluorescence emission spectra of MPA-capped CdS QDs. As shown in Fig. 3, there was an increased absorption around 415 nm and a little shoulder around 350 nm, which was the result of 1Sh–1Se excitonic transition characteristic of CdS QDs.29 The fluorescent emission spectra of the MPA-CdS QDs showed a symmetrical and narrow peak at 554 nm. As shown in Fig. 4 (curve b), the majority of MPA functional groups could be clearly found according to the vibrations of –COOH (1580.59 cm−1 asymmetrical stretching vibration, 1407 cm−1 symmetrical stretching vibration) and –OH (3303.99 cm−1 stretching vibration), –CH2 (2911 cm−1 asymmetrical stretching vibration). These characteristic peaks were not observed in the FT-IR spectra of uncapped CdS (Fig. 4 curve a). In addition to it, the intensity of characteristic absorption band of S–H (2500–2680 cm−1) had been dropped, which was because that the S–H here was coordinated to the metal atom on the surface of CdS QDs. These evidences indicated the successful capping of MPA on the surface of the CdS QDs.
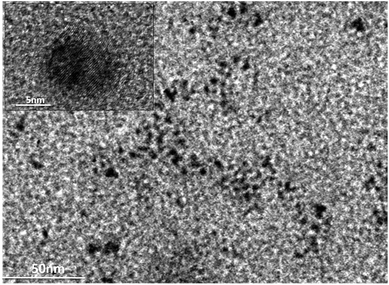 |
| Fig. 1 TEM image of MPA-CdS QDs. | |
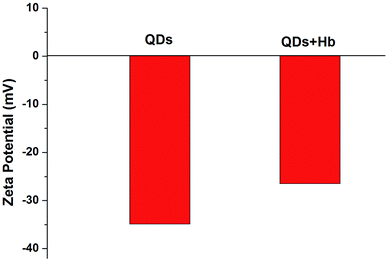 |
| Fig. 2 Zeta potentials of MPA capped-CdS QDs and MPA capped-CdS QDs with hemoglobin. | |
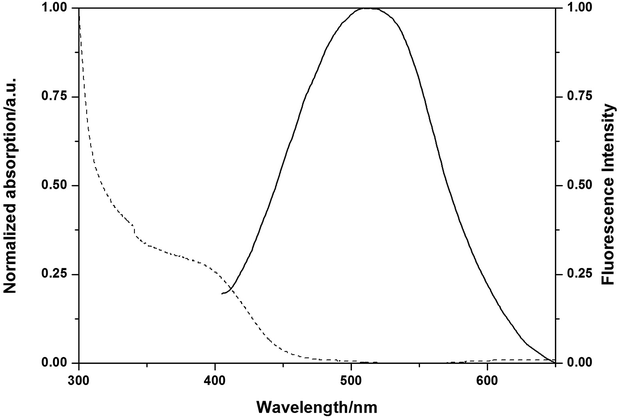 |
| Fig. 3 The UV-Vis absorption (dash line) and fluorescence emission spectra of MPA-capped CdS QDs (solid line). | |
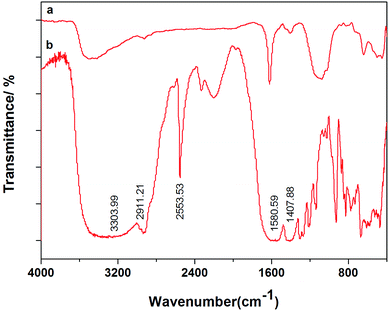 |
| Fig. 4 The FT-IR spectra of the uncapped CdS QDs (curve a) and MPA-capped CdS QDs (curve b). | |
Optimization
First, we studied the effect of pH value on the relative fluorescence intensity of the CdS QDs–Hb (curve a) and CdS QDs–Hb–lysozyme (curve b) system. As shown in Fig. 5, with the increase of the pH value, the quenching ability of Hb on the fluorescence of CdS QDs started to decrease after 6.6, and the ability of fluorescence recovery of lysozyme on the CdS QDs–Hb system reached the maximum at 6.6 and kept relatively stable after that. So we chose pH 6.6 in the future experiments.
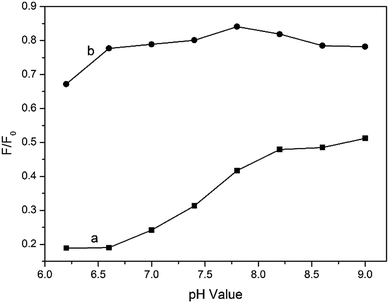 |
| Fig. 5 Relative fluorescence intensity of CdS QDs–Hb system (curve a) and CdS QDs–Hb–lysozyme system (curve b). F/F0: the intensity of CdS QDs in the present and absent of Hb (curve a) and Hb + lysozyme (curve b), respectively. | |
Then we studied the effect of incubation time on the fluorescent intensity of the CdS QDs–Hb and CdS QDs–Hb–lysozyme system. The results showed in Fig. 6(A) demonstrated that the quenching effect of Hb on the CdS QDs was completed within 30 s and slightly weakened afterward. Fig. 6(B) showed that after the addition of lysozyme, the recovery of fluorescence reached maximum at about 2 minutes and then remained constant. So the optimal incubation time for CdS QDs–Hb and CdS QDs–Hb–lysozyme system were set to be 40 seconds and 3 minutes respectively to ensure the complete reaction.
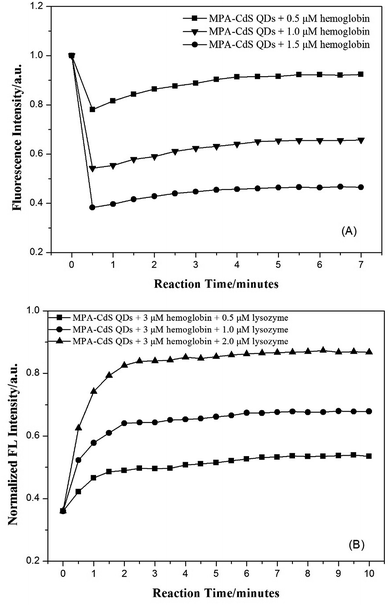 |
| Fig. 6 The effect of incubation time on the fluorescent intensity of the CdS QDs–Hb (A) and CdS QDs–Hb–lysozyme system (B). | |
Reaction between Hb and MPA-CdS QDs
In this work, we studied the quenching effect of Hb on the fluorescence of MPA-CdS QDs. As shown in Fig. 7, after the addition of Hb to the CdS QDs solution the FL intensity of CdS QDs at 554 nm was gradually decreased with the increasing concentration of Hb. Also, a blue shift was observed as a consequence of the change of quantum confinement of the CdS QDs. The inset in Fig. 7 showed that the relative fluorescence intensity F0/F (F0 and F is the intensity of MPA-CdS QDs in the presence and absence of Hb respectively) and concentration of Hb exhibited a good linear relationship in the range of 0.15–7.5 μmol L−1. The regression equation is
F0/F = 1.028 + 0.369[Hb] (μmol L−1) |
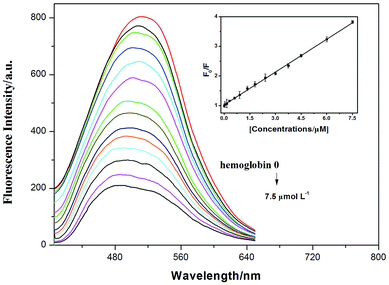 |
| Fig. 7 Fluorescence spectra of MPA-CdS QDs in the presence of different concentration of Hb. The concentration of Hb is 0, 0.045, 0.15, 0.3, 0.6, 0.9, 1.35, 1.8, 2.4, 3, 3.75, 4.5, 6, 7.5 μmol L−1. The inset showed the linear relationship between F0/F and the concentration of Hb. F0 and F is the intensity of MPA-CdS QDs in the presence and absence of Hb respectively. | |
The corresponding regression coefficient (R2) is 0.998. We selected 3.75 μmol L−1 Hb in the following experiments.
Fluorescent detection of lysozyme
When the pH value of the detection system is 6.6, lysozyme (pI ≈ 11) has a stronger electrostatic interaction with negatively charged MPA-CdS QDs than Hb (pI ≈ 7.4) does. So lysozyme could replace the Hb, which is already on the surface of MPA-CdS QDs, thus the fluorescence of the system would be recovered by the addition of lysozyme. As shown in Fig. 8, after the fluorescence of MPA-CdS QDs had been quenched by 3.75 μmol L−1 Hb, the fluorescence intensity of the system was obviously restored with the lysozyme concentration increased from 15 to 600 nmol L−1. Furthermore, the inset in Fig. 8 showed that there was a good linear relationship between the relative fluorescence intensity ratio F/F0 (F and F0 is the intensity of MPA-CdS QDs–Hb system in the presence and absence of lysozyme respectively) and the lysozyme concentration in the range of 15–600 nmol L−1. The regression equation is
F/F0 = 1.006 + 0.001[lysozyme] (nmol L−1). |
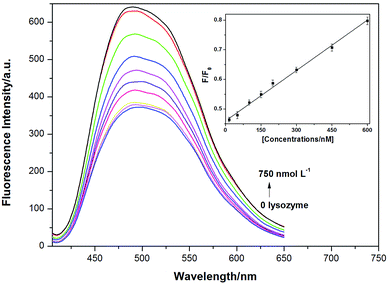 |
| Fig. 8 Fluorescence spectra of MPA-CdS QDs in the presence of 3.75 μmol L−1 Hb and different concentrations of lysozyme. The concentrations of lysozyme are 0, 15, 50, 100, 150, 200, 300, 450, 600, 750 nmol L−1. The inset showed the linear relationship between F/F0 and the concentration of lysozyme. F and F0 is the intensity of MPA-CdS QDs–Hb system in the presence and absence of lysozyme respectively. | |
The corresponding regression coefficient (R2) is 0.999. The detection limit (LOD) is 6.3 nmol L−1. The detection limit is defined by the equation LOD = 3σ/s, where σ is the standard deviation of the corrected blank signals (n = 11) of the ssDNA-QDs/GO and s is the slope of the calibration curve.
A comparison among the proposed method and several reported methods for lysozyme determination in linear range and detection limit was summed up in Table 1.15,16,19,20,30,31 It could be seen from Table 1 that the sensitivity of the method in this work was better than most of the reported methods.
Table 1 Comparison of different methods for the detection of lysozyme
Methods |
Linear range (nmol L−1) |
LOD (nmol L−1) |
Ref. |
Visual detection |
0–120 |
10 |
30 |
Luminescent detection |
2–50 |
2 |
16 |
Electrochemistry |
20–840 |
30 |
15 |
SPR detection |
0–75.08 |
28.53 |
20 |
Colorimetric detection |
50 ng L−1 to 25 μg mL−1 |
23 ng mL−1 |
31 |
Fluorescent detection |
8.9–71.2 |
4.3 |
26 |
ECL detection |
0.5–9 ng mL−1 |
150 ng L−1 |
19 |
Fluorescent detection |
15–600 |
6.3 |
This work |
Interference study
Selectivity is a very important parameter to evaluate the performance of a new analytical method, especially for ones with potential applications in biomedical samples. Thus a highly selective response to the target over other potentially competing species is necessary. Therefore, we further evaluated the selectivity of our method with various coexistence protein added.
Under optimal conditions, the selectivity of the developed method for lysozyme detection was studied by using 3.75 μmol L−1 Hb, 0.8 μmol L−1 lysozyme and 8 μmol L−1 other common proteins, including BSA, HAS, HRP, Urase, ALP, trypsin and pepsin. As shown in Fig. 9, only lysozyme could effectively restore the fluorescence of the system, and other proteins had little influence on the fluorescence intensity compared to the blank test (in the presence of Hb and absence of other proteins). The results showed that the presented method could provide good ability in resisting interference for lysozyme detection.
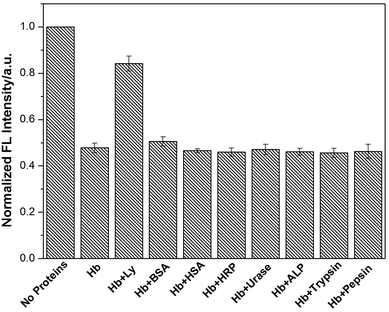 |
| Fig. 9 The fluorescent intensity of CdS QDS in the presence of Hb (3.75 μmol L−1), lysozyme (0.8 μmol L−1) and 8 μmol L−1 other proteins, including BSA, HSA, HRP, Urase, ALP, trypsin and pepsin. | |
Real samples detection
To demonstrate the potential application of the proposed method for lysozyme analysis in biological media, the assay of lysozyme in human serum was performed. Specifically, different concentrations of lysozyme were added into the 30-fold diluted serum, and the results obtained by the standard addition method were showed in Table 2. The recovery of added lysozyme in the spiked serum samples ranged from 92% to 106%, and the relative standard deviations were no more than 1.42%. The above results suggested that the proposed approach had potential bio-medical application since the abnormal lysozyme level in serum indicates some diseases.
Table 2 Determination of lysozyme in serum samples
Samples |
Original found (nmol L−1) |
Added (nmol L−1) |
Total found (nmol L−1) |
Recovery (%) |
RSD (%, n = 3) |
1 |
203.1 |
200.0 |
398.8 |
98.9 |
0.96 |
2 |
233.6 |
230.0 |
461.6 |
99.6 |
1.24 |
3 |
253.4 |
250.0 |
507.4 |
100.8 |
1.42 |
Conclusion
In summary, a novel biosensor based on the fluorescent “turn off–on” of 3-mercaptopropionic acid-capped CdS quantum dots (MPA-CdS QDs) was designed for the detection of lysozyme. The fluorescence of CdS QDs can be quenched by Hb due to the electronic transferring, and then recovered by lysozyme via the replacement of Hb. Compared with most of the literatures about lysozyme assay, the method we presented was cost-effective, convenient, and did not require any complicated synthetic procedures.
Acknowledgements
This work was financially supported by the National Natural Science Foundation of China (No. 21075050 and 21275063) and the Science and Technology Development project of Jilin province, China (No. 20150204010GX).
References
- D. Liao, J. Chen, W. Li, Q. Zhang, F. Wang, Y. Li and C. Yu, Chem. Commun., 2013, 49, 9458–9460 RSC
. - B. Wang and C. Yu, Angew. Chem., Int. Ed., 2010, 49, 1485–1488 CrossRef CAS PubMed
. - S. Liu, W. Na, S. Pang, F. Shi and X. Su, Talanta, 2014, 139, 3048–3054 CAS
. - C. Q. Jiang and L. Luo, Anal. Chim. Acta, 2004, 511, 11–16 CrossRef CAS
. - K. C. Lin, M. T. Wey, L. S. Kan and D. Shiuan, Appl. Biochem. Biotechnol., 2008, 158, 631–641 CrossRef PubMed
. - J. Li, X.-W. He, Y.-L. Wu, W.-Y. Li and Y.-K. Zhang, Anal. Sci., 2007, 23, 331–335 CrossRef PubMed
. - S. S. Levinson, R. J. Elin and L. Yam, Clin. Chem., 2002, 48, 1131–1132 CAS
. - O. S. Elkhatib, O. Lebwohl, A. A. Attia, C. A. Flood, J. A. Stein, J. G. Sweeting, R. T. Whitlock, E. F. Osserman and P. R. Holt, Am. J. Dig. Dis., 1978, 23, 297–301 CrossRef CAS
. - K. Shima, M. Hirota, M. Fukuda and A. Tanaka, Clin. Chem., 1986, 32, 1818–1822 CAS
. - Y. Lian, F. He, X. Mi, F. Tong and X. Shi, Sens. Actuators, B, 2014, 199, 377–383 CrossRef CAS
. - S. Li, J. J. Mulloor, L. Wang, Y. Ji, C. J. Mulloor, M. Micic, J. Orbulescu and R. M. Leblanc, ACS Appl. Mater. Interfaces, 2014, 6, 5704–5712 CAS
. - Z. L. Jiang and G. X. Huang, Clin. Chim. Acta, 2007, 376, 136–141 CrossRef CAS PubMed
. - H. Zheng, S. Qiu, K. Xu, L. Luo, Y. Song, Z. Lin, L. Guo, B. Qiu and G. Chen, Analyst, 2013, 138, 6517–6522 RSC
. - A. Arabzadeh and A. Salimi, Biosens. Bioelectron., 2015, 74, 270–276 CrossRef CAS PubMed
. - B. M. B. Felisilda, E. A. de Eulate and D. W. M. Arrigan, Anal. Chim. Acta, 2015, 893, 34–40 CrossRef CAS PubMed
. - L. Lu, W. Wang, M. Wang, T.-S. Kang, J.-J. Lu, X.-P. Chen, Q.-B. Han, C.-H. Leung and D.-L. Ma, J. Mater. Chem. B, 2016, 4, 2407–2411 RSC
. - Y.-M. Chen, C.-J. Yu, T.-L. Cheng and W.-L. Tseng, Langmuir, 2008, 24, 3654–3660 CrossRef CAS PubMed
. - Y. Song, C. Xu, W. Wei, J. Ren and X. Qu, Chem. Commun., 2011, 47, 9083–9085 RSC
. - H. Wang, W. Gong, Z. Tan, X. Yin and L. Wang, Electrochim. Acta, 2012, 76, 416–423 CrossRef CAS
. - A. Erdem, E. Eksin and M. Muti, Colloids Surf., B, 2014, 115, 205–211 CrossRef CAS PubMed
. - J. J. Hill and C. A. Royer, Methods Enzymol., 1997, 278, 390–416 CAS
. - J. Wang, L. Gao, D. Han, J. Pan, H. Qiu, H. Li, X. Wei, J. Dai, J. Yang and H. Yao, J. Agric. Food Chem., 2015, 63, 2392–2399 CrossRef CAS PubMed
. - H. Sunayama, T. Ooya and T. Takeuchi, Biosens. Bioelectron., 2010, 26, 458–462 CrossRef CAS PubMed
. - J. K. Jaiswal and S. M. Simon, Trends Cell Biol., 2004, 14, 497–504 CrossRef CAS PubMed
. - M. A. Ali, S. Srivastava, M. K. Pandey, V. V. Agrawal, R. John and B. D. Malhotra, Anal. Chem., 2014, 86, 1710–1718 CrossRef CAS PubMed
. - S. Li, Z. Gao and N. Shao, Talanta, 2014, 129, 86–92 CrossRef CAS PubMed
. - P. Wu, T. Zhao, Y. Tian, L. Wu and X. Hou, Chem.–Eur. J., 2013, 19, 7473–7479 CrossRef CAS PubMed
. - T. C. Prathna, N. Chandrasekaran and A. Mukherjee, Colloids Surf., A, 2011, 390, 216–224 CrossRef CAS
. - H. Matsumoto, T. Sakata, H. Mori and H. Yoneyama, J. Phys. Chem., 1996, 100, 13781–13785 CrossRef CAS
. - T. T. Moghadam and B. Ranjbar, Talanta, 2015, 144, 778–787 CrossRef PubMed
. - H. Zheng, S. Qiu, K. Xu, L. Luo, Y. Song, Z. Lin, L. Guo, B. Qiu and G. Chen, Analyst, 2013, 138, 6517–6522 RSC
.
|
This journal is © The Royal Society of Chemistry 2016 |
Click here to see how this site uses Cookies. View our privacy policy here.