DOI:
10.1039/C6RA17214H
(Paper)
RSC Adv., 2016,
6, 98945-98951
Preparation and characterization of sodium polyacrylate-grafted bentonite and its performance removing Pb2+ from aqueous solutions
Received
5th July 2016
, Accepted 7th October 2016
First published on 7th October 2016
Abstract
A new adsorbent, sodium polyacrylate (PAANa) grafted onto the surface of bentonite (Bent), was successfully synthesized by a facile and green Ce(IV)-redox process, for the removal of Pb2+ from aqueous solutions. The results indicate that by this method the original layered structure of the clay can be protected along with its cation exchange capability (CEC), leading to an improved adsorption capacity. The adsorbent (Bent–PAANa) was characterized by scanning electron microscopy (SEM), Fourier transform infrared spectrometry (FTIR), X-ray diffraction (XRD), thermogravimetric analysis (TGA) and N2 adsorption–desorption isotherm studies. Subsequently the effects of pH, initial concentration and contact time on the adsorption of Pb2+ from aqueous solutions were investigated. The results of the batch experiments specifically indicate that a high adsorption capacity (149.62 mg g−1) of Bent–PAANa for the removal of Pb2+ was obtained at pH = 5, C0 = 1100 mg L−1 and 25 °C. Furthermore, the adsorption kinetics of Pb2+ onto the composite followed a pseudo-second-order kinetic model. The adsorption data fitted better to a Freundlich adsorption isotherm than a Langmuir adsorption isotherm hence confirming multilayer adsorption.
1. Introduction
Currently, heavy metals are widely used in many industrial applications, including textiles, printing, plastics, food, leather and papermaking. Large amounts of waste water polluted by these heavy metals are directly discharged into natural water without decontamination in many places. The wide-spread occurrence of heavy metal pollution is one of the major problems in the environment, because the associated toxicity and carcinogenicity is a big threat to humans and animals.1–3 Unlike organic pollutants, toxic heavy metals are non-biodegradable, and even worse they accumulate in living organisms through the food cycle.4,5 Generally, an excess of heavy metal ions in living organisms causes a lot of damage, such as dysfunction of the kidneys, liver, brain, central nervous system, and so on.6 Therefore, the removal of heavy-metal ions from industrial wastewater is necessary before discharging into natural water to avoid water pollution.
At present, several conventional techniques are used for removing heavy metals from aqueous solutions, such as chemical precipitation, ion exchange, solvent extraction, reverse osmosis and adsorption.7–11 Among these methods, adsorption is attractive because it is a highly efficient and easy to implement process in the treatment of waste water.12–14 This is because the adsorption process can be achieved at ambient pressure and temperature and without the use of any expensive hydrogen as well as a catalyst.15
Conventional adsorbents such as active carbon, zeolite and chitosan, etc., have been used to adsorb dyes and heavy metals in waste water. However, these traditional adsorbents are severely limited in their application, because their prices are relatively expensive and the preparation processes are more complex.16–20 From this point of view, it is necessary to research and develop cheaper and more effective adsorbents as substitutes for the normal adsorbents.
Bent is a good adsorbent for heavy metals from aqueous solutions because it has many advantages, such as high cation-exchange capacities, nontoxicity, low cost and the ability to be recycled.21–24 And also, on the crystal edges of the aluminate sheets, there are a large amount of terminal –OH groups providing enough anchoring sites for modification.25 In previous studies, clay has been mostly modified by intercalation polymerization.26–28 However, the polymerization method always destroys the layered structure of the clay and then reduces the cation exchange capacity. In this paper, the polymer is grafted onto the surface of Bent without destroying its layered structure. At the same time, keeping the cation exchange capability of Bent, the adsorption capacity of the modified Bent to remove Pb2+ is highly improved. In recent years, clay/polymer composites have been reported to have enhanced adsorptivity. In our previous work, He et al.29 prepared a PAANa/Bent complex by in situ polymerization for the removal of Pb2+. However, the maximum adsorption capacity was only about 70 mg g−1 and the synthesis method was extremely complex. Consequently, compared to other methods,30–32 the polymerization method for Bent–PAANa can be completed by a simple method to get the carboxyl functionalized Bent at low temperatures.
2. Materials and method
2.1. Materials
Bentonite (Bent, Fenghong, China) was acid-activated by 1 M HNO3 at 60 °C for 24 h before used. The monomer sodium acrylate was synthesized by mixing acrylic acid (AA, 98%, Aladdin) and sodium hydroxide (NaOH, 98%, Aladdin). Ceric ammonium nitrate (CAN, 99%, Aladdin) was used as an initiator. Deionized water was used to prepare all test solutions as needed.
2.2. Synthesis of clay-based composite (Bent–PAANa)
A schematic illustration of the preparation route is shown in Scheme 1. Bent (3.0 g) was added to 100 mL water in a round-bottom flask having a volume of 250 mL. The Bent was completely dissolved in water by mixing with a magnetic stirrer, and after it was well-dispersed, 20 mL of aqueous solution of the monomer sodium acrylate (1.5 g mL−1) was introduced into the mixture and stirred for 30 min at 30 °C under a nitrogen atmosphere. Ce(NH4)2(NO3)6 (0.025 g mL−1, 20 mL) was added dropwise under a nitrogen atmosphere, and the dispersion was heated at 30 °C for 2 h. After the polymerization, the mixture was cooled to 0 °C in an ice bath. The Bent–PAANa was filtered and washed with copious amounts of water and then dried at 60 °C in a vacuum oven.
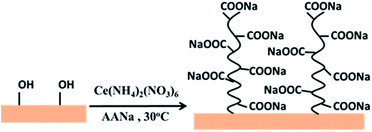 |
| Scheme 1 Cerium (IV) ion initiated polymerization of sodium acrylate on the surface of Bent. | |
2.3. Characterization
XRD patterns were recorded on a Bruker AXS D8 Focus X-ray diffractometer operating at 40 kV and 40 mA. The scan angle 2θ varied from 2° to 40° and the scan speed was 0.02° s−1. FTIR spectra were recorded on a Bruker VECTOR-22 and the spectra were collected over the spectral range of 400–4000 cm−1. TGA was conducted on a Perkin-Elmer Diamond TG/DTA instrument with a heating rate of 10 °C min−1 under a flow of nitrogen at temperatures ranging from 25 to 900 °C. The morphology of Bent and Bent–PAANa was examined by SEM (JSM-6700F, JEOL Ltd.). Nitrogen adsorption–desorption isotherms were measured at −196 °C with a Tristar II 3020 (Micromeritics Inc., USA). The bentonite sample was degassed overnight at 150 °C prior to the adsorption measurements. The BET specific surface areas were calculated from the adsorption data in the relative pressure (P/P0). The pore size and pore volume were calculated using the BJH method applied to the desorption leg of the isotherms.
2.4. Pb2+ solutions
The stock solution of Pb2+ used here was obtained by dissolving an accurate quantity of Pb(NO3)2 in deionized water. Other concentrations were prepared from this stock solution by dilution. The solution pH was adjusted with 0.1 M HNO3 or 0.1 M NaOH. Fresh dilutions were used for each experiment.
2.5. Batch adsorption studies
All the adsorption experiments were conducted 3 times and the averaged values were used as the experimental data to make the results reliable. What’s more, all the batch experiments were conducted in a temperature controlled water bath shaker (Dong Peng SHA-C) at 230 rpm and 25 °C. The effects of initial concentration, pH and time were studied in the adsorption experiments. Firstly, 0.05 g of Bent–PAANa and 40 mL of Pb2+ solution were added to a 50 mL conical flask. After a predetermined time, the solid and the liquid phases were separated by centrifugation at 8000 rpm for 3 min. The supernatant solutions were analyzed using an atomic absorption spectrophotometer (AAS). The adsorption amount was calculated using absorbance values measured before and after adsorption according to the following equation: |
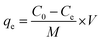 | (1) |
where qe is the amount of Pb2+ adsorbed by the adsorbent (mg g−1), C0 is the initial Pb2+ concentration (mg L−1), Ce is the Pb2+ concentration after adsorption (mg L−1), M is the mass of the Bent–PAANa adsorbent (g), and V is the volume of the Pb2+ solution (L).
3. Results and discussion
3.1. Characterization of Bent–PAANa
The XRD patterns of Bent and Bent–PAANa are shown in Fig. 1. The structure of the composite can be determined using XRD techniques from the positions, shape and intensity of the basal reflections in the XRD pattern. The acute diffraction peak at 2θ = 6.68° in Bent is the (001) reflection, which indicates the existence of a layered structure. The basal spacing for Bent is 1.32 nm. After modification with PAANa the 2θ changes from 6.68° to 6.22° and the basal spacing for Bent–PAANa is 1.42 nm. Nevertheless, there is no significant change in the basal spacing. This indicates that the polymer brushes are mostly grafted on the surface of the clay mineral.
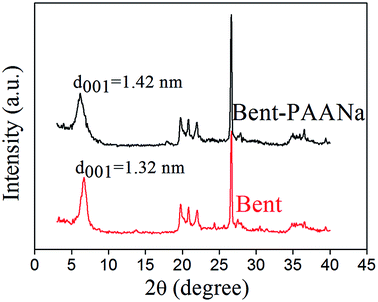 |
| Fig. 1 The XRD patterns of Bent and Bent–PAANa. | |
Fig. 2 shows the typical FTIR spectra of Bent and the Bent–PAANa composite. The band at 3614 cm−1 is a stretching vibration of the hydroxyl bound to an aluminum (Al(Al)OH) or magnesium (Al(Mg)OH) of the clay mineral. The band at 3449 cm−1 is ascribed to a stretching of the hydroxyl group of the adsorbed water molecules.33 The strong band at 1033 cm−1 represents the Si–O–Si groups of the tetrahedral sheets, while the bands at 797, 624, and 521 cm−1 are due to the deformation and bending modes of the Si–O bond.34 The strong band at 1024 cm−1 is attributed to the Si–O–Si groups of the tetrahedral sheets. The band at 1638 cm−1 is due to the bending vibrations of the H–O–H bonds of the water molecules intercalated in the clay mineral. It is observed that the bands of the spectrum of PAANa at 1565 and 1455 cm−1 are indicative of COO− asymmetric and symmetric stretching, respectively. In the spectrum of Bent–PAANa, the asymmetric CH2 stretch and the symmetric CH2 have shifted to 2922 and 2852 cm−1, respectively.33 And there appears to be two new bands at 1439 and 1545 cm−1, which are characteristic of the asymmetric νas (COO−) and the symmetric νs (COO−) stretch, instead.35,36 As can be seen from Fig. 2, the spectrum of Bent–PAANa coincides with that of PAANa.
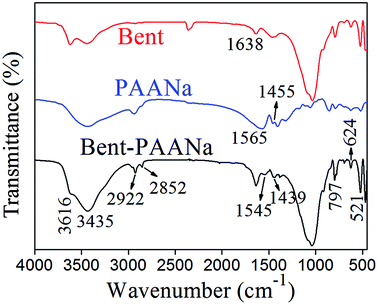 |
| Fig. 2 FTIR spectra of Bent, PAANa and the Bent–PAANa composite. | |
The TG curves of Bent and the Bent–PAANa composite are illustrated in Fig. 3. Bent undergoes two stages of thermal degradation. The TG curve exhibits an initial dry weight loss of 4.0% in the range of 40–200 °C. Firstly, weight loss from 40 to 110 °C could be attributed to desorption of physically adsorbed water on the surface layer, and the other mass loss from 110 to 200 °C could be attributed to the loss of structural water located in the interlayer. In the second stage, almost 3.0% mass loss occurs in the temperature range of 600–700 °C due to the dehydroxylation of the aluminosilicate layer. Three stages of thermal decomposition take place in the case of the Bent–PAANa composite. The first weight loss stage of 4% from 40 to 150 °C is due to the loss of water molecules. The second weight loss stage of 6% from 200 to 630 °C is attributed to the decomposition of the polymer matrix and the third one of 1% from 600 to 700 °C is because of the dehydroxylation of the aluminosilicate layer.
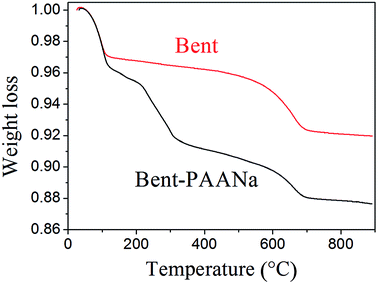 |
| Fig. 3 The TGA curves of Bent and the Bent–PAANa composite. | |
SEM observations were used to characterize the surface, shape and porosity of the adsorbent. As can be seen from Fig. 4a, Bent appears as closely packed flakes, which is consistent with descriptions in a previous study.37 These results indicate that the majority of smectite crystals have a layered structure. As is shown clearly in Fig. 4b, the surface of Bent–PAANa becomes smoother and is evidently wrapped up. To sum up, the change of morphology of bentonite supports the occurrence of surface graft polymerization.
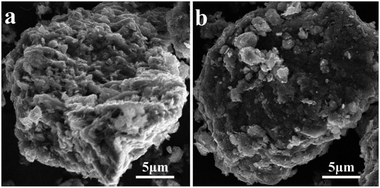 |
| Fig. 4 SEM images of Bent (a) and Bent–PAANa (b). | |
Fig. 5 shows the N2 adsorption–desorption isotherms of Bent and Bent–PAANa. In Fig. 5, the data represent type IV adsorption isotherms with type H3 hysteresis loops which are characteristic of slit-like pore materials. The textural properties of the two samples are presented in Table 1. It is apparent that the values for these main properties of Bent, including SBET and pore volume, are larger than those of Bent–PAANa. The results reveal that the surface of the clay is covered.38 In other words, the sodium polyacrylate has been grafted onto the surface of bentonite successfully. Although the specific surface area of the clay decreases, the grafting of sodium polyacrylate creates more adsorption sites to increase the adsorption capacity.
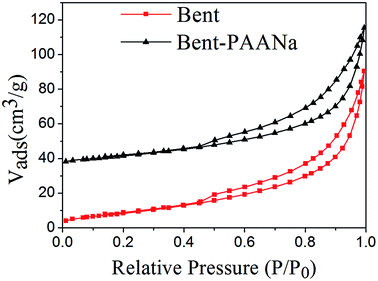 |
| Fig. 5 N2 adsorption–desorption isotherms of Bent and Bent–PAANa. | |
Table 1 Textural properties of Bent and Bent–PAANa
Sample |
SBET (m2 g−1) |
Pore volume (cm3 g−1) |
Average pore diameter (nm) |
Bent |
33.6326 |
0.140163 |
16.66987 |
Bent–PAANa |
24.6262 |
0.115562 |
19.35801 |
3.2. Adsorption studies
3.2.1. The effect of contact time and kinetic study. The effect of contact time on the removal efficiency of Pb2+ was studied at pH = 5, 298 K, with 50 mg adsorbent and 40 mL Pb2+ solution (600 mg L−1). The effect of time on the adsorption properties of Bent and Bent–PAANa are shown in Fig. 6. It can be noted from Fig. 6 that the equilibrium amount of Pb2+ adsorbed by Bent is observed in the first 4 h. In contrast, the adsorption process of Bent–PAANa is rapid during the first 2 h of contact time and then the adsorption capacity increases slowly and reaches equilibrium within 10 h.
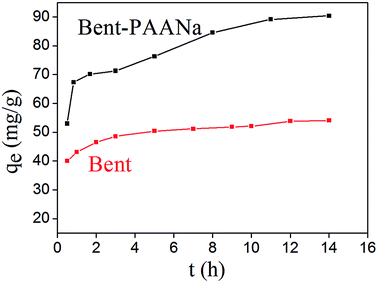 |
| Fig. 6 The effect of time on the adsorption of Pb2+ onto Bent and Bent–PAANa (C0 = 600 mg L−1; pH = 5). | |
The kinetics of adsorption is one of the most attractive characterizations that is responsible for the efficiency of adsorption. Two kinetic models, pseudo-first-order and pseudo-second-order kinetic models, were used to analyze the best fitted model for the experimental data. The pseudo-first-order kinetic model can be expressed as:
|
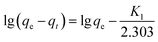 | (2) |
qt (mg g−1) is the amount of Pb2+ absorbed at time t (h), qe is the amount of adsorbed Pb2+ at equilibrium (mg g−1), and K1 (h−1) is the equilibrium rate constant of the pseudo-first-order kinetic model.
The pseudo-second order process is written as follows:
|
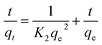 | (3) |
K2 is the equilibrium rate constant of the pseudo-second-order model. The plots of the pseudo-first-order and pseudo-second-order models are shown in Fig. 7 and their parameters are listed in Table 2. The correlation coefficients (R2) for the pseudo-second-order kinetic model is higher in comparison with the values obtained for the pseudo-first-order kinetic model. Therefore, it is reasonable to conclude that the pseudo-second-order model is the obeyed model.
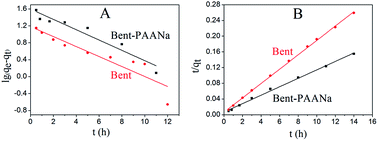 |
| Fig. 7 Pseudo-first order kinetic model (A) and pseudo-second order kinetic model (B) fitting for the adsorption of Pb2+ on Bent and Bent–PAANa. | |
Table 2 Calculated kinetic model constants
Adsorbent |
First order kinetic model |
Second order kinetic model |
K1 (h−1) |
R2 |
K2 (h−1) |
R2 |
Bent |
0.11801 |
0.83024 |
0.05397 |
0.99913 |
Bent–PAANa |
0.12193 |
0.91658 |
0.01835 |
0.99626 |
3.2.2. The effect of pH. As a general rule, the pH of the aqueous solution is the dominant parameter in the adsorption process of Pb2+. To avoid precipitation of Pb2+, all the experiments were conducted under slightly acidic conditions, where the adsorption process was performed at pH = 2.0 to 5.0. The results depicted in Fig. 8 show that, indeed, adsorption capacity gradually increases with an increase in the pH. This is attributable to the competition between H+ and Pb2+. Under low pH conditions, the adsorption capacity of Bent–PAANa becomes lower due to H+ competing with Pb2+ for absorption sites on the adsorbent.39 However, under higher pH conditions, the carboxyl (COOH) groups of the adsorbent will ionize and convert to carboxylate (COO−) groups with negative charges, that will then capture the target metal ions (positively charged Pb2+) by electrostatic forces of attraction.40–42
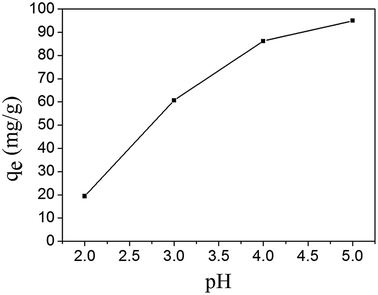 |
| Fig. 8 The effect of pH on the adsorption of Pb2+ onto Bent–PAANa (C0 = 600 mg L−1; t = 10 h). | |
3.2.3. The effect of the initial metal concentration and isotherm study. In batch experiments, the initial concentration of Pb2+ in the solution played an important role as a driving force to overcome the mass transfer resistance between the aqueous and solid phases.43 Experiments to explore the effect of the various initial Pb2+ concentrations were carried out by using 1.25 g L−1 of Bent, at 25 °C and pH = 5. As can be seen from Fig. 9, the adsorption capacity (qe) evidently increases with an increase in the initial Pb2+ concentration and becomes almost smooth after 800 mg L−1 until the equilibrium state. In a certain concentration range, when the Pb2+ concentration become higher, the adsorption of Pb2+ on Bent–PAANa will also be improved. However, when adsorption saturation is reached, the amount of adsorbed Pb2+ will no longer be increased. To determine the surface properties and the affinity of the adsorbent, the experimental data were analyzed using the Langmuir and Freundlich isotherm models. Fig. 10 shows the adsorption isotherms. In addition, Table 3 shows the parameters being calculated according to the Langmuir and Freundlich models.
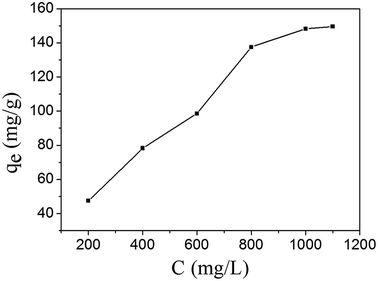 |
| Fig. 9 The effect of initial concentration and contact time on the adsorption of Pb2+ on Bent–PAANa (t = 10 h; pH = 5). | |
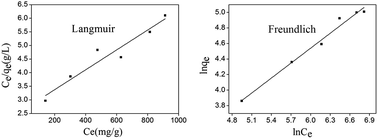 |
| Fig. 10 Langmuir and Freundlich adsorption isotherms for Pb2+ on Bent–PAANa (C0 from 200 to 1200 mg L−1; pH = 5; t = 10 h). | |
Table 3 The isotherm parameters for the adsorption of Pb2+ by Bent–PAANa
Langmuir |
Freundlich |
KL (L mg−1) |
qm (mg L−1) |
R2 |
KF (mg g−1) (L mg−1)−1/n |
n (L mg−1) |
R2 |
0.00138 |
273.97 |
0.91678 |
2.00168 |
1.56021 |
0.98014 |
The Langmuir isotherm theory is based on the assumption of monolayer adsorption without interactions between the adsorbed molecules.44 The Langmuir equation can be expressed as:
|
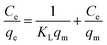 | (4) |
where
KL is the Langmuir binding constant,
Ce is the equilibrium concentration of the Pb
2+ solution (mg L
−1),
qe is the adsorbed value of Pb
2+ at the equilibrium concentration (mg g
−1) and
qm is the maximum adsorption amount (mg g
−1).
The Freundlich model is based on the assumption of adsorption on a heterogeneous surface.45 The equation can be expressed as:
|
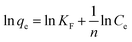 | (5) |
qe is the adsorbed value of Pb2+ at the equilibrium concentration (mg g−1). Ce is the equilibrium concentration of the Pb2+ solution (mg L−1). KF (L g−1) and n are Freundlich constants related to the capacity and intensity of adsorption, respectively. Comparing the correlation coefficients of the analyzed isotherms, the high values for the correlation coefficient (R2) indicated that the adsorption process can be better described by the Freundlich model.
3.2.4. Adsorption mechanism. Under the same adsorption conditions, the maximum adsorption capacity of Pb2+ onto the Bent–PAANa composite is 149.62 mg g−1. The high adsorption capacity could be explained as follows. Pb2+ adsorption onto the clay proceeds in three ways. The first one is that retention of heavy-metal ions on Bent mainly occurs via cation exchange46 which can be represented as: |
Bent Mn+ + Pb2+ → Bent Pb2+ + Mn+, [Mn+ = Na+, K+, Ca2+, Mg2+, etc.]
| (6) |
The second one is that Pb2+ can be adsorbed by the silanol groups (Si–OH) as well.47 This process can be expressed as:
|
Si–OH + Pb2+ → Si–OPb+ + H+
| (7) |
|
2Si–OH + Pb2+ → (Si–O)2Pb + 2H+
| (8) |
The third one is that Pb2+ can also be adsorbed by complex-ion formation with carboxylic ions (COO−). This process can be expressed as:
|
2(R–COONa+) + Pb2+ → (R–COO)2Pb2+ + 2Na+
| (9) |
According to eqn (6)–(9), it can be seen that Bent–PAANa has multiple metal binding sites that were closely associated with Pb2+. This is due to the grafting of sodium polyacrylate with carboxyl groups that can complex with Pb2+. Therefore, the adsorbent’s ability for adsorption is outstanding.
3.3. Regeneration
It is well known that the reuse of adsorbents is an important process in adsorption studies. The reusability of Bent–PAANa was tested over five cycles, with the same adsorbent washed with 0.1 M HCl solution first and then with deionized water to neutral-pH. Finally the washed adsorbent was dried at 60 °C and used for the next adsorption cycle.
Fig. 11 shows the reusability of Bent–PAANa with the same initial concentration of Pb2+ (600 mg L−1). As can be seen, after several consecutive cycles the adsorption capacity was only slightly decreased when compared with Bent–PAANa when used for the first time (98.6 mg g−1). The second adsorption capacity was 85.4 mg g−1 and then the adsorption capacity was almost unchanged. This may be attributed to residual Pb2+ ions occupying adsorption sites after the desorption process.48 In general, Bent–PAANa materials have potential to be applied as a promising adsorbent for the removal of heavy metals from aqueous solutions and industry waste water.
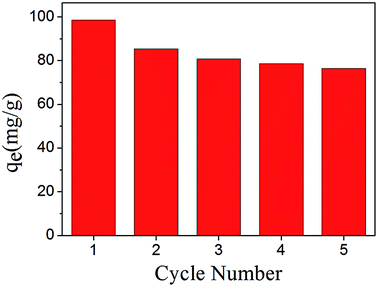 |
| Fig. 11 The reusability of Bent–PAANa for Pb2+ adsorption (C0 = 600 mg L−1; T = 298 K; pH = 5; t = 10 h). | |
4. Conclusions
In this paper, the novel adsorbent Bent–PAANa was synthesized successfully by a Ce(IV)-redox process. The experimental process can be simply operated and the reaction temperature is close to room temperature. These characteristics, such as simple operation, energy-saving, low-cost and so on, hold an important advantage in practical application on a large scale. The sodium polyacrylate anchored on the surface of bentonite is different from those clay/polymer composites from traditional intercalation polymerization processes. Thus the layered structure of bentonite was well protected to improve adsorption capacity and the obtained maximum adsorption capacity is 149.62 mg g−1 (pH = 5, C0 = 1100 mg L−1 and 25 °C). The adsorption capacity in this paper is about 2 times larger than that reported in the literature before.28 Meanwhile, Pb2+ adsorption onto the composite follows the pseudo-second-order kinetic model and the adsorption isotherm data is better described by the Freundlich isotherm. In addition, the Bent–PAANa adsorbent possessed excellent reusability. To summarise, the modified bentonite has great application prospects for the removal of pollutants from the environment.
References
- G. Flora, D. Gupta and A. Tiwari, Interdiscip. Toxicol., 2012, 5, 47–58 CrossRef CAS PubMed.
- M. I. Khan, N. Islam, A. A. Sahasrabuddhe, A. A. Mahdi, H. Siddiqui, M. Ashquin and I. Ahmad, J. Hazard. Mater., 2011, 189, 255–264 CrossRef CAS PubMed.
- L. Yuan, W. Zhi, Y. Liu, S. Karyala, P. J. Vikesland, X. Chen and H. Zhang, Environ. Sci. Technol., 2015, 49, 824–830 CrossRef CAS PubMed.
- Y. J. An, Y. M. Kim, T. I. Kwon and S. W. Jeong, Sci. Total Environ., 2004, 326, 85–93 CrossRef CAS PubMed.
- C. A. Oliveira Ribeiro, Y. Vollaire, A. Sanchez-Chardi and H. Roche, Aquat. Toxicol., 2005, 74, 53 CrossRef PubMed.
- W. H. Lo, H. Chua, K. H. Lam and S. H. Bi, Chemosphere, 1999, 39, 2723 CrossRef CAS PubMed.
- O. D. Uluozlu, M. Tuzen, D. Mendil and M. Soylak, J. Hazard. Mater., 2010, 176, 1032–1037 CrossRef CAS PubMed.
- I. Koyuncu, N. Akcin, G. Akcin and K. F. Mutlu, Rev. Anal. Chem., 2010, 29, 93–106 CAS.
- A. B. Resterna, R. Cierpiszewski and K. Prochaska, J. Hazard. Mater., 2010, 179, 828–833 CrossRef PubMed.
- D. I. Stewart, I. T. Burke, D. V. Hughes-Berry and R. A. Whittleston, Ecol. Eng., 2010, 36, 211–221 CrossRef.
- F. Fu and Q. Wang, J. Environ. Manage., 2011, 92, 407 CrossRef CAS PubMed.
- Lalhmunsiama, S. M. Lee and D. Tiwari, Chem. Eng. J., 2013, 225, 128–137 CrossRef CAS.
- F. N. Azad, M. Ghaedi, K. Dashtian, M. Montazerozohori, S. Hajati and E. Alipanahpour, RSC Adv., 2015, 5(75), 61060–61069 RSC.
- S. Babel and T. A. Kurniawan, J. Hazard. Mater., 2003, 97, 219 CrossRef CAS PubMed.
- M. Ishaq, S. Sultan, I. Ahmad, H. Ullah, M. Yaseen and A. Amir, J. Saudi Chem. Soc., 2015 DOI:10.1016/j.jscs.2015.02.003.
- M. Rafatullah, O. Sulaiman, R. Hashim and A. Ahmad, J. Hazard. Mater., 2010, 177, 70–80 CrossRef CAS PubMed.
- P. Pengthamkeerati, T. Satapanajaru and O. Singchan, J. Hazard. Mater., 2008, 153, 1149–1156 CrossRef CAS PubMed.
- E. Hoseinzadeh, M. Samarghandi, G. McKay, N. Rahimi and J. Jafari, Desalin. Water Treat., 2014, 52, 4999–5006 CrossRef CAS.
- S. G. Ghugal, S. S. Umare and R. Sasikala, RSC Adv., 2015, 5, 63393–63400 RSC.
- D. Mahanta, G. Madras, S. Radhakrishnan and S. Patil, J. Phys. Chem. B, 2008, 112, 10153–10157 CrossRef CAS PubMed.
- A. S. Bhatt, P. L. Sakaria, M. Vasudevan, R. R. Pawar, N. Sudheesh, H. C. Bajaj and H. M. Mody, RSC Adv., 2012, 2, 8663 RSC.
- H. A. Patel, G. V. Joshi, R. R. Pawar, H. C. Bajaj and R. V. Jasra, Polym. Compos., 2010, 31, 399–404 CAS.
- Y. H. Dong, S. W. Wang, M. L. He, L. Chen and X. J. Yu, Appl. Clay Sci., 2009, 43, 164–171 CrossRef.
- P. X. Wu, Z. W. Liao, H. F. Zhang and J. G. Guo, Environ. Int., 2010, 26, 401–407 CrossRef.
- S. Ng and J. Plank, Cem. Concr. Res., 2012, 42, 847–854 CrossRef CAS.
- Z. H. Qin, P. Yuan, S. Q. Yang, D. Liu, H. P. He and J. X. Zhu, Appl. Clay Sci., 2014, 99, 229–236 CrossRef CAS.
- M. R. Jalil, M. Baschini, E. R. Castellon, A. I. Molina and K. Sapag, Appl. Clay Sci., 2014, 87, 245–253 CrossRef.
- Q. Li, Q. Y. Yue, H. J. Sun, Y. Su and B. Y. Gao, J. Environ. Manage., 2010, 91, 1601–1611 CrossRef CAS PubMed.
- Y. J. He, M. S. Pei, N. Xue, L. Y. Wang and W. J. Guo, RSC Adv., 2016, 6, 48145–48154 RSC.
- K. C. Gupta and K. Khandekar, Biomacromolecules, 2011, 4, 758–765 CrossRef PubMed.
- A. Zaharia, A. Sarbu, A. L. Radu, K. Jankova, A. Daugaard, S. Hvilsted, F. X. Perrin, M. Teodorescu, C. Munteanu and V. Fruth-Oprisan, Appl. Clay Sci., 2015, 103, 46–54 CrossRef CAS.
- K. C. Gupta, S. Sahoo and K. Khandekar, Biomacromolecules, 2002, 3, 1087–1094 CrossRef CAS PubMed.
- H. W. Liang, X. Cao, W. J. Zhang, H. T. Lin, F. Zhou, L. F. Chen and S. H. Yu, Adv. Funct. Mater., 2011, 21, 3851–3858 CrossRef CAS.
- T. S. Anirudhan and M. Ramachandran, Ind. Eng. Chem. Res., 2008, 47, 6175–6184 CrossRef CAS.
- Z. Ling, H. Rong and G. Hong, Appl. Surf. Sci., 2006, 253, 2611–2617 CrossRef.
- L. Yu, X. K. Liu, W. C. Yuan, L. J. Brown and D. Y. Wang, Langmuir, 2015, 31, 6351–6366 CrossRef CAS PubMed.
- M. Toor, B. Jin, S. Dai and V. Vimonses, J. Ind. Eng. Chem., 2015, 21, 653–661 CrossRef CAS.
- X. Wang, L. Yang, J. Zhang, C. Wang and Q. Li, Chem. Eng. J., 2014, 251, 404–412 CrossRef CAS.
- M. Solener, S. Tunali, A. S. Ozcan, A. Ozcan and T. Gedikbey, Desalination, 2008, 308–322 CrossRef CAS.
- C. Wang, J. Liu, Z. Zhang, B. Wang and H. Sun, Ind. Eng. Chem. Res., 2012, 51, 4397 CrossRef CAS.
- E. I. Unuabonah, K. O. Adebowale, B. I. Olu-Owolabi, L. Z. Yang and L. X. Kong, Hydrometallurgy, 2008, 93, 1 CrossRef CAS.
- O. Abollino, M. Aceto, M. Malandrino, C. Sarzanini and E. Mentasti, Water Res., 2003, 37, 1619 CrossRef CAS PubMed.
- J. U. K. Oubagaranadin, Z. V. P. Murthy and V. P. Mallapur, C. R. Chim., 2010, 13, 1359–1363 CrossRef CAS.
- I. Langmuir, J. Am. Chem. Soc., 1916, 38, 2221–2295 CrossRef CAS.
- M. N. Shafqat and G. M. Pierzynski, Environ. Sci. Technol., 2014, 99, 72–80 CAS.
- R. Naseem and S. S. Tahir, Water Res., 2001, 35, 3982 CrossRef CAS PubMed.
- S. Yang, D. Zhao, H. Zhang, S. Lu, L. Chen and X. Yu, J. Hazard. Mater., 2010, 183, 632–640 CrossRef CAS PubMed.
- J. L. Yin, M. S. Pei, Y. J. He, Y. K. Du, W. J. Guo and L. Y. Wang, RSC Adv., 2015, 5, 89839 RSC.
|
This journal is © The Royal Society of Chemistry 2016 |
Click here to see how this site uses Cookies. View our privacy policy here.