DOI:
10.1039/C6RA20996C
(Paper)
RSC Adv., 2016,
6, 98935-98944
Coordination-driven multilayer of phosvitin-polyphenol functional nanofibrous membranes: antioxidant and biomineralization applications for tissue engineering†
Received
20th August 2016
, Accepted 12th October 2016
First published on 12th October 2016
Abstract
The layer-by-layer (LBL) deposition technique has been widely used to decorate the nanofibers formed from polymer pairs with complementary functional groups. In the current study, an antioxidative coordination-driven multilayer electrospun nanofibrous film was fabricated from tannic acid (TA) and phosvitin (PV) obtained from egg yolk. PV was reported to bond with 95% of yolk iron which could provide chelating sites for TA. The surface morphology of the nanofibrous mats was observed by scanning electron microscopy (SEM). Also the TEM image of the cross-section illustrated a uniform shell formed around the cellulose nanofibers. The deposition of TA and PV was further confirmed by X-ray photoelectron spectroscopy (XPS) and Fourier transform infrared spectroscopy (FT-IR). TA/PV nanofibrous mats showed good 1,1-diphenyl-2-picrylhydrazyl (DPPH) radical-scavenging activity regardless of the outmost component. While for superoxide-scavenging and hydroxyl radical-scavenging activity, the outmost component affected the scavenging capacity of nanofibrous mats. Scanning electron microscopy (SEM), X-ray photoelectron spectroscopy (XPS) and X-ray diffraction (XRD) were combined to characterize the morphology and structure of the deposited mineral phase on the scaffolds after culturing in a simulated body fluid (SBF) for 5 days. From the result, the TA/PV nanofibrous mats were satisfactory for use in bioapplications.
1. Introduction
In recent years, electrospinning of polymers has become an internationally highly recognized method for the preparation of ultrafine fibers with micro- to nano-scale and controlled surface morphology.1,2 Electrospun nanofibers with a high surface area to volume ratio have received much attention due to their potential applications in the areas of antibacterial coatings, wound dressings, tissue engineering and drug delivery, etc.3–5 To improve the functions for biomedical use, the surfaces of electrospun nanofibers have been decorated with bioactive molecules or drugs after the electrospinning process. Many methods have been explored including plasma treatment, wet chemical methods, surface graft polymerization and layer-by-layer (LBL) deposition techniques.4,6–8 Among the various surface modification techniques, the LBL deposition technique, is of special interest, and has been widely used to decorate the formed nanofibers from polymer pairs with complementary functional groups.9,10 Polyanion/polycation composites, including protein–polysaccharide, polysaccharide–polysaccharide, protein-other polymers and others, could be good candidates as deposition materials to form LBL structured films to improve the properties of the templates.11
In the field of tissue engineering, electrospun nanofibers have been widely used as scaffolds to maintain, repair, or enhance tissue and organ function. Cellulose-based nanofibers that was necessary to catalyze the calcium phosphate mineralization on the scaffold during subsequent exposure to SBF solution, has been used in both hard and soft tissue engineering.12 In addition, the phosphorylation of cellulose fibers could effectively induce the growth of carbonate-containing hydroxyapatite13 to form a 3D nanocomposite network with appropriate mechanical performance.14 So the phosphorylated decoration of cellulose nanofibers was implied for further enhancement in the biomineralization process.
Egg yolk phosvitin (PV) is a highly phosphorylated protein that represents about 7% of the proteins found in egg yolk.15 It possesses very strong metal chelating property due to its polyanionic character. This unique primary structure makes this protein one of the strongest metal chelating agents which bond with 95% of yolk iron.16 The phosphate groups may bond with Ca2+ through coordination bonds, and then induce the growth of Ca–P crystals, regulating the phase transformation of the mineral calcium phosphate in a biomimetic mineralization solution.17 Additionally, PV is more effective than other iron-binding proteins or metal chelators in inhibiting Fe2+-catalyzed hydroxyl radicals (OH) production in Fenton reaction system.18 It also showed a protective effect against OH-mediated damages of DNA in vivo. So PV could be deposited on the surface of nanofibers to further impact the functional properties.
In this current work, freshly prepared electrospun cellulose nanofibrous membranes were first decorated by tannic acid, a polyphenol that known to have antitumor, antibacterial, and antioxidant activity.19 Besides, it is a high-molecular-weight polyphenol and the dominant constituents facilitate interactions with a variety of materials via multiple reaction pathways, including electrostatic, hydrogen bonding, and hydrophobic interactions.20 Furthermore, the strong metal chelation ability and materials surface binding affinity make it a perfect material to act as a polydentate ligand for metal ion coordination.21–23 Previous studies have illustrated that TA can react with Fe3+ ion to form a stable octahedral complex, allowing each TA molecule to react with several Fe3+ centers to form a cross-linked film.24 So the Fe3+ ion in PV could provide metal sites for coordination bonding. The aim of this work was to investigate the free radical scavenging activity of formed TA/PV cellulose scaffolds and evaluate the enhancement on the biomineralization process due to PV adsorption on the cellulose fibers.
2. Materials and methods
2.1. Materials
Cellulose acetate (CA, Mn 30
000) was purchased from Sigma-Aldrich Co., USA. Hen egg yolk phosvitin was isolated from the egg yolk.11 Tannic acid and 1,1-diphenyl-2-picrylhydrazyl (DPPH) was obtained from Aladdin Chemistry Co. Ltd. (Shanghai, China). Superoxide anion assay kit and hydroxyl free radical assay kit was purchased from Nanjing Jiancheng Biotechnology Co., Ltd (Nanjing, China). The other reagents were analytical grade purchased from China National Pharmaceutical Group Industry Corporation Ltd. All aqueous solutions were prepared using ultrapure water with a resistance of 18.2 MΩ cm through a Millipore (Millipore, Milford, MA, USA), Milli-Q water purification system.
2.2. Preparation of cellulose nanofibers
Nanofibrous CA mats were fabricated by our previous method.25,26 Briefly, 17% CA dissolved in acetone–N,N-dimethyl acetamide (DMAc) (2
:
1, w/w) mixed solvent was transferred into a plastic syringe driven by a syringe pump (LSP02-1B, Baoding Longer Precision Pump Co., Ltd., China). A grounded cylindrical layer was used as a collector which rotated with a linear velocity of 100 m min−1. The applied voltage was 17 kV and the tip-to-collector distance was 20 cm. The ambient temperature and relative humidity were maintained at 25 °C and 45%, respectively. The prepared nanofibrous mats were dried at 80 °C in vacuum for 24 h to remove the trace solvent. Hydrolysis of the CA mats was performed in 0.05 M NaOH solution at ambient temperature for 7 days following the previous reports.27,28
2.3. Formation of nanocomposite films on template nanofibers
The bilayer film was then deposited, by spraying TA (1 mg mL−1, in 0.01 M PBS, pH = 7.4) followed by PV (1 mg mL−1, in 0.01 M PBS, pH = 7.4) each for 10 mL solution. Following each deposition step, the template nanofibers washed with 20 mL PBS (0.01 M, pH = 7.4).19,29 Here, (TA/PV)n was used as a formula to label the LbL structured films, where n was the number of the TA/PV bilayers. The LbL films coated fibrous mats were dried at 40 °C for 2 h under vacuum prior to further characterizations.
2.4. Characterization of composite nanofibrous membranes
The surface morphology of the composite nanofibrous mats was performed by scanning electron microscopy (SEM) (S-4800, Hitachi Ltd., Japan). The cross-sections of the nanofibers were observed using JEOL transmission electron microscope (TEM) (H-7650, Hitachi, Japan). Fourier transform infrared (FT-IR) spectra were acquired on a Nicolet 170-SX instrument (Thermo Nicolet Ltd., USA) in the wavenumber range of 4000–400 cm−1. X-ray photoelectron spectroscopy (XPS) was operated on an axis ultra DLD apparatus (Kratos, U.K.), to identify chemical compositions of the nanofibrous membranes. X-ray diffraction (XRD) was carried out using a diffractometer type D/max-rA (Rigaku Co., Japan) with Cu target and Kα radiation (=0.154 nm).
2.5. In vitro antioxidant activity assay
Superoxide radical-scavenging activity. The superoxide scavenging ability of the nanofibrous membranes was based on the removal rate of xanthine/xanthine oxidase-generated superoxide radical (O2˙−) by measuring the reduction of NBT using superoxide anion assay kit. The reaction mixture, containing nanofibrous mats was incubated at room temperature for 10 min and the color was read at 550 nm against a blank.
DPPH radical-scavenging activity. The antioxidant activity of nanofibrous mats was measured according to the published DPPH scavenging method with minor modification.30 Briefly, scavenging activity assay was carried out by recording the absorbance of DPPH solution (100 μM) at 517 nm in the presence of the nanofibrous mats at room temperature with a UV-vis spectrophotometer. The free radical scavenging ability of the nanofibrous mats was expressed as the percentage of DPPH that was decreased in comparison with that of the control condition after 30 min preservation in the dark.
Hydroxyl radical-scavenging activity. The hydroxyl radical-scavenging activity was assayed based on typical Fenton reaction using hydroxyl free radical assay kit. The reaction mixture, containing nanofibrous mats was incubated at room temperature for 20 min and the color was read at 550 nm against a blank.
2.6. Biomimetic growth of HAP on nanofibers
The 1.5 times simulated body fluid (1.5 × SBF) was prepared as reported.31 The composite nanofibrous mats were incubated in 1.5 × SBF at 37 °C for 5 days. Fresh SBF solution was replaced once every other day. At desired time, membranes were taken out from SBF solution, washed with deionized water and finally freeze-dried.
2.7. Statistics analysis
Data are presented as the mean ± standard deviation. Statistical comparisons were analyzed by ANOVA analysis and the Tukey post hoc test using SPSS 11.5 software. A value of p < 0.05 was considered to be significant.
3. Results and discussion
3.1. Preparation of TA/PV nanofibrous mats
PV is a heavily phosphorylated protein and the phosphoserines are arranged in a singular way, forming blocks that can carry up to 15 consecutive residues.32 In the egg yolk, PV almost bond with about 95% of the whole ferric ions (Fe3+) due to the strong metal chelation ability.33–35 TA containing galloyl groups, also has the strong metal chelation ability which can be use as a polydentate ligand for metal ion coordination. Then we would like to explore whether TA could be coordinated to Fe3+ bonded in PV. As known, Fe3+–TA complexes exhibit dark purple color at pH 7.4 and have an adsorption at around 530 nm.36,37 The color of the complexes suspension was pH-dependent which can be attributed to transitions between mono-, bis-, and tris-states of Fe3+–TA complexes.24 In Fig. 1, there existed an adsorption at around 530 nm which indicated the formation of Fe3+–TA complexes. Additionally, the color of the TA–PV solution changed to purple. The result indicated indirectly TA could interact with Fe3+ in PV by coordination bonding.
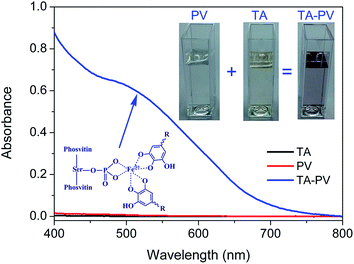 |
| Fig. 1 UV spectra of TA, PV, and TA/PV and the digital image of TA, PV, TA/PV. | |
In our previous study, the formation of hydrogen bonding between TA and cellulose led to the adsorption of TA onto the surface of cellulose nanofibrous mats.6 After the decoration of TA, PV was then deposited on the surface of TA modified nanofibrous mats. Subsequently, the nanofibrous mats were fabricated by depositing more layers of TA and PV alternately on the surface through vacuum-assisted LBL assembly method. The multilayer nanofibrous films were constructed by coordination bonding between TA and Fe3+ in PV. The structure of TA/PV nanofibrous mats was shown in Scheme 1. The color of the mats changed from white to light purple, which indicated the formation of “PV–Fe3+–TA” complexes.
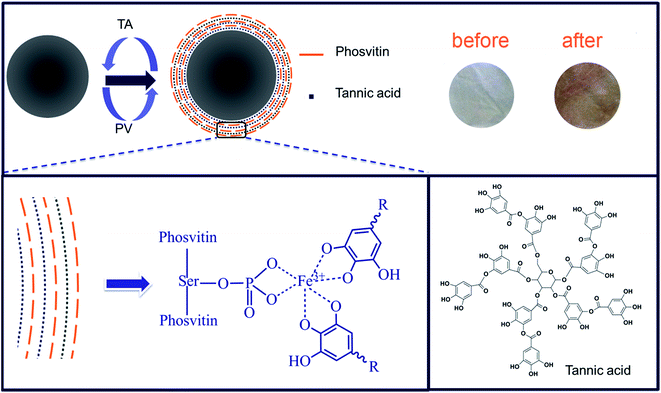 |
| Scheme 1 Scheme of layer-by-layer assembly of TA/PV composite nanofibrous mats and the structural formula of tannic acid. | |
3.2. Surface morphology analysis of TA/PV nanofibrous mats
The impact of TA and PA deposition on the morphology of cellulose nanofibrous membranes was conducted by SEM. In Fig. 2a and a′, the representative SEM images of cellulose nanofibrous mats revealed randomly oriented 3D nonwoven mats with uniform diameter (average diameter of 471.5 nm) and possessed loosely packed cylindrical fibers, continuous and long without any defects. After LBL coating process, it could be observed that the average diameter of the fibers increased compared with cellulose nanofibers. In order to get a more direct image of LBL coating, TEM was used to observe the cross section of (TA/PV)9 nanofibrous mat (Fig. S1†). It can be clearly seen that a uniform shell formed around the cellulose nanofibers. These images visually demonstrated that TA and PV were successfully assembled onto the surface of the cellulose fibers.
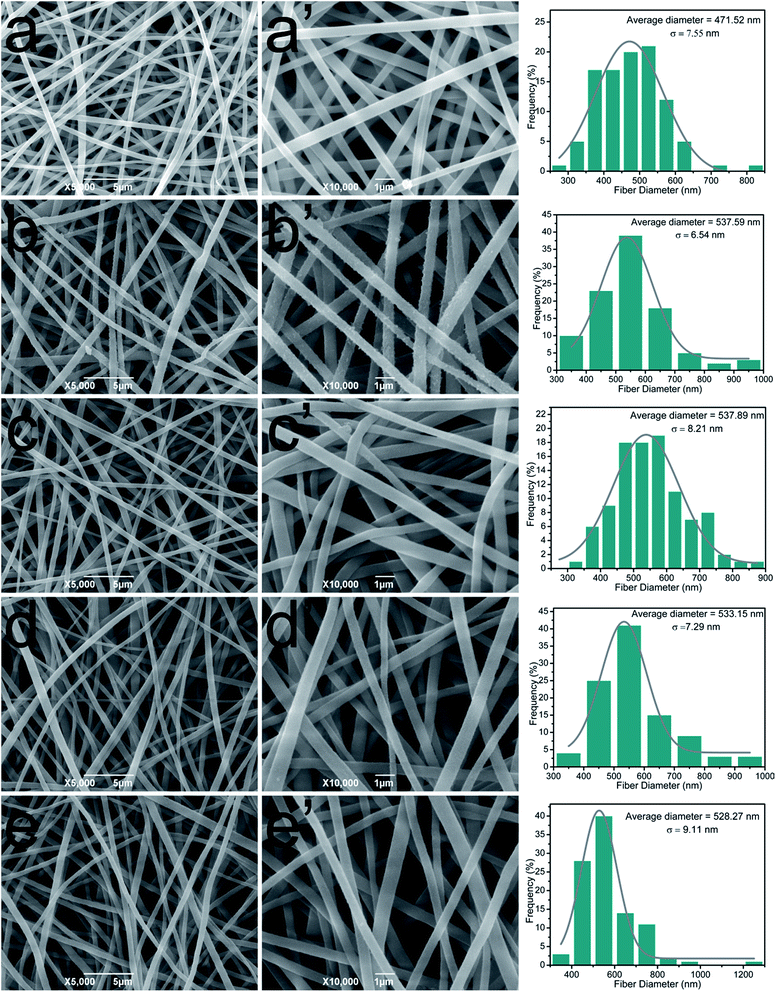 |
| Fig. 2 SEM images of (a–e): cellulose nanofibrous mats, (TA/PV)3, (TA/PV)6, (TA/PV)6.5, (TA/PV)9. Image (a′–e′) showed high magnification images of a–e, respectively. The right column reveals the diameter distribution histograms of the nanofibrous mats. | |
3.3. FT-IR spectra of TA/PV nanofibrous mats
The representative spectra of the raw materials and the composite nanofibrous membranes were shown in Fig. 3. From the FTIR spectra, we found the membranes showed an abroad absorption band at about 3500–3200 cm−1, which was attributed to the free O–H stretching vibration of hydroxyl groups.38 For the LBL coated films, the observed shoulder peak at 1716 cm−1 was due to the stretching band of carbonyl groups in TA ester bond.39 The new peak at 1203 cm−1 appeared in the spectra of TA/PV nanofibrous mats resulted from phenolic C–O stretching vibrations.36,40,41 On the base of the above results, we could see that TA was deposited on the surface of the cellulose fibers.
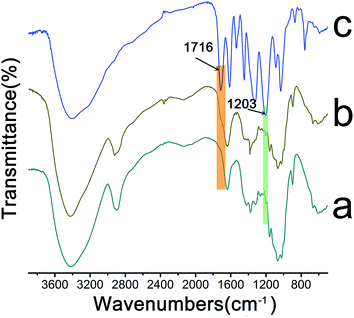 |
| Fig. 3 FT-IR spectra of: cellulose fibrous mats (a), (TA/PV)6.5 (b) and TA (c). | |
3.4. Surface composition analysis of TA/PV nanofibrous mats
To further confirm the deposition of PV and TA on the LbL films, XPS analysis was performed to identify the surface chemical composition of the composite nanofibrous mats. The overview spectra revealed that C 1s, N 1s, O 1s, and P 2p existed obviously in TA/PV nanofibrous mats (Fig. 4a). As sown in Fig. 4b, the C 1s core-level photoelectron spectrum can be curved into three peak components located at 284.6 eV, 286.0 eV, and 288.2 eV, assigned to C–C, C–O, and C
O or O–C
O group from TA or PV.22,42 The N 1s spectrum had one peak centered at 399.8 eV which was characteristic of pyridinic nitrogen (sp2 hybridization),43 and it was associated with the assignment of the binding energy of C–N covalent bonds of PV. Moreover, in the spectrum of O 1s, a major peak at 533.5 eV and a relatively small peak at 531.6 eV, which can be ascribed to the C
O and HO–C groups respectively,36 also confirm the existence of TA or PV. PV is a phosphoglycoprotein present in egg yolk,15,16 the characteristic element of phosphorus further verified that PV was assembly on the surface of cellulose films successfully. The relative weak Fe 2p signal ranging from 710.6 eV to 724.2 eV implied the existence of Fe3+ in PV.
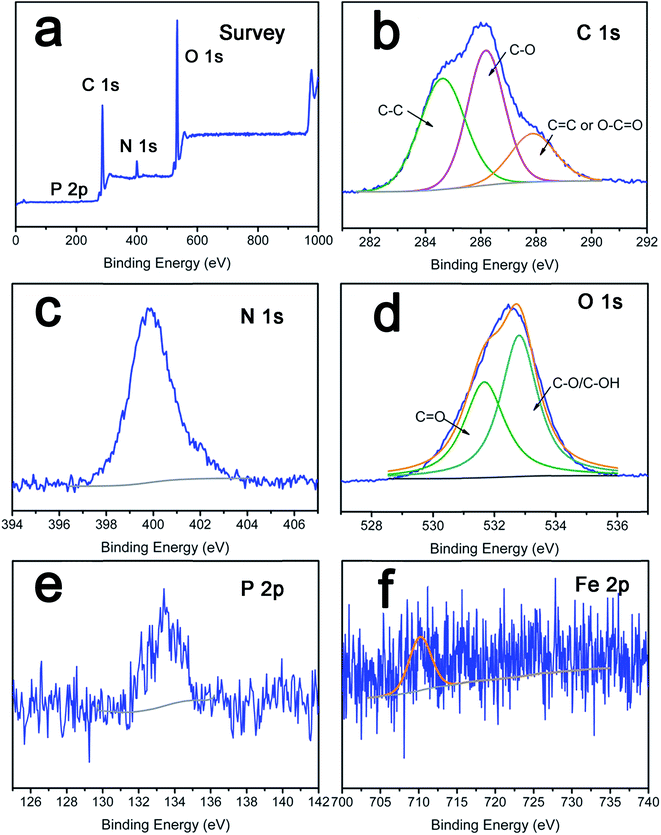 |
| Fig. 4 XPS spectra of (TA/PV)9 composite nanofibrous mats. | |
3.5. Free radical scavenging activity of TA/PV nanofibrous mats
Free radicals, which, if overproduced, indiscriminately attack on DNA, proteins, and other important biomolecules, and are associated with many degenerative conditions.20,44 TA and PV have well been reported for the free radical scavenging activity and antioxidant activity against lipid peroxidation in foods.6,45,46 So it is necessary to evaluate the protective effects of its antioxidant activity in this system. Antioxidant activity of the nanofibrous mats were evaluated by determining the scavenging activities against free radicals including superoxide radicals, DPPH radicals, and hydroxyl radicals.
The superoxide-scavenging activity of nanofibrous mats was measured using the xanthine–xanthine oxidase system, and the results were indicated as the inhibition rate of superoxide activity. From the result in Fig. 5A, cellulose nanofibrous mats had no effect on the superoxide-scavenging activity, while the deposition of PV and TA on the fibers impacted the superoxide-scavenging activity of composite nanofibrous mats. Additionally, the superoxide-scavenging capacity of these membranes increased with the increase of the number of bilayer when PV was chosen as the outmost layer. The scavenging rate of (TA/PV)3, (TA/PV)6, (TA/PV)9 was about 27%, 30% and 35% respectively. However, when the outmost component was TA, the superoxide-scavenging capacity of (TA/PV)6.5 decreased dramatically compared to (TA/PV)6, which might be due to the higher superoxide-scavenging activity of PV than TA.
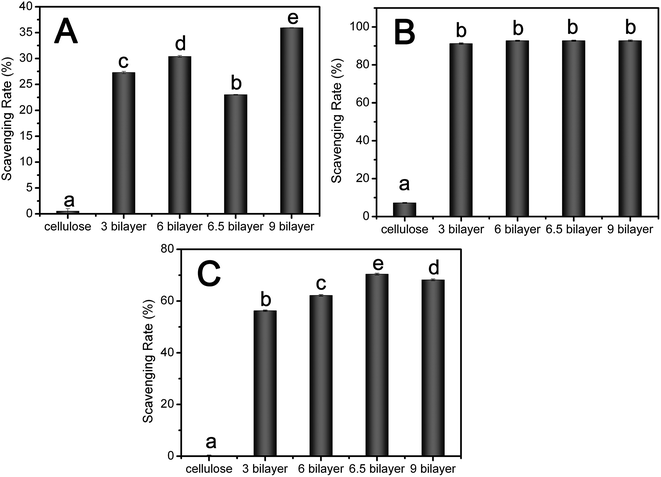 |
| Fig. 5 Superoxide radical scavenging activities of cellulose nanofibrous mats and composite nanofibrous mats (A); DPPH radical scavenging activities of cellulose nanofibrous mats and composite nanofibrous mats (B); hydroxyl radical scavenging activities of cellulose nanofibrous mats (C). | |
DPPH is a free radical compound and has been widely employed to test the free radical-scavenging ability of various samples.46–49 Fig. 5B showed the scavenging activity of nanofibrous mats on DPPH radicals, in which the TA/PV nanofibrous mats exhibited good antioxidant performance compared with the cellulose nanofibrous mats, reaching 90% for all composite nanofibrous mats. The outmost layer had no significant effect on the scavenging ability.
The hydroxyl radical, among the oxygen radicals, is the most reactive radicals, which could severely damages adjacent biomolecules.46 The scavenging effect against hydroxyl radicals was investigated by using the Fenton reaction and the inhibition rate was used to evaluate the scavenging ability. According to Fig. 5C, TA/PV nanofibrous mats showed hydroxyl radical-scavenging activity and its activity increased with an increase in bilayer number, exhibiting 56%, 63% and 68% scavenging activity for (TA/PV)3, (TA/PV)6, (TA/PV)9 respectively. The (TA/PV)6.5 nanofibrous mats exhibited 70% scavenging activity higher than that of (TA/PV)6. This result showed that TA has strong hydroxyl radical-scavenging effects, so the presence of TA on the outmost layer enhanced the hydroxyl radical-scavenging activity of the nanofibrous mats.
From the result mentioned above, it obviously illustrated that the fabricated LBL deposited nanofibrous membranes showed good radical-scavenging activity which displayed promising applications in the area of wound dressing to scavenge the free racial and promote the wound healing process.50–52
3.6. Biomimetic growth of HAP on nanofibers
PV, a heavily phosphorylated protein shows a very strong affinity to bivalent metals such as calcium, magnesium, and iron.18 At pH 6.5, PV binds up to 127 ions of Ca2+.32 These peculiar structures and properties suggest that PV should have biological properties similar to phosphorylated proteins which can initiate and regulate the formation of mineral crystals, and may play a vital role in biological mineralization in human hard tissues.17,53 Several researchers have shown that PV can promote calcium phosphate rapidly transformed into hydroxyapatite (HAP) when immobilized on a substrate surface in vitro.53,54 HAP, a major inorganic component of natural bone, is a biomimetic material with good biocompatibility and bioactivity and it has been widely used in bone replacement systems.55,56 In this study, we would like to investigate the effect of TA/PV nanofibrous mats in promoting the transformation to HAP.
The morphology changes of nanofibrous mats surface were identified from the SEM micrographs. For the cellulose nanofibrous mats, biomimetic crystal growth was nucleated from the fiber surface within 5 days of culturing (Fig. 6a and a′). When deposited of PV and TA on the fibers, it showed increased mineralization with the increase of the bilayer number as the fibers were more decorated with mineralized clusters. On (TA/PV)9 nanofibrous mats, it began to present a loose, porous, amorphous shape and finally the particles gathered into a massive structure covered around the fibers. These results suggested that cellulose nanofibrous mats could induce HAP formation under SBF treatment. And the deposition of PV and TA on the fibers could promote the transformation to HAP, especially for (TA/PV)9 nanofibrous mats.
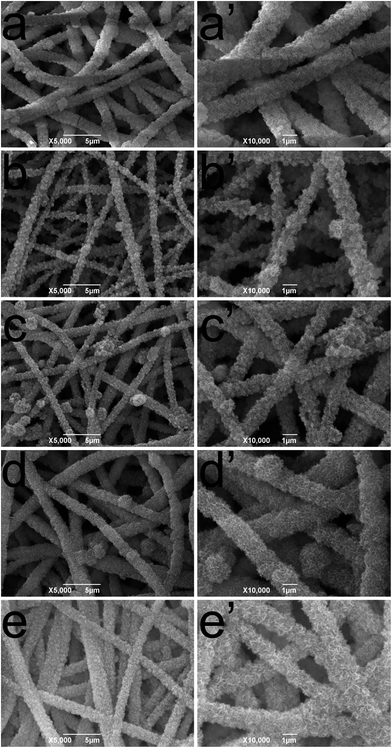 |
| Fig. 6 SEM images of nanofibrous mats after incubated in 1.5 × SBF for 5 days (a–e): cellulose nanofibrous mats, (TA/PV)3, (TA/PV)6, (TA/PV)6.5, and (TA/PV)9. Image (a′–e′) showed high magnification images of a–e, respectively. | |
The crystalline phases of (TA/PV)6.5 and HAP–(TA/PV)6.5 nanofibrous mats were characterized by XRD measurements. As shown in Fig. 7a, (TA/PV)6.5 mats exhibited obvious crystalline peaks 12.2, 20.1, and 21.8 corresponding to typical cellulose crystal.11 After formed HAP on the (TA/PV)6.5 nanofibrous mats, XRD peaks observed at 2θ equal to 25.7, 31.8, 39.9, 46.8 and 49.5 correspond to HAP as highlighted by the reference spectrum.12,57,58
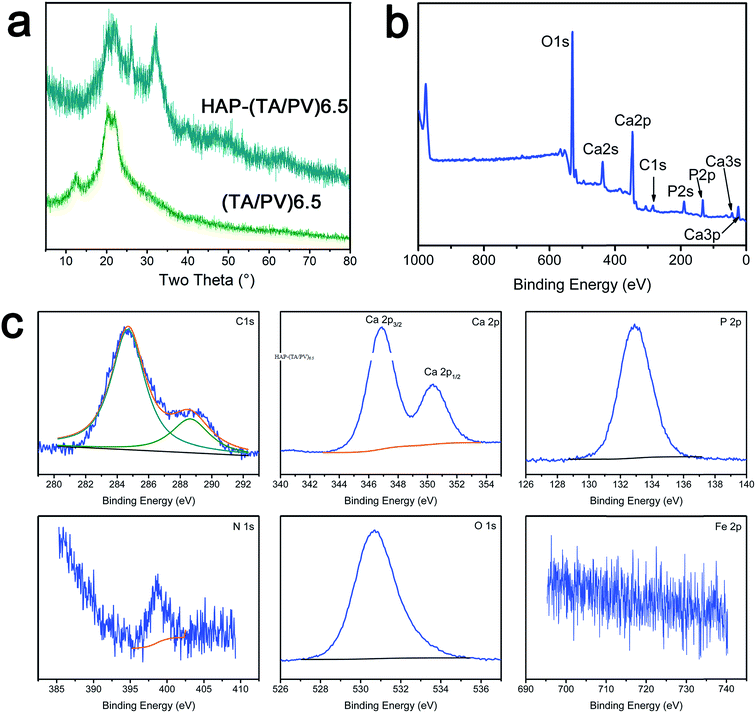 |
| Fig. 7 XRD patterns of (TA/PV)6.5 and HAP–(TA/PV)6.5 (a), XPS spectra of HAP–(TA/PV)9 (b), core-level spectra of C 1s, Ca 2p, P 2p, N 1s, O 1s and Fe 2p (c). | |
Surface chemistry of HAP–(TA/PV)6.5 nanofibrous mats was analyzed by XPS (Fig. 7b and c) and the element content was listed in Table S1.† In the survey scan spectrum of HAP–(TA/PV)6.5 nanofibrous mats, Ca 2s, Ca 2p and Ca 3s core-levels existed obviously (Fig. 7b). Additionally, the intensity of P 2s and P 2p peak increased dramatically compared with these in (TA/PV)6.5 nanofibrous. As shown in Fig. 7c, the peak of the O–C group in the C 1s spectra shifted from 286.5 eV to a lower binding energy of 285.4 eV, which could be attributed to the interaction of –OH group and HAP. This interaction also led to the shift in binding energy of O 1s. Because HAP covered the surface of the nanofibrous mats, the intensity of N 1s peak decreased and the weak peak of Fe 2p disappeared. In addition, the C/O ratio in HAP–(TA/PV)6.5 nanofibrous mats decreased significantly from 1.9 to 0.15 calculated from Table S1.† The Ca/P ratio in HAP–(TA/PV)6.5 nanofibrous mats was 1.44, which is less than that of the pure HAP (Ca/P = 1.67),58 indicating that the HAP formed in this system is calcium deficient. These excellent properties bring it promising applications in tissue engineering.
4. Conclusions
TA and PV were alternatively deposited on the surface of cellulose nanofibrous mats through the coordination bonding. PV was reported to bond with 95% of yolk iron which could provide chelating sites for TA. The deposition of TA/PV assembly can be clearly seen from the TEM image of the cross-section. The TA/PV nanofibrous mats showed good antioxidant activity including superoxide-scavenging, DPPH radical and hydroxyl radical-scavenging activity, which exhibited potential application as wound dressing to promote the wound healing. After cultured in 1.5 × SBF solution, the surface of the nanofibers covered by the HAP crystals, especially for (TA/PV)9 nanofibrous mats. This TA/PV nanofibrous mats is also a promising scaffold in tissue engineering. Additionally, the coordination bonding between PV and TA is pH-responsive, which impacts the system potential applications in pH-stimuli delivery of polyphenolic drug or other types of drug.
Acknowledgements
This work was financially supported by Fundamental Research Funds for the Central Universities (Grant No. 0900206196) and Hubei Provincial Science-technology Supporting Plan of China (Grant No. 2014BBB018). The authors greatly thank colleagues of Key Laboratory of Environment Correlative Dietology of Huazhong Agricultural University for offering many conveniences.
References
- M. Ignatova, N. Manolova and I. Rashkov, Macromol. Biosci., 2013, 13, 860–872 CrossRef CAS PubMed.
- S. Guzman-Puyol, J. A. Heredia-Guerrero, L. Ceseracciu, H. Hajiali, C. Canale, A. Scarpellini, R. Cingolani, I. S. Bayer, A. Athanassiou and E. Mele, ACS Biomater. Sci. Eng., 2016, 2, 526–534 CrossRef CAS.
- N. Wang, X. Wang, B. Ding, J. Yu and G. Sun, J. Mater. Chem., 2012, 22, 1445–1452 RSC.
- B. V. Worley, R. J. Soto, P. C. Kinsley and M. H. Schoenfisch, ACS Biomater. Sci. Eng., 2016, 2, 426–437 CrossRef CAS.
- S. Müller-Herrmann and T. Scheibel, ACS Biomater. Sci. Eng., 2015, 1, 247–259 CrossRef.
- B. Zhou, X. Hu, J. Zhu, Z. Wang, X. Wang and M. Wang, Int. J. Biol. Macromol., 2016, 91, 68–74 CrossRef CAS PubMed.
- H. S. Yoo, T. G. Kim and T. G. Park, Adv. Drug Delivery Rev., 2009, 61, 1033–1042 CrossRef CAS PubMed.
- B. Zhou, Y. Li, H. Deng, Y. Hu and B. Li, Colloids Surf., B, 2014, 116, 432–438 CrossRef CAS PubMed.
- H. Deng, X. Wang, P. Liu, B. Ding, Y. Du, G. Li, X. Hu and J. Yang, Carbohydr. Polym., 2011, 83, 239–245 CrossRef CAS.
- L. Zhou, M. Chen, L. Tian, Y. Guan and Y. Zhang, ACS Appl. Mater. Interfaces, 2013, 5, 3541–3548 CAS.
- B. Zhou, Y. Hu, J. Li and B. Li, Int. J. Biol. Macromol., 2014, 64, 402–408 CrossRef CAS PubMed.
- K. Rodríguez, S. Renneckar and P. Gatenholm, ACS Appl. Mater. Interfaces, 2011, 3, 681–689 Search PubMed.
- S. Sahu, B. Behera, T. K. Maiti and S. Mohapatra, Chem. Commun., 2012, 48, 8835–8837 RSC.
- K. Li, J. Wang, X. Liu, X. Xiong and H. Liu, Carbohydr. Polym., 2012, 90, 1573–1581 CrossRef CAS PubMed.
- S. P. Volk, D. U. Ahn, M. Zeece and S. Jung, J. Sci. Food Agric., 2012, 92, 3095–3098 CrossRef CAS PubMed.
- M. Anton, O. Castellani and C. Guerin Dubiard, Bioactive Egg Compounds, Springer, 2007 Search PubMed.
- X. Zhang, F. Geng, X. Huang and M. Ma, J. Cryst. Growth, 2015, 409, 44–50 CrossRef CAS.
- H. Samaraweera, W. G. Zhang, E. J. Lee and D. U. Ahn, J. Food Sci., 2011, 76, 143–150 CrossRef PubMed.
- A. Shukla, J. C. Fang, S. Puranam, F. R. Jensen and P. T. Hammond, Adv. Mater., 2012, 24, 492–496 CrossRef CAS PubMed.
- H. Liang, Y. Pei, J. Li, W. Xiong, Y. He, S. Liu, Y. Li and B. Li, RSC Adv., 2016, 6, 31374–31385 RSC.
- H. Liang, J. Li, Y. He, W. Xu, S. Liu, Y. Li, Y. Chen and B. Li, ACS Biomater. Sci. Eng., 2016, 2, 317–325 CrossRef CAS.
- H. Liang, B. Zhou, J. Li, W. Xu, S. Liu, Y. Li, Y. Chen and B. Li, Colloids Surf., B, 2015, 136, 1224–1233 CrossRef CAS PubMed.
- Y. Ping, J. Guo, H. Ejima, X. Chen, J. J. Richardson, H. Sun and F. Caruso, Small, 2015, 11, 2032–2036 CrossRef CAS PubMed.
- H. Ejima, J. J. Richardson, K. Liang, J. P. Best, M. P. van Koeverden, G. K. Such, J. Cui and F. Caruso, Science, 2013, 341, 154–157 CrossRef CAS PubMed.
- H. Deng, X. Zhou, X. Wang, C. Zhang, B. Ding, Q. Zhang and Y. Du, Carbohydr. Polym., 2010, 80, 474–479 CrossRef CAS.
- B. Zhou, X. Jin, L. Hongshan, J. Li, S. Liu, Y. Li, Y. Chen and B. Li, RSC Adv., 2015, 5, 26965–26971 CAS.
- J. Du, X. Li, C. Yang, W. Li, W. Huang, R. Huang, X. Zhou and H. Deng, Curr. Nanosci., 2013, 9, 8–13 CAS.
- W. Li, X. Li, Q. Wang, Y. Pan, T. Wang, H. Wang, R. Song and H. Deng, Carbohydr. Polym., 2014, 99, 218–225 CrossRef CAS PubMed.
- J. C. Antunes, C. L. Pereira, M. Molinos, F. Ferreira-da-Silva, M. Dessi, A. Gloria, L. Ambrosio, R. M. Goncalves and M. A. Barbosa, Biomacromolecules, 2011, 12, 4183–4195 CrossRef CAS PubMed.
- B. Zhou, J. Xing, J. Li, W. Xu, Y. Li and B. Li, RSC Adv., 2014, 4, 54517–54524 RSC.
- H. Liu, J. Wang, W. Qin and X. Liu, RSC Adv., 2013, 3, 11132–11139 RSC.
- O. Castellani, C. Guérin-Dubiard, E. David-Briand and M. Anton, Food Chem., 2004, 85, 569–577 CrossRef CAS.
- S. Nakamura, M. Ogawa, S. Nakai, A. Kato and D. D. Kitts, J. Agric. Food Chem., 1998, 46, 3958–3963 CrossRef CAS.
- R. Huopalahti, M. Anton, R. López-Fandiño and R. Schade, Bioactive egg compounds, Springer, 2007 Search PubMed.
- C. Guérin-Dubiard, M. Anton, A. Dhene-Garcia, V. Martinet and G. Brulé, Eur. Food Res. Technol., 2002, 214, 460–464 CrossRef.
- J. Guo, Y. Ping, H. Ejima, K. Alt, M. Meissner, J. J. Richardson, Y. Yan, K. Peter, D. von Elverfeldt and C. E. Hagemeyer, Angew. Chem., Int. Ed., 2014, 53, 5546–5551 CrossRef CAS PubMed.
- T. S. Sileika, D. G. Barrett, R. Zhang, K. H. A. Lau and P. B. Messersmith, Angew. Chem., Int. Ed., 2013, 52, 10766–10770 CrossRef CAS PubMed.
- X. Cao, B. Ding, J. Yu and S. S. Al-Deyab, Carbohydr. Polym., 2012, 90, 1075–1080 CrossRef CAS PubMed.
- M. M. Kumari, S. A. Aromal and D. Philip, Spectrochim. Acta, Part A, 2013, 103, 130–133 CrossRef PubMed.
- A. Dutta and S. K. Dolui, Appl. Surf. Sci., 2011, 257, 6889–6896 CrossRef CAS.
- E. Bulut and M. Özacar, Ind. Eng. Chem. Res., 2009, 48, 5686–5690 CrossRef CAS.
- T. Zeng, X. Zhang, Y. Guo, H. Niu and Y. Cai, J. Mater. Chem. A, 2014, 2, 14807–14811 CAS.
- W. Li, X. Li, Q. Wang, Y. Pan, T. Wang, H. Wang, R. Song and H. Deng, Carbohydr. Polym., 2014, 99, 218–225 CrossRef CAS PubMed.
- B. Nemzer, T. Chang, Z. Xie, Z. Pietrzkowski, T. Reyes and B. Ou, Food Sci. Nutr., 2014, 2, 647–654 CrossRef CAS PubMed.
- M. Shin, K. Kim, W. Shim, J. W. Yang and H. Lee, ACS Biomater. Sci. Eng., 2016, 2, 687–696 CrossRef CAS.
- S. Sakanaka and Y. Tachibana, Food Chem., 2006, 95, 243–249 CrossRef CAS.
- S. Benvenuti, F. Pellati, M. a. Melegari and D. Bertelli, J. Food Sci., 2004, 69, 164–169 Search PubMed.
- R. van Lith, X. Wang and G. A. Ameer, ACS Biomater. Sci. Eng., 2016, 2, 268–277 CrossRef CAS PubMed.
- J. Bhaumik, N. S. Thakur, P. K. Aili, A. Ghanghoriya, A. K. Mittal and U. C. Banerjee, ACS Biomater. Sci. Eng., 2015, 1, 382–392 CrossRef CAS.
- S. Shetty, S. Udupa and L. Udupa, J. Evidence-Based Complementary Altern. Med., 2008, 5, 95–101 CrossRef PubMed.
- D. Gopinath, M. R. Ahmed, K. Gomathi, K. Chitra, P. Sehgal and R. Jayakumar, Biomaterials, 2004, 25, 1911–1917 CrossRef CAS PubMed.
- K. Gouthamchandra, R. Mahmood and H. Manjunatha, Environ. Toxicol. Pharmacol., 2010, 30, 11–18 CrossRef CAS PubMed.
- K. Onuma, J. Phys. Chem. B, 2005, 109, 8257–8262 CrossRef CAS PubMed.
- S. Ito, M. Iijima, F. Motai, I. Mizoguchi and T. Saito, J. Biomed. Mater. Res., Part A, 2012, 100, 2760–2765 CrossRef PubMed.
- Q. Ruan, D. Liberman, Y. Zhang, D. Ren, Y. Zhang, S. R. Nutt and J. Moradian-Oldak, ACS Biomater. Sci. Eng., 2016, 2, 1049–1058 CrossRef CAS.
- M. Wu, Q. Wang, X. Liu and H. Liu, Carbon, 2013, 51, 335–345 CrossRef CAS.
- C. J. Grande, F. G. Torres, C. M. Gomez and M. C. Bañó, Acta Biomater., 2009, 5, 1605–1615 CrossRef CAS PubMed.
- S. A. Hutchens, R. S. Benson, B. R. Evans, H. M. O'Neill and C. J. Rawn, Biomaterials, 2006, 27, 4661–4670 CrossRef CAS PubMed.
Footnote |
† Electronic supplementary information (ESI) available. See DOI: 10.1039/c6ra20996c |
|
This journal is © The Royal Society of Chemistry 2016 |