DOI:
10.1039/C6RA17209A
(Paper)
RSC Adv., 2016,
6, 79180-79184
Tetranuclear Zn2Ln2 coordination clusters as catalysts in the Petasis borono-Mannich multicomponent reaction†
Received
5th July 2016
, Accepted 10th August 2016
First published on 15th August 2016
Abstract
We report herein for the first time the efficiency of heteronuclear Zn/Ln coordination clusters (CCs) as catalysts for the multicomponent Mannich-type condensation that involves amines, aldehydes and boronic acids, known as the Petasis borono-Mannich (RBR) reaction. The reaction proceeds in very good to excellent yields (84–98%, 17 products) at room temperature with catalyst loadings as low as 1.0 mol%.
Introduction
The coordination chemistry of 3d and 4f polynuclear coordination clusters (CCs) offers many opportunities to connect Chemistry and Materials Science. The possibility of combining the properties of 3d as well as 4f elements within one molecule has received tremendous attention due to the fascinating structures1–3 and properties ranging from molecular magnetism,4–6 magnetic resonance7 and luminescence.8,9 So far, however, there are few examples of bimetallic 3d/4f CCs as efficient catalysts for water10 or alcohol oxidation,11 and our group has showcased the efficiency of isoskeletal12 3d/4f CCs in domino electrocyclization13,14 and Friedel–Crafts alkylation reactions.15,16
Multicomponent reactions (MCRs) dominate synthetic chemistry for the following reasons: they yield products from simple starting materials, in fewer steps, and in a shorter time when the reactions are carried out in a combinatorial way.17–28 Among these transformations the Mannich-type condensation that involves amines, aldehydes and boronic acids, developed by Petasis,29 known as the Petasis borono-Mannich (PBR) reaction has received considerable attention because it produces skeletons that can easily be converted to amino acids, heterocycles and alkylaminophenols.30
More specific, the reaction shown in Scheme 1 in which aryl boronic acids react with amines and salicylaldehydes yield in a single step novel aminophenol derivatives31 which are suitable for the preparation of dihydro-1,3-oxazines,32,33 triarylmethanes,34 and polycyclic N,O-acetals.30 It is worth noting that aldehydes lacking an OH group in position 2 failed to deliver the desired product, showcasing the importance of the hydroxyl group in the aldehyde to activate the boronic acid.30 The first report for the specific transformation shown in Scheme 1 incorporated a reaction at 90 °C, in dioxane as solvent, in 16 hours and in absence of catalyst in very good yields.35 In 2004, Tye et al. developed a rapid, microwave assisted protocol for carrying this reaction that required a 10 min reaction time.36 In 2009, Gois et al., reported the synthesis of several alkylaminophenols in moderate to good yields, in water, at 80 °C, in absence of catalyst.37 Recently, an effective and rapid synthesis was carried out using protonated trititanate (H2Ti3O7) nanotubes as a heterogeneous solid–acid catalyst.38 However, these methods suffer from a number of disadvantages such as high temperatures, high catalyst loadings (10%) and long-time reactions.
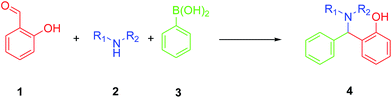 |
| Scheme 1 An example of the Petasis borono-Mannich (PBR) reaction used in this study. | |
We recently started to study the catalytic properties of 3d/4f CCs stabilized by the Schiff base organic ligand [(E)-(2-hydroxy-3-methoxybenzylidene-amino)phenol], H2L.39 These CCs are very easily accessible in two steps. The first step involves the synthesis of the ligand H2L (yield > 98%) from cheap and commercially available materials (o-vanillin and 2-aminophenol) and the second step involves the synthesis of the CC in high yields (>80%) at ambient conditions. We showed that compounds [ZnII2LnIII2L4(NO3)2(DMF)2] (5)15 (Fig. 1) where Ln is Y (5Y),40 Nd (5Nd), Gd (5Gd), Dy (5Dy), Tb (5Tb) and Yb (5Yb) retain their core intact in solution as confirmed by ESI-MS, EPR and NMR15 and are highly efficient catalysts in two Friedel–Crafts type reactions of indole; with aldehydes15 and with substituted nitro-styrenes.16 In both cases, the reactions proceed smoothly with as low as 1 mol% catalyst loadings, in environmentally friendly solvents, with excellent yields (80–100%) and with wide substrate scope. Having in mind that the Zn–Y/Ln metals are very close (∼3.3 Å) in this specific topology and observing a chelation preference of aldehydes15 to Y/Ln metals in 5, we envisioned that these catalysts would be an ideal synergistic template for the MCR reaction shown in Scheme 1. Therefore, we employed compounds 5 as catalysts in the title reaction (Scheme 1) and the results of this study are presented herein.
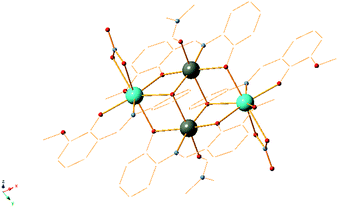 |
| Fig. 1 Molecular structure of 5Ln. Colour code: ZnII: grey; LnIII: light blue; O: red; N: blue. C and H atoms are omitted for clarity. | |
Experimental section
General methods
All data supporting this study are provided as ESI accompanying this paper.† All chemicals and solvents were purchased from Sigma Aldrich, S. D. Fine Chemicals, and commercial suppliers. The progress of the reaction was monitored by thin layer chromatography (TLC) using Merck silica gel 60 F254 plates. Products were purified by column chromatography on silica gel (60–120 mesh). NMR spectra were collected using a Bruker Advance III HD 500 MHz spectrometer. The 1H and 13C NMR spectroscopic data were analysed with a 500 MHz spectrometer in either CDCl3. Chemical shifts are reported in parts per million (δ) relative to tetramethylsilane as the internal standard. The coupling constants (J) are reported in Hz, and the splitting patterns of the proton signals are described as s (singlet), d (doublet), t (triplet), and m (multiplet).
Synthesis of complexes.
All CCs were synthesised according to the literature.15
General procedure for the multicomponent reaction.
All experiments were carried out in the open atmosphere and on a mmol scale. A 10 mL round bottom flask was charged with catalyst 1Dy (1.0 mol%), 2-hydroxybenzaldehyde, secondary amine and boronic acid. Solvent (5 mL) was added and the reaction mixture was stirred for 12–16 h at room temperature. The product was purified by silica gel column chromatography.
Single crystal X-ray structure determinations.
Crystals suitable for single crystal X-ray diffraction analyses were obtained for compound 4aba. Preliminary data on the space group and unit cell dimensions as well as intensity data were collected on an Agilent Xcalibur Eos Gemini Ultra diffractometer with CCD plate detector under a flow of nitrogen gas at 173(2) K using Mo Kα radiation (λ = 0.71073 Å). CRYSALIS CCD and RED software. Reflection intensities were corrected for absorption by the multi-scan method. Structure solution and refinement were accomplished using Olex2,41 solved using either Superflip42 and refined with SHELXL.43 The non-hydrogen atoms were refined with anisotropic thermal parameters. Hydrogen atoms were geometrically fixed and allowed to refine using riding model. Geometric/crystallographic calculations were performed using Olex2,41 package; graphics were prepared with Crystal Maker.44 CCDC 1486441.†
Results and discussion
Our first step was to optimize the reaction conditions for the multicomponent reaction. Thus, we tested several reaction parameters, such as the use of solvents (Table 1), different catalysts (Table 2), temperature (Table 3) and catalyst loading (Table 4). In previous studies 5Y and 5Dy showed similar catalytic efficacy at room temperature,15,16 thus we decided to perform the solvent screening reactions with 5Dy. We studied the reaction between salicylic aldehyde (0.50 mmol) 1a, indoline (0.50 mmol) 2a and phenylboronic acid (0.50 mmol) 3a and a catalyst loading of 1.0% mmol% at room temperature. As can be seen from Table 1, 1,2-dimethoxyethane (DME) appears to be the most suitable solvent (entry 5, Table 1). An almost full conversion is achieved. The use of THF, toluene or ethanol, gave the anticipated product in substantially lower yields (entries 1–4, Table 2), showcasing an inhibitory influence on the reaction. Interestingly, the use of DMSO or DMF yielded very low conversions (entries 6 and 7, Table 2).
Table 1 Influence of the solventa
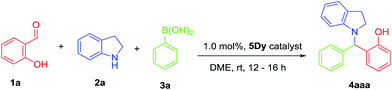
|
Entry |
Solvent |
Yieldb [%] |
Reaction conditions: 1a (0.50 mmol), 2a (0.50 mmol), 3a (0.50 mmol), 5 mL solvent, 1.0 mol% 5Dy catalyst, room temperature.
Isolated yield by column chromatography.
|
1 |
Toluene |
34 |
2 |
Water |
28 |
3 |
THF |
40 |
4 |
Ethanol |
80 |
5 |
DME |
96 |
6 |
DMSO |
12 |
7 |
DMF |
14 |
Table 2 MCR of 2-hydroxybenzaldehyde 1a, indoline 2a, phenylboronic acid 3a catalyzed by 5Ln complexesa
Entry |
Catalyst |
Yieldb% |
Reaction conditions: 1a (0.50 mmol), 2a (0.50 mmol), 3a (0.50 mmol), 5 mL solvent, 1.0 mol% 5Dy catalyst, room temperature.
Isolated yield by column chromatography.
|
1 |
None |
40 |
2 |
Zn(OTf)2 |
56 |
3 |
Dy(OTf)3 |
36 |
4 |
5Y
|
74 |
5 |
5Nd
|
55 |
6 |
5Gd
|
54 |
7 |
5Dy
|
96 |
8 |
5Tb
|
66 |
9 |
3Yb
|
57 |
Table 3 Influence of the temperaturea
Entry |
Temperature [°C] |
Yieldb [%] |
Reaction conditions: 1a (0.50 mmol), 2a (0.50 mmol), 3a (0.50 mmol), 5 mL solvent, 1.0 mol% 5Dy catalyst, room temperature.
Isolated yield by column chromatography.
|
1 |
0 |
0 |
2 |
rt |
96 |
3 |
60 |
76 |
4 |
80 |
72 |
5 |
100 |
65 |
6 |
120 |
62 |
Table 4 Catalyst 5Dy loadinga
Entry |
Catalyst loading [5Dy mol%] |
Yieldb% |
Reaction conditions: 1a (0.50 mmol), 2a (0.50 mmol), 3a (0.50 mmol), 5 mL solvent, 1.0 mol% 5Dy catalyst, room temperature.
Isolated yield by column chromatography.
|
1 |
— |
40 |
2 |
0.5 |
57 |
3 |
1.0 |
96 |
4 |
1.5 |
70 |
5 |
2.0 |
40 |
6 |
5.0 |
24 |
We then examined the catalytic efficiency of compounds 5. The results are summarized in Table 1. The desired product 4aaa was obtained in moderate to excellent yields. A blank experiment in the absence of the 3d/4f CCs catalyst showed 40% conversion (Table 1, entry 1). Moderate conversions were obtained in the presence of Dy or Zn salts (Table 2, entries 2 and 3). Both 5Dy and 5Y show higher activity than 5Gd, 5Nd, 5Yb and 5Tb (Table 1, entries 4–9). Therefore, we selected 5Dy for the following experiments. Efforts to recover the catalyst after the MCR completion were not successful.
We then decided to identify the influence of the temperature on the catalytic performance. The reactions were carried out at various temperatures ranging from room temperature to 120 °C (Table 3, entries 1–6). At room temperature (Table 3), the yield of 4aaa was 96%, but 0% at 0 °C. Lower yields were obtained at 60, 80, 100 and 120 °C (Table 3, entries 3–6), therefore the following reactions were performed at temperature.
After optimizing the reaction conditions, we varied the loadings of catalyst 5Dy (Table 4). It was sufficient to use a catalyst loading of 1.0 mol% to obtain a yield up to 96% (Table 4, entry 3). An increase of the catalyst loading from 1.0 mol% to 5 mol% led to remarkable decrease in the yield of the desired product 4aaa (Table 4, entries 3–6).
Further, a decrease in the catalyst loading to 0.5 mol% also showed lower yield of desired product 4aaa (Table 4, entry 2). In absence of catalyst a 40%conversion is observed (Table 4, entry 1). Finally, we determined that the use of 1.0 mol% 5Dy, in DME and at room temperature were the optimal conditions to further explore the scope of the MCR.
To demonstrate the applicability of the optimised reaction conditions, different secondary amines, aldehydes and boronic acids were employed in this MCR, using 5Dy as catalyst (Fig. 2). The reaction proceeds in very good to excellent yields (84–98%, 17 products) at room temperature with catalyst loading 1.0 mol%. Compound 4aba was characterized via single crystal X-ray crystallography (see Fig. S1†). Very good yields were obtained for the reaction involving indoline as secondary amine (Fig. 2), whereas the reaction with N-methylaniline, and N-benzylmethylamine, gave very good yields, 94 and 93%, respectively (Fig. 2). The presence of an electron donating group in the para position of boronic acid gives the multicomponent product in very good yields, whereas the nature of secondary amine influences the total yield (Fig. 2). Compounds 4cab, 4cea, 4cab and 4ceb (allyl functional group) were isolated in moderate yields. Moreover, the MCRs between salicylic aldehyde or o-vanillin, secondary amines and benzene 1,4-diboronic acid afforded products 4aac, 4bac, 4aec and 4bec in very good yields (Fig. 2). To the best of our knowledge, this is the first time that benzene 1,4-diboronic acid is successfully involved in the titled reaction. It is worth mentioning that the reaction does not proceed with the use of primary amines or benzaldehyde indicating the importance of the hydroxyl group in the aldehyde to activate the boronic acid.31
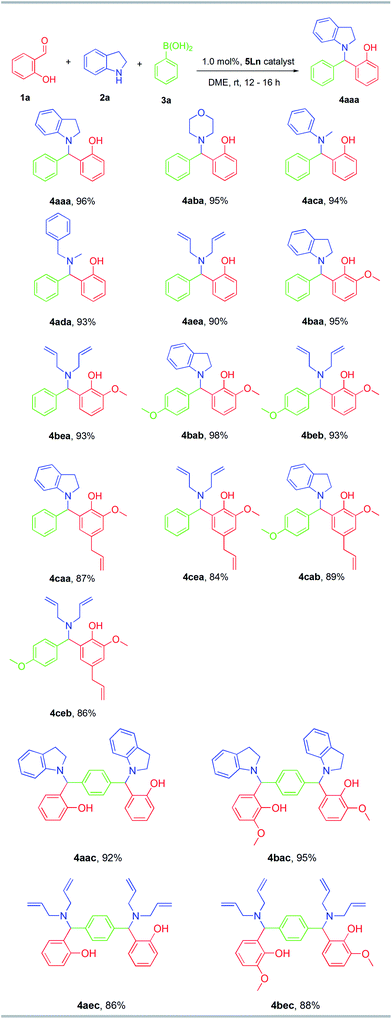 |
| Fig. 2
5Dy catalysed MCR of aldehydes, amines and boronic acids. Reaction conditions; aldehyde, 0.5 mmol; substituted indole, 1 mmol; DME 10 mL; room temperature; 12–16 h. 1.0% loading of 5Dy. | |
Notwithstanding, the MCR with 2-methoxy-benzaldehyde, indoline and boronic acid yields the desired product (as shown by the presence of a peak in the region of 5.30 ppm in the 1H NMR spectra, corresponding to the tetra substituted carbon atom), however all efforts to purify it by column chromatography were not successful.
Altering the 4f centres without changing the core topology in 5Ln, allows the use of a pallet of techniques [NMR (for 5Y), EPR (for 5Gd), UV-Vis] to study in situ a catalytic reaction. In the present case, to gain further information on the plausible mechanism we attempted to monitor the reaction by NMR and UV-Vis. Efforts to monitor the catalytic cycle by NMR, using the diamagnetic 5Y as catalyst, were not successful due to solubility issues. Salicylic aldehyde shows a broad peak in the UV region prohibiting thus a complete study, however according to our previous UV-Vis binding studies, aldehydes prefer coordination to Ln over Zn.15 Having all these in mind, we envision the plausible mechanism shown in Scheme 2. The first step of the cycle involves the coordination of aldehyde to Ln metal and secondary amine to Zn metal centres and giving rise to N-substituted Zn-complex in step 2. Then, the reaction of the boronic acid with N-substituted Zn-complex (step 2) affords the desired final compounds with the release of boronic acid (steps 3–5) with the formation of transition state in step 5.
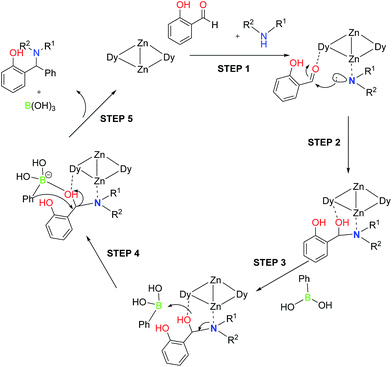 |
| Scheme 2 Proposed reaction mechanism. | |
Conclusions
Compound 5Dy was successfully employed for the first time as catalyst in the PBR MCR (Scheme 1) exhibiting excellent efficacy at room temperature, in short time, with 1% loading and excellent yields. The present successful paradigm adds value on the usefulness of 5Ln CCs as catalysts. These species can efficiently catalyse at room temperature and low loadings, two different type of transformations, Friedel–Crafts alkylation15,16 and the present MCR, thus opening new directions in 3d/4f coordination chemistry. We currently exploring the catalytic efficiency of 5Ln to other MCRs and organic transformations as well to domino reactions. Involvement of chiral ligands for the synthesis of 3d/4f CCs to achieve enantioselectivity and immobilization of 5Ln are also currently under investigation in our laboratory.
Acknowledgements
We thank the EPSRC (UK) for funding (grant number EP/M023834/1, PDRA position for P. K.) and the University of Sussex for offering PhD position to K. G.
References
- C. Papatriantafyllopoulou, E. E. Moushi, G. Christou and A. J. Tasiopoulos, Chem. Soc. Rev., 2016, 45, 1597–1628 RSC.
- X.-J. Kong, Y.-P. Ren, W.-X. Chen, L.-S. Long, Z. Zheng, R.-B. Huang and L.-S. Zheng, Angew. Chem., Int. Ed., 2008, 47, 2398–2401 CrossRef CAS PubMed.
- J.-D. Leng, J.-L. Liu and M.-L. Tong, Chem. Commun., 2012, 48, 5286–5288 RSC.
- E. C. Sañudo and L. Rosado Piquer, Dalton Trans., 2015, 44, 8771–8780 RSC.
- K. Liu, W. Shi and P. Cheng, Coord. Chem. Rev., 2015, 289–290, 74–122 CrossRef CAS.
- J.-L. Liu, Y.-C. Chen, F.-S. Guo and M.-L. Tong, Coord. Chem. Rev., 2014, 281, 26–49 CrossRef CAS.
- G. Guthausen, J. R. Machado, B. Luy, A. Baniodeh, A. K. Powell, S. Krämer, F. Ranzinger, M. P. Herrling, S. Lackner and H. Horn, Dalton Trans., 2015, 44, 5032–5040 RSC.
- J. Long, J. Rouquette, J.-M. Thibaud, R. A. S. Ferreira, L. D. Carlos, B. Donnadieu, V. Vieru, L. F. Chibotaru, L. Konczewicz, J. Haines, Y. Guari and J. Larionova, Angew. Chem., Int. Ed., 2015, 54, 2236–2240 CrossRef CAS PubMed.
- J. Jankolovits, C. M. Andolina, J. W. Kampf, K. N. Raymond and V. L. Pecoraro, Angew. Chem., Int. Ed., 2011, 50, 9660–9664 CrossRef CAS PubMed.
- F. Evangelisti, R. More, F. Hodel, S. Luber and G. R. Patzke, J. Am. Chem. Soc., 2015, 137, 11076–11084 CrossRef CAS PubMed.
- G. Maayan and G. Christou, Inorg. Chem., 2011, 50, 7015–7021 CrossRef CAS PubMed.
- K. Griffiths, V. N. Dokorou, J. Spencer, A. Abdul-Sada, A. Vargas and G. E. Kostakis, CrystEngComm, 2016, 18, 704–713 RSC.
- K. Griffiths, C. W. D. Gallop, A. Abdul-Sada, A. Vargas, O. Navarro and G. E. Kostakis, Chem.–Eur. J., 2015, 21, 6358–6361 CrossRef CAS PubMed.
- K. Griffiths, P. Kumar, J. D. Mattock, A. Abdul-Sada, M. B. Pitak, S. J. Coles, O. Navarro, A. Vargas and G. E. Kostakis, Inorg. Chem., 2016, 55, 6988–6994 CrossRef CAS PubMed.
- K. Griffiths, P. Kumar, G. R. Akien, N. F. Chilton, A. Abdul-Sada, G. J. Tizzard, S. J. Coles and G. E. Kostakis, Chem. Commun., 2016, 52, 7866–7869 RSC.
-
P. Kumar, S. Lymperopoulou, K. Griffiths, S. I. Sampani and G. E. Kostakis, unpublished results.
- B. H. Rotstein, S. Zaretsky, V. Rai and A. K. Yudin, Chem. Rev., 2014, 114, 8323–8359 CrossRef CAS PubMed.
- R. Kakuchi, Angew. Chem., Int. Ed., 2014, 53, 46–48 CrossRef CAS PubMed.
- M. Shiri, Chem. Rev., 2012, 112, 3508–3549 CrossRef CAS PubMed.
- A. Dömling, W. Wang and K. Wang, Chem. Rev., 2012, 112, 3083–3135 CrossRef PubMed.
- E. Ruijter, R. Scheffelaar and R. V. A. Orru, Angew. Chem., Int. Ed., 2011, 50, 6234–6246 CrossRef CAS PubMed.
- N. Isambert, M. D. M. S. Duque, J.-C. Plaquevent, Y. Génisson, J. Rodriguez and T. Constantieux, Chem. Soc. Rev., 2011, 40, 1347–1357 RSC.
- C. Kalinski, M. Umkehrer, L. Weber, J. Kolb, C. Burdack and G. Ross, Mol. Diversity, 2010, 14, 513–522 CrossRef CAS PubMed.
- R. Zhou, Q. Wu, M. Guo, W. Huang, X. He, L. Yang, F. Peng, G. He and B. Han, Chem. Commun., 2015, 51, 13113–13116 RSC.
- M. Varyani, P. K. Khatri and S. L. Jain, Catal. Commun., 2016, 77, 113–117 CrossRef CAS.
- T. Flagstad, M. T. Petersen and T. E. Nielsen, Angew. Chem., Int. Ed., 2015, 54, 8395–8397 CrossRef CAS PubMed.
- S. Roy and O. Reiser, Angew. Chem., Int. Ed., 2012, 51, 4722–4725 CrossRef CAS PubMed.
- I. Ibrahem, P. Breistein and A. Córdova, Chem.–Eur. J., 2012, 18, 5175–5179 CrossRef CAS PubMed.
- N. A. Petasis and I. Akritopoulou, Tetrahedron Lett., 1993, 34, 583–586 CrossRef CAS.
- N. R. Candeias, F. Montalbano, P. M. S. D. Cal and P. M. P. Gois, Chem. Rev., 2010, 110, 6169–6193 CrossRef CAS PubMed.
- N. A. Petasis and S. Boral, Tetrahedron Lett., 2001, 42, 539–542 CrossRef CAS.
- M. L. Deb, S. S. Dey, I. Bento, M. T. Barros and C. D. Maycock, Angew. Chem., Int. Ed., 2013, 52, 9791–9795 CrossRef CAS PubMed.
- M. T. Richers, M. Breugst, A. Y. Platonova, A. Ullrich, A. Dieckmann, K. N. Houk and D. Seidel, J. Am. Chem. Soc., 2014, 136, 6123–6135 CrossRef CAS PubMed.
- Y. Huang and T. Hayashi, J. Am. Chem. Soc., 2015, 137, 7556–7559 CrossRef CAS PubMed.
- Q. Wang and M. G. Finn, Org. Lett., 2000, 2, 4063–4065 CrossRef CAS PubMed.
- N. J. McLean, H. Tye and M. Whittaker, Tetrahedron Lett., 2004, 45, 993–995 CrossRef CAS.
- N. R. Candeias, L. F. Veiros, C. A. M. Afonso and P. M. P. Gois, Eur. J. Org. Chem., 2009, 1859–1863 CrossRef CAS.
- B. R. Prasad Reddy, P. V. Govardhana Reddy, D. P. Kumar, B. N. Reddy and M. V. Shankar, RSC Adv., 2016, 6, 14682–14691 RSC.
- J. Chojnacki, B. Oleksyn and E. Zukowska, Rocz. Chem., 1971, 45, 487 CAS.
- YIII is not a lanthanide, but has similar ionic radii to HoIII and catalytic behaviour to DyIII. Furthermore, the Y derivatives can be studied by 89Y-NMR.
- O. V. Dolomanov, L. J. Bourhis, R. J. Gildea, J. A. K. Howard and H. Puschmann, J. Appl. Crystallogr., 2009, 42, 339–341 CrossRef CAS.
- L. Palatinus and G. Chapuis, J. Appl. Crystallogr., 2007, 40, 786–790 CrossRef CAS.
- G. M. Sheldrick, Acta Crystallogr., Sect. C: Struct. Chem., 2015, 71, 3–8 CrossRef PubMed.
- C. F. Macrae, P. R. Edgington, P. McCabe, E. Pidcock, G. P. Shields, R. Taylor, M. Towler and J. Van De Streek, J. Appl. Crystallogr., 2006, 39, 453–457 CrossRef CAS.
Footnote |
† Electronic supplementary information (ESI) available. CCDC 1486441. For ESI and crystallographic data in CIF or other electronic format see DOI: 10.1039/c6ra17209a |
|
This journal is © The Royal Society of Chemistry 2016 |
Click here to see how this site uses Cookies. View our privacy policy here.