DOI:
10.1039/C6RA11030D
(Paper)
RSC Adv., 2016,
6, 62157-62164
Reversible intercalation of aluminium into vanadium pentoxide xerogel for aqueous rechargeable batteries†
Received
28th April 2016
, Accepted 22nd June 2016
First published on 23rd June 2016
Abstract
Rechargeable batteries based on the intercalation of aluminium ions may be competitive against lithium-ion batteries, but their development and comprehension are full of difficulties. The charge/discharge processes are particularly complex in aqueous electrolyte solutions. The electrochemical behaviour of orthorhombic V2O5, obtained from xerogel, in an aluminium cell is studied here by using electrochemical cycling, impedance spectroscopy, XRD and XPS results. After electrochemical intercalation of aluminium, the resulting (Al3+)x/3[(V4+)x,(V5+)2−x]O5·nH2O is XRD-amorphous at approximately x = 0.5. The reversible capacity is ca. 120 mA h g−1 (equivalent to Al0.27V2O5). The loss of crystallinity induced by the electrochemical intercalation enhances the chemical exchange between the electrode material and the electrolyte solution. In the presence of acidic water solution, besides the faradic electrochemical process driven by the electrical current, aluminium, protons and water also can be intercalated into V2O5 by chemical reactions or ion exchange.
Introduction
Lithium-ion batteries have been successfully commercialized since the 1990s. At present, batteries based on aluminium may be advantageous against lithium batteries in terms of economy, sustainability and energy density.1 Although, lithium and sodium intercalation reactions have been extensively studied over decades, the study of the insertion of multivalent cations has received less attention and it is still in its infancy. Several of the most promising materials proposed for reversible intercalation of aluminium ions are titanium dioxides2–4 and vanadium oxides.5–8
Orthorhombic vanadium pentoxide (V2O5) possesses a layered structure built up from VO5 square pyramids sharing edges and corners, with V2O5 layers held together via weak vanadium–oxygen interaction.9 The layered character of V2O5 and the high redox potential of V5+ make it an interesting and versatile host material for many guest species and for batteries based on intercalation of ions such as lithium, sodium, magnesium and aluminium. It is worth to note that xero-V2O5 adsorbs water at the oxide–air interface and that, according to the layer structure of V2O5, water absorption may be described as an intercalation process.10 The xerogel (xero-V2O5) consists of V2O5 bilayers with water between them and it is a Brønsted acid. Thus, the intercalated water can play an important role in the electrochemistry of V2O5.11–13 In addition, vanadium pentoxide gels are very sensitive to some reduction of vanadium ions (weak mixed V5+–V4+ character) and its content in V4+ is related to the water content (dehydratation leads to some reduction of vanadium). In non-aqueous lithium cell, vanadium pentoxide exchanges its residual water with the organic solvent and probably the discrepancies in the electrochemical behaviour reported by different authors may be due to different water content in their samples.10–15 According to the literature,14,15 the xerogel of V2O5 can contain protonated water (H3O+) in the interlayer space and the layers of vanadium oxide are negatively charged (V2O5m−) with about 0.2 charge per vanadium atom. This fact is due to the acidity of V5+ which drives to hydrolysis. Thus, the xero-V2O5 is a protonic conductor and presents ionic exchange properties between the H3O+ cations and various charged species (Mn+), such as Al3+.14 By immersion of amorphous or poorly crystallized xero-V2O5 in an aqueous solution of cations, the negative charge of the V2O5m− layer remains fixed while the H3O+ ions in the interlayer space are exchanged with the outer cations of the solution. By thermal annealing of the M-intercalated xero-V2O5 materials (Mxn+, (V2O5)m−, yH2O), vanadium bronzes are formed (MxV2O5) in which the initial vanadium is partially reduced (about 600 °C for M = Al).15
Surprisingly, in contrast to lithium and magnesium batteries, the role of water molecules in the aluminium intercalation into V2O5 has received little attention. Within the field of post-lithium batteries, the intercalation of aluminium into TiO2 for batteries using aqueous electrolyte solutions has been explored.2,3 However, to the best of our knowledge, the intercalation of aluminium into vanadium pentoxide for batteries using aqueous electrolyte has not been reported yet; irrespectively of the reports using electrolytes based on ionic liquids.16–18
Experimental
Synthesis of V2O5
The V2O5 sample was prepared by using the ion exchange method. A 0.5 M solution of sodium metavanadate in water was eluted through a proton exchange resin (Downing). The resulting hydrogel was aged and dried in an air atmosphere for two weeks. Amorphous xerogel of vanadium pentoxide is obtained at this stage. Then, the xerogel (xero-V2O5) is manually milled, further dried at 120 °C and crystallized at 300 °C for three hours in air atmosphere.
Characterization of materials
X-ray diffraction (XRD) patterns were recorded in a Bruker D8 instrument with CuKα radiation and reflection geometry. For recording XRD of the recuperated electrodes, the samples were protected against reaction with air by covering with kapton tape.
For the images of electron microscopy, TEM (JEM 1400) and FE-SEM (JSM 7800F) were used.
Thermogravimetric analysis (TGA) was carried out in Shimadzu instrument at 10 °C min−1 of heating rate in air atmosphere.
The chemical state and composition were analyzed by X-ray photoelectron (XP) spectra that were recorded using a SPECS Phobios 150MCD and Mg Kα source. Before recoding the XPS of the electrode recuperated from the electrochemical cell, the electrode material was washed with water to remove the remaining electrolyte solution and then dried under vacuum.
Electrochemical tests
All the electrochemical experiments were performed in a VMP Biologic instrument. The electrochemical cells were of Swagelok-type in T-configuration and, in order to prevent corrosion, the use of metals as current collectors was avoided. The active material (xero-V2O5 annealed at 300 °C, 80%) was mixed with polytetrafluoroethylene (PTFE, 10%) binder and carbon black (conductive additive, 10%), and the resulting mixture was spread on carbon paper (graphite, Goodfellow). The electrolyte solution was 1 M AlCl3 in deionized water which was previously outgassed by flowing Ar. Several discs of Whatman glass fiber filter were used like separator. The counter electrode was glassy carbon. Mercury/mercurous sulfate electrode Hg/Hg2SO4, K2SO4(sat'd) was used as reference electrode (MSE).
Results and discussion
Microstructure
The V2O5 xerogel dried at 120 °C is XRD-amorphous (Fig. 1a). After annealing at 300 °C it shows a XRD-pattern (Fig. 1b) that is ascribed to orthorhombic vanadium pentoxide (layered structure), with space group Pmmn. The resulting lattice parameters are a = 11.521(2) Å, b = 3.563(1) Å and c = 4.3719(6) Å. The relative intensity of the reflection (001) is in good agreement with the layered character and with preferred orientation of the particles. The average crystallite size obtained by applying the Scherrer equation (L = λβ−1
cos
θ−1) to the broadening (β) of the Bragg reflections is L = 45(4) nm.
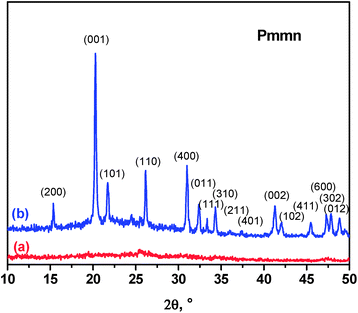 |
| Fig. 1 XRD pattern of xero-V2O5 dried at 120 (a) and 300 °C (b). The Miller indexes are given. | |
The morphology of the particles was examined by SEM and TEM (Fig. 2). In the SEM results the particles of the sample dried at 120 °C have the appearance of crumpled layers, and after annealing at 300 °C the layered particles become less crumpled and more flat. In the TEM micrographs (Fig. 2E and F), the particles show layered character, with around 30–250 nm of diameter.
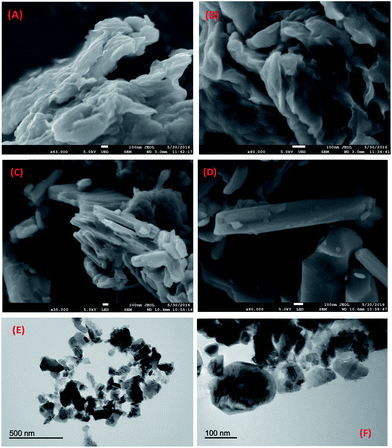 |
| Fig. 2 SEM (A–D) and TEM (E and F) micrographs of xero-V2O5 heated to 120 (A and B) and 300 °C (C–F). | |
Thermogravimetric analysis
The water content of V2O5 can greatly influence on its electrochemical cycling and capacity in batteries.11,12 Water may be detrimental for non-aqueous batteries, but a small amount of water can help to the intercalation of the cation into vanadium oxide. It is possible to obtain the anhydrous form of V2O5 whether the sample is heated for enough time at 250 °C or higher temperature, although the water can be reabsorbed. Fig. 3 shows to the TGA results of a xero-V2O5 sample annealed at 300 °C and stored in air atmosphere for two months. The xero-V2O5 sample contains a small amount of water (V2O5·nH2O). Firstly, weakly bonded or adsorbed water is released at ca. 100 °C. Then, more strongly water is lost at 250–300 °C. If one takes into account all the water content found in the TGA up in all the range of temperature from 30 to 300 °C, then it results that n = 0.29 in V2O5·nH2O. If the water loss at lower temperature is considered to be free or weakly adsorbed water, the content of more strongly bonded water is n = 0.16 (from 100 to 300 °C). According to the resulting spacing of the basal plane obtained from the XRD pattern and the literature,19 the water molecules are not placed in the interlayer spacing. Even after annealing at 600 °C, the XRD pattern of vanadium oxide remains nearly unchanged (not shown), confirming that there was not a significant amount of water in the interlayer space. These results show that xero-V2O5 has tendency to adsorb limited amounts of water from the atmosphere.
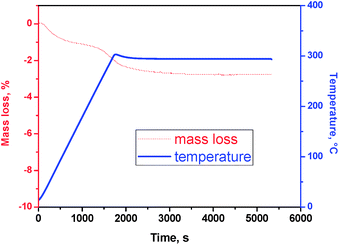 |
| Fig. 3 TGA curve of xero-V2O5 (heated to 300 °C two months before recording the TGA experiment). Firstly, the sample was heated at a rate of 10 °C min−1 up to 300 °C, and then the temperature was hold at 300 °C for one hour. | |
Electrochemical performance
The electrochemical behavior of the as-prepared V2O5 sample was examined using cyclic voltammetry (Fig. 4) and galvanostatic cycling (Fig. 5). In the cyclic voltammogram (CV) of V2O5 in aluminium cell a pair of redox peaks can be observed at −0.30 and −0.03 V (vs. MSE) in the cathodic and anodic sweep, respectively (Fig. 4A). These peaks agree well with a reversible faradic process. Corrosion and electrolyte decomposition are not taking place in the voltage range in which V2O5 is employed.
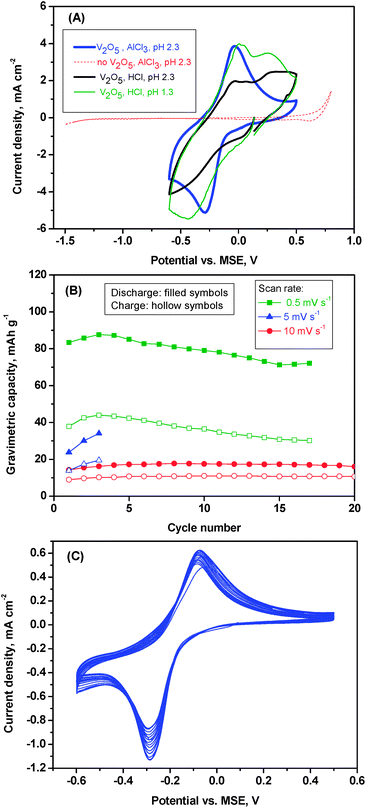 |
| Fig. 4 Cyclic voltammetry results. (A) Comparison of cyclic voltammograms (CV) of V2O5 (working electrode) and blank experiment (no V2O5) using different solutions and at scanning rate of 10 mV s−1. (B) Capacity as a function of cycle number at different scan rates in 1 M AlCl3(aq.) solution (pH 2.3). (C) CV of V2O5 in 1 M AlCl3(aq.) at 0.5 mV s−1. | |
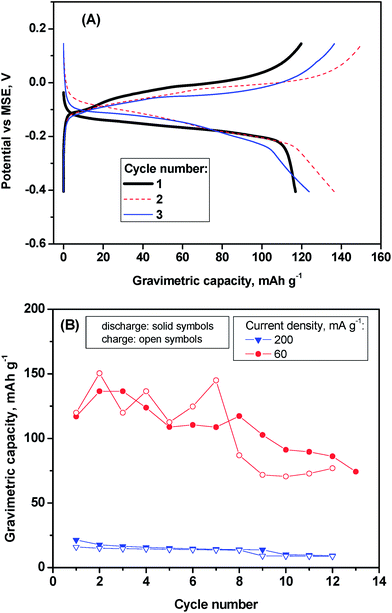 |
| Fig. 5 Galvanostatic experiments. (A) A typical voltage–capacity curve of V2O5 in aluminium aqueous cell at 60 mA g−1 of current density. (B) Specific capacity as a function of cycle number at different current densities. | |
As a blank test, the CV obtained in the absence of active material (the working electrode is a carbon foil) shows no redox activity in the voltage range between −1.3 and +0.5 V. In comparison with anatase,2 the voltage of V2O5 is higher, and this fact can involve higher energy density and that H2 is not evolved during the reduction process. For the sake of comparison, aqueous solutions of AlCl3 an HCl were used as electrolytes.
If one looks at the cyclic voltammetry results of V2O5 in hydrochloric solution (Fig. 4A), it is evident that the protons can play an important role. In solution of aluminium-free hydrochloric acid at pH 1.3 the current becomes more intense than at pH 2.3, strongly suggesting that more protons are inserted at more acidic pH.
The electrochemical intercalation in aqueous solution of protons yielding HxV2O5 (0 < x < 0.5) and the insertion of lithium to form LixV2O5 (0 < x < 1.0) were previously reported by other authors.20 The same authors reported that larger cations are not intercalated into V2O5. Taking into account that the crystal ionic radius of Al3+ (0.68 Å) is smaller than Li+ (0.90 Å), it seems reasonable to believe that aluminium can be intercalated. In this sense, other authors found minimum activation energy for aluminium insertion (by exchange in water solution) into xero-V2O5.21 Thus, in Fig. 4A, for a pH value of 2.3, the CV curve is modified in the presence of aluminium ions in comparison with aluminium-free solution at the same pH, indicating that aluminium insertion is preferential to proton insertion. The redox peaks in 1 M AlCl3(aq.) are resulted from aluminium insertion. In Fig. 4B, it is observed that the capacity is higher at lower scan rates, and the capacity retention is good. In the CV at low rate the peak intensity is smaller (Fig. 4C), as expected for a diffusion-controlled process.
In the galvanostatic experiment, the voltage–capacity curve shows a reversible pseudoplateau at ca. −0.15 V in the discharge process and at ca. 0.0 V in the charge process (Fig. 5A). The origin of this pseudoplateau can be the intercalation process of hydrated aluminium and the coexistence of crystalline and amorphous phase as it is further discussed below in the light of XRD and XPS results. The reversible capacity is substantially lower at higher rates (ca. 20 mA h g−1 at 200 mA g−1 of current density) than at low rate (in the order of 120 mA h g−1 at 60 mA g−1) (Fig. 5B), suggesting that the reaction is controlled by diffusion.
Hydrated aluminium ions are intercalated into the interlayer space of V2O5 and V5+ ions are reduced to V4+ according to the next reaction:
|
x/3Al(H2O)n3+ + (V5+)2O5 + xe− → (Al3+)x/3[(V4+)x,(V5+)2−x]O5·nH2O
| (1) |
Water molecules can shield the charge of the aluminium ion facilitating its insertion/deinsertion into vanadium oxide. Thus, it is expected that water molecules are co-intercalated simultaneously to aluminium-intercalation. On the other hand, it is known that in the interlayer space of V2O5 in contact with water, protonated water (H3O+) and Al3+ can be chemically exchanged.22 In the same sense, other authors found that V2O5 can be doped with aluminium ions (AlxV2O5·nH2O) using soft chemistry.15,23 Thus, chemical exchange can overlap with the electrochemical process that is driven by the flow of electrons through the external electrical circuit during the discharge/charge. There is a competition between chemical exchange and electrochemical insertion/deinsertion of aluminium ions and water molecules (or H3O+). Most probably, some protons are also intercalated during the discharge process. Then, aluminium ions are also chemically inserted into V2O5 by cationic exchange with protons in competition to the electrochemical deinsertion during the charge process, though the ion exchange process is slower than the faradic reaction. It involves that the aluminium content in AlxV2O5, determined from charge passed through the cell, would be overestimated. Therefore, the apparent discharge capacity could be slightly lower than the charge capacity, particularly at low kinetics (60 mA g−1 in Fig. 5B).
For the sake of comparison, the electrochemical intercalation of lithium into V2O5 was explored. The results agree well with the literature and hence the capacity fade upon cycling in Fig. 5 and 6 is due to the dissolution of vanadium compounds.24–26 A discharge capacity around 130–250 mA h g−1 and a charge capacity of only ca. 70 mA h g−1 is observed in (Fig. 6). The operating voltage is lower in the lithium electrolyte than in aluminium one. In contrast to aluminium, lithium is intercalated in non-hydrated form,20 only a fraction of lithium can be deintercalated and the charge capacity is substantially smaller than the discharge capacity (Fig. 6). Some amount of lithium is irreversibly trapped in the framework of V2O5, and the charge of Li+ helps to shield the repulsions between the oxygens in the framework of V2O5. It is worth to note that, in comparison with other ions, such as Li+ and Na+, Al3+ is a smaller ion with a higher hydration number. It is expected that the chemical exchange between the solution and intercalated vanadium oxide is enhanced for aluminium, and that water (or protonated water) helps to shield the repulsions between the oxygens in the framework of AlxV2O5.
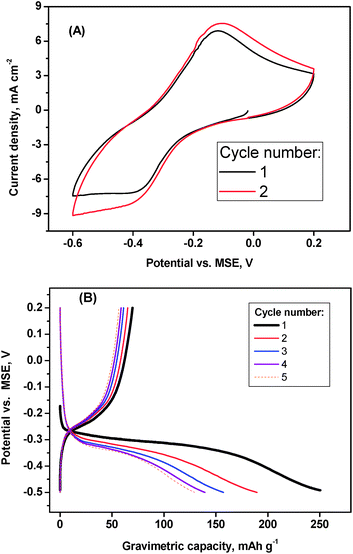 |
| Fig. 6 Electrochemistry of V2O5 in lithium cell: (A) CV at 10 mV s−1 of scan rate and (B) galvanostatic cycling at 20 mA g−1 of current density. Electrolyte: 1 M LiCl in water. | |
Ex situ XRD
According to the ex situ XRD patterns (Fig. 7) of the electrodes recorded at different states of discharge, the structure of V2O5 is initially preserved during the beginning of the discharge process, the position of the Bragg peaks remain nearly unchanged and become more broadened. New Bragg peaks are not observed. It is worth to note that the interlayer spacing in V2O5 is much larger that the ionic size of Al3+. With further discharge depth, the oxide irreversibly becomes XRD-amorphous at the nominal composition Al0.5V2O5 (equivalent to 221 mA h g−1). The amorphous state of Al-intercalated vanadium oxide is strongly indicative of the hydration of the interlayer spacing.10 In other words, aluminium and water molecules are co-intercalated and the resulting hydrated vanadium oxide (Al3+)x/3[(V4+)x,(V5+)2−x]O5·nH2O is XRD-amorphous. Another possible explanation would be that the chemical exchange between intercalated aluminium and protons from the solution in the form of H3O+ provokes the amorphization of the vanadium oxide electrode, but it could not explain alone the high capacity of the charge process. In contrast to the insertion of hydrated aluminium, according to the literature the insertions of lithium and protons preserve the crystalline character of V2O5.20
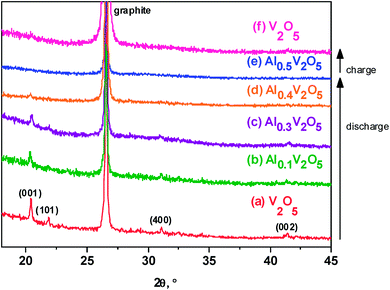 |
| Fig. 7 Ex situ XRD patterns of (a) raw V2O5 and electrodes recuperated from aluminium cells at selected states of discharge (b–e) and charge (f) at slow current density. The (002) reflection of hexagonal graphite (substrate of the electrode active material) is also observed at ca. 26.4°. | |
Chemical and electrochemical intercalation
The redox potential of V5+ is quite high (E°(VO2+/VO2+) = +1.0 V vs. SHE), but this potential in principle would not be enough to decompose water (E°(O2/H2O) = +1.229 V vs. SHE). In contrast to crystalline V2O5, it is known that amorphous or poorly crystallized xero-V2O5 immersed into an aqueous solution containing certain cations can intercalate these cations by easy exchange with the H3O+ ion.15 We tested the stability of crystalline V2O5 in aqueous solution containing Al3+ ions. After immersion of V2O5 in 1 M AlCl3(aq.) solution during two hours, a slight decrease of the pH value (ca. 0.15 units) and formation of tiny bubbles (more probably oxygen) were observed. In addition, the crystalline structure of vanadium oxide is preserved (see XRD in ESI†) and no relevant change of the unit cell parameters was observed. The solution remained colourless. These results suggest that, although V2O5 is not soluble, it may spontaneously suffers a very limited intercalation, proton/aluminium exchange and redox reaction in which vanadium oxide would be slightly reduced and O2 is released: |
V2O5 + xAl(H2O)n3+ + 3x/2H2O → AlxV2O5·nH2O + 3x/4O2 + 3xH+
| (2) |
The positive charge of the chemically intercalated aluminium ion balances the negative charge left in V2O53x− by the electrons released by oxygen.
In the electrochemical cell, the loss of crystallinity that is induced by the electrochemical intercalation of aluminium (reaction (1)) can enhance the extent of the chemical exchange in XRD-amorphous (Al3+)x/3[(V4+)x,(V5+)2−x]O5·nH2O (reaction (2)). The reaction (2) also can explain the apparently higher charge capacity observed in some cycles of the galvanostatic experiments. In the same sense, the reduction of V5+ and formation of O2 was reported by Znaidi et al.14 In addition, Schöllhorn et al. reported the proton-exchange reaction in vanadium bronzes.22
X-ray photoelectron spectroscopy
To further understand the mechanism of aluminium intercalation into V2O5, the X-ray photoelectron spectra of the samples are shown in Fig. 8. For the raw V2O5 sample the main XP lines of O 1s (Fig. 8Aa) and V 2p3/2 (Fig. 8Ba) are placed at the binding energies lines of 530.5 (O2−) and 517.5 eV (V5+), respectively. These values agree very well with the reported data for V2O5.27 A minor component at 516.1 eV is ascribed to traces of V4+. These V4+ ions can increase the electronic conductivity. There are two additional O 1s components with very low intensity and placed at ca. 532 and 534 eV that are ascribed to more covalently bonded oxygen in defects and contamination on the surface of V2O5.27,28
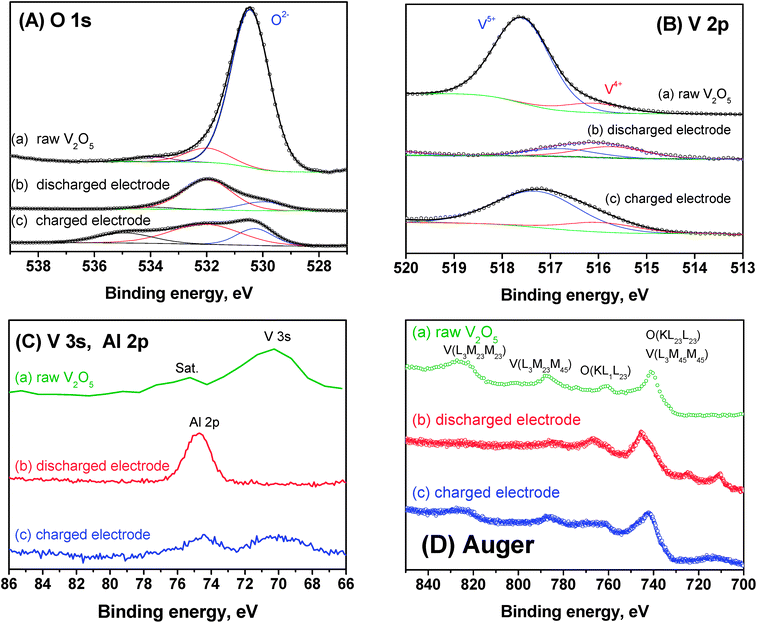 |
| Fig. 8 X-ray photoelectron spectra of (A) O 1s core level and (B) V 2p3/2, (C) Al 2p and V 3s core levels for (a) as prepared V2O5, (b) discharged electrode (end of the first discharge at −0.3 V vs. MSE) and (c) electrode after the first discharge–charge cycle (+0.15 V vs. MSE). | |
After reaction with aluminium, the V 2p line is shifted to lower binding energy as due to the reduction of V5+ to V4+ (Fig. 8Bb), and the O 1s line is shifted to higher energy (Fig. 8Ab). The charge process leads to the re-oxidation of vanadium (Fig. 8Bb). The broadening of XPS in the region of O 1s core peak in the charged electrode (Fig. 8Ac) is in good agreement with a more disordered structure.
In the discharged electrode, the Al 2p line is observed at 74.6 eV (Fig. 8C). The V 3s core hole lines have a binding energy of ca. 70.3 eV and a small satellite at ca. 4–8 eV higher binding energy (Fig. 8C). It has been suggested that the satellite structure of V 3s is caused by core hole screening.29 The satellite observed in raw V2O5 (Fig. 8Ca) is in good agreement with the presence of traces of V4+. According to the literature, in vanadium oxides there is exchange coupling of the 3s electrons and the valence shell (3d electrons), but this exchange does not directly reflect the 3d occupancy.29 In the discharged electrode (Fig. 8Cb), more probably the V 3s signal is too small to be observed.
The Auger transitions are significant to reveal the reversible change of the oxidation state of vanadium (Fig. 8D). The V(L3M4,5M4,5) transition is mixed with the O(KL2,3L2,3) transition, making this Auger peak more intense.27 The V(L3M4,5M4,5) transition is shifted to higher energies for the discharged electrode that contains V4+ (3d1). The peak of the V (L3M2,3M4,5) Auger transition is due not only to the vanadium transition, but also to interatomic transitions between vanadium and oxygen, and such peak has lower intensity for the discharged electrode (Fig. 8Db) as expected according to the literature.27 In V2O5 (V5+, 3d0), the greater covalency leads to a higher density of electrons (coming from oxide ion) around vanadium ions as compared with AlxV2O5.
From the quantitative analysis of the XP spectra, the chemical compositions of the discharged and charged electrodes would be Al5V2O5x5.6H2O and Al0.52V2O5, respectively. These formulas must be regarded with a lot of caution because XPS is a surface technique and the electrode surface can be contaminated with traces of electrolyte, but the results strongly suggest that hydrated aluminium is reversibly intercalated into vanadium pentoxide in good agreement with reaction (1).
Impedance spectra
The electrochemical impedance spectra (Fig. 9) are in good agreement with the diffusion of aluminium ions into the framework of V2O5. The spectrum recorded on the freshly assembled cell, at −0.05 V of open circuit voltage (OCV), shows a linear behaviour with an angle of ca. 45°. Is is characteristic of a system under diffusion control since the kinetics of the charge transfer at the electrode–electrolyte interface is faster than the diffusion of the Al3+ ions in V2O5. After the first discharge at −0.2 V, the resulting spectrum still shows a linear behaviour. After 10 cycles, a depressed semicircle is observed at high–medium frequencies, which is ascribed to charge transfer resistance and double-layer capacitance. The detailed view of the high–medium frequencies region is shown in Fig. 9B. The linear part at medium–low frequencies is ascribed to Warburg impedance associated with diffusion of aluminium in V2O5. The small size of the semicircle shows that the charge transfer resistance is low, and according to the fitting using the equivalent circuit shown as an inset in Fig. 9B, the resistance values is R2 = 18 Ω.
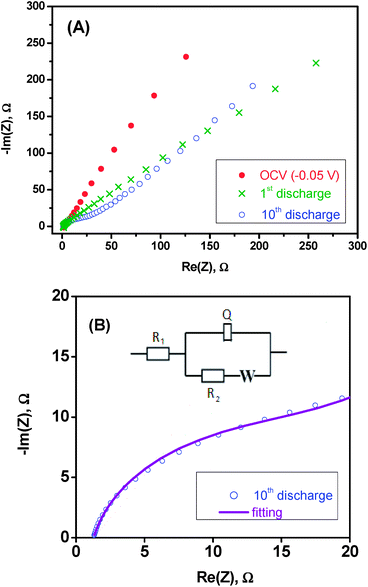 |
| Fig. 9 (A) Impedance spectra (Nyquist plots) of V2O5 electrode measured at OCV and at the end of the first discharge (−0.3 V vs. MSE) and after ten cycles. (B) A detailed view of the high–medium frequencies region after ten cycles and equivalent circuit. W = Warburg impedance. R = resistance. Q = constant phase element. | |
Conclusions
Vanadium pentoxide has been used as an active electrode material in rechargeable aluminium battery based on aqueous electrolyte. These batteries can be environmentally friendly and more sustainable in comparison with lithium batteries. The intercalation/deintercalation process of aluminium is complex. During the discharge process, vanadium is reduced and the resulting product becomes XRD-amorphous at Al0.5V2O5, in good agreement with bulk intercalation. As due to the high charge-to-size ratio of Al3+ and the chemical exchange properties, the electrochemical (faradic) intercalation of aluminium into V2O5 is parallel to water intercalation, and some protons also can be intercalated. During the charge (oxidation) process the chemical intercalation and exchange of protons and aluminium can compete against the faradic deintercalation process. These features should be taken into account for the future development of batteries based on intercalation of multivalent ions. Perhaps, further optimization of the electrolyte composition, the selection of another binder and the formation of composite materials may improve the coulombic efficiency and cycling stability.
Acknowledgements
The authors would like to greatly acknowledge MINECO, FEDER (MAT2014-56470-R). The instruments for XPS, SEM and TEM were provided by SCAI (UCO Central Service for Research Support). We also thank Institute of Fine Chemistry and Nanochemistry and European Research Institute ERI-ALISTORE.
References
- M. C. Lin, M. Gong, B. Lu, Y. Wu, D. Y. Wang, M. Guan, M. Angel, C. Chen, J. Yang, B. J. Hwang and H. Dai, Nature, 2015, 520, 324 CrossRef CAS PubMed; V. Rani, V. Kanakaiah, T. Dadmal, M. S. Rao and S. Bhavanarushi, J. Electrochem. Soc., 2013, 160, A1781 CrossRef; R. Mori, RSC Adv., 2013, 3, 11547 RSC; R. Mori, RSC Adv., 2014, 4, 1982 RSC.
- S. Liu, J. J. Hu, N. F. Yan, G. L. Pan, G. R. Li and X. P. Gao, Energy Environ. Sci., 2012, 5, 9743 Search PubMed.
- Y. J. He, J. F. Peng, W. Chu, Y. Z. Li and D. G. Tong, J. Mater. Chem. A, 2014, 2, 1721 RSC.
- S. Sang, Y. Liu, W. Zhong, K. Liu, H. Liu and Q. Wu, Electrochim. Acta, 2016, 187, 92 CrossRef CAS.
- H. Wang, Y. Bai, S. Chen, X. Luo, C. Wu, F. Wu, J. Lu and K. Amine, ACS Appl. Mater. Interfaces, 2015, 7, 80 Search PubMed.
- N. Jayaprakash, S. K. Das and L. A. Archer, Chem. Commun., 2011, 47, 12610 RSC.
- W. Wang, B. Jiang, W. Xiong, H. Sun, Z. Lin, L. Hu, J. Tu, J. Hou, H. Zhu and S. Jiao, Sci. Rep., 2013, 3, 3383 CrossRef PubMed.
- D. B. Le, S. Passerini, F. Coustier, J. Guo, T. Soderstrom, B. B. Owens and W. H. Smyrl, Chem. Mater., 1998, 10, 682 CrossRef CAS.
- R. Enjalbert and J. Galy, Acta Crystallogr., Sect. C: Cryst. Struct. Commun., 1986, 42, 1467 CrossRef.
- J. Livage, Chem. Mater., 1991, 3, 578 CrossRef CAS; H. H. Kristoffersen and H. Metiu, J. Phys. Chem. C, 2016, 120, 3986 CrossRef.
- J. Wang, C. J. Curtis, D. L. Schulz and J. G. Zhang, J. Electrochem. Soc., 2004, 151, A1 CrossRef CAS.
- Y. Wang, H. Shang, T. Chou and G. Cao, J. Phys. Chem. B, 2005, 109, 11361 CrossRef CAS PubMed.
- M. Vujković, I. Pašti, I. Stojković Simatovića, B. Šljukić, M. Milenković and S. Mentus, Solid State Ion., 2015, 176, 130 Search PubMed.
- L. Znaidi, N. Baffier and M. Huber, Mater. Res. Bull., 1989, 24, 1501 CrossRef CAS.
- N. Baffier, L. Znaidi and M. Huber, Mater. Res. Bull., 1990, 25, 705 CrossRef CAS.
- N. Jayaprakash, S. K. Das and L. A. Archer, Chem. Commun., 2011, 47, 12610 RSC.
- L. D. Reed and E. Menke, J. Electrochem. Soc., 2013, 160, A915 CrossRef CAS.
- M. Chiku, H. Takeda, S. Matsumura, E. Higuchi and H. Inoue, ACS Appl. Mater. Interfaces, 2015, 7, 24385 Search PubMed.
- P. Aldebert, N. Baffier, N. Gharbi and J. Livage, Mater. Res. Bull., 1981, 16, 669 CrossRef CAS.
- P. G. Dickens, A.
M. Chippindale and S. J. Hibble, Solid State lon., 1989, 34, 79 CrossRef CAS.
- J. C. Badot and N. Baffier, J. Mater. Chem., 1992, 2, 1167 RSC.
- R. Schöllhorn, F. Klein-Reesink and R. Reimold, J. Chem. Soc., Chem. Commun., 1979, 9, 398 RSC.
- K. Zhu, H. Qiu, Y. Zhang, D. Zhang, G. Chen and Y. Wei, ChemSusChem, 2015, 8, 1017 CrossRef CAS PubMed.
- I. Stojkovic, N. Cvjeticanin, I. Pašti, M. Mitric and S. Mentus, Electrochem. Commun., 2009, 11, 1512 CrossRef CAS.
- M. Vujković, I. Pašti, I. Stojković Simatović, B. Šljukić, M. Milenković and S. Mentus, Electrochim. Acta, 2015, 176, 130 CrossRef.
- H. Wang, Y. Zeng, K. Huang, S. Liu and L. Chen, Electrochim. Acta, 2007, 52, 5102 CrossRef CAS.
- Q. H. Wu, A. Thißen and W. Jaegermann, Appl. Surf. Sci., 2005, 252, 1801 CrossRef CAS; G. Silversmit, D. Depla, H. Poelman, G. B. Marin and R. De Gryse, Surf. Sci., 2006, 600, 3512 CrossRef; A. Benayad, H. Martinez, A. Gies, B. Pecquenard, A. Levasseur and D. Gonbeau, J. Electron Spectrosc. Relat. Phenom., 2006, 150, 1 CrossRef; A. Meisel, K. H. Hallmeier, R. Szargan, J. Muller and W. Schneider, Phys. Scr., 1990, 41, 513 CrossRef; J. Kasperkiewicz, J. A. Kovacich and D. Lichtman, J. Electron Spectrosc. Relat. Phenom., 1983, 32, 123 CrossRef; P. Mezentzeff, Y. Lifshitz and J. W. Rabalais, Nucl. Instrum. Methods Phys. Res., Sect. B, 1990, 44, 296 CrossRef.
- A. Thissen, D. Ensling, F. J. Fernández-Madrigal, W. Jaegermann, R. Alcántara, P. Lavela and J. L. Tirado, Chem. Mater., 2005, 17, 5202 CrossRef CAS.
- P. Krüger, M. Taguchi, J. C. Parlebas and A. Kotani, Phys. Rev. B: Condens. Matter Mater. Phys., 1997, 55, 16466 CrossRef CAS; R. Zimmermann, R. Claessen, F. Reinert, P. Steiner and S. Hüfner, J. Phys.: Condens. Matter, 1998, 10, 5697 CrossRef.
Footnote |
† Electronic supplementary information (ESI) available: XRD results. See DOI: 10.1039/c6ra11030d |
|
This journal is © The Royal Society of Chemistry 2016 |
Click here to see how this site uses Cookies. View our privacy policy here.