DOI:
10.1039/C6RA09599B
(Paper)
RSC Adv., 2016,
6, 54463-54470
The combination of adsorption by functionalized halloysite nanotubes and encapsulation by polyelectrolyte coatings for sustained drug delivery
Received
13th April 2016
, Accepted 27th May 2016
First published on 27th May 2016
Abstract
A novel organic–inorganic hybrid nanocomposite was established for the sustained release of an analgesic. Adsorption by functionalized halloysite nanotubes (HNTs) and encapsulation by polyelectrolyte coatings (PECs) via the layer-by-layer (LBL) self-assembly of chitosan (CHI) and sodium alginate (ALG) were combined for the proposed drug delivery. In order to increase loading capacity, HNT lumen enlargement and γ-aminopropyltriethoxysilane (APTES) modification were introduced to manufacture nanocontainers denoted as AHNTs. Ibuprofen (C13H18O2, IBU) is an active analgesic that was selected as a model drug to evaluate the pharmaceutical properties. Fourier transform infrared spectroscopy, transmission electron microscopy, thermal gravimetric analysis, zeta potential, and high-performance liquid chromatography were performed to characterize the structure and properties of ALG/CHI PECs on AHNTs loaded with IBU (IBU-AHNTs@PECs). The release behavior was investigated by changing the PEC and pH conditions. An enhanced capacity for adsorption (32%) and a sustained-release performance (115 h) of IBU-AHNTs@PECs toward IBU were demonstrated, which followed the power law kinetic model. The proposed combination is expected to provide an important strategy to regulate drug delivery and extend pharmaceutical applications.
1 Introduction
Various drug delivery systems, such as emulsions,1 hydrogels,2 liposomes,3 nanofibers,4 and micro/nanoparticles,5 have shown great potential in sustained drug delivery. These systems are generally formulated from numerous types of materials, including macromolecular compounds,6 polymers,7 and other organic and inorganic substances.8,9 Consequently, regulating drug release and targeting the drug to specific tissue can be performed favourably. Especially, the layer-by-layer (LBL) self-assembly method has currently attracted considerable scientific interest because of its potential applications in drug delivery.
LBL self-assembly technique is a powerful tool for polymer matrix encapsulation.10 Polyelectrolyte coatings (PECs) with controlled thickness and composition are elaborated on various particles through stepwise deposition, which is mainly caused by the electrostatic attraction between oppositely charged polyelectrolytes. Biological macromolecules,11 drug molecules,12 and some active ingredients13 can be successfully encapsulated through this technique, thereby resulting in expected release characteristics. Natural polyelectrolyte pair, which comprises chitosan (CHI) and sodium alginate (ALG),14 has been widely investigated for drug delivery systems because of environmental friendliness, biodegradability, and biocompatibility. Shiqu Ye and coworkers prepared hollow CHI/ALG microcapsules through LBL assembly and confirmed the new loading process and release properties of insulin.15 Yang et al. also used two polyelectrolyte pairs, namely, sodium polystyrene sulfonate/polycation poly(allylamine hydrochloride) and ALG/CHI, to encapsulate drug-loaded mesoporous silica nanotubes and obtained two different types of drug delivery systems.16 A targeted drug delivery system based on single-wall carbon nanotubes and coated with a polysaccharide material could be loaded with the anticancer drug doxorubicin.17 An oral sustained drug release pattern was also achieved when felodipine-loaded mesoporous silica nanosphere was coated with CHI and acacia via LBL self-assembly method.18 Nevertheless, drug storage capacity, drug burst release, and weak stability are the frequently encountered problems for these polymer matrices. In this situation, preparing the smart inorganic–organic hybrid nanocomposites was an excellent choice to make drug administration simple and efficient.
Organic–inorganic hybrid nanocomposites are currently developed as the remarkable drug delivery system because of their biological stability and controlled release property.19,20 The environmental friendliness and good biocompatibility of halloysite nanotubes (HNTs) render them excellent scaffolds for developing new organic–inorganic hybrid nanocomposites. HNTs, with a chemical formula of Al2Si2O5(OH)4·nH2O, are a type of natural aluminosilicate clay mineral.21,22 The chemical structure is similar to that of kaolin. However, HNTs feature a dominantly hollow tubular structure. The HNT length varies in the range 0.2–1.5 mm, whereas the inner tube diameter ranges from 10 nm to 20 nm. In HNTs, layers are separated by single sheets of water molecules and the interlayer ions can be available for exchange. Therefore, HNTs have a larger ion exchange capacity and higher reactivity than kaolinite.23 These characteristics make them attractive candidates for biological and non-biological applications. In addition, their gibbsite octahedral sheet aluminum (innermost) and silicate (outermost) surfaces allow different chemical functionalization onto the internal and external surfaces.24,25 For instance, selective modification of HNTs by immobilizing functional groups has been successfully applied in new applications, such as molecular storage,26,27 molecular separation,28,29 and molecular delivery.30,31 HNTs are also promising drug vehicles in drug delivery system to realize the optimal encapsulation and control of drug administration. Biocompatibility and nontoxicity, which are revealed in vitro cell toxicity tests,32,33 are the main prerequisites for safe usage of HNTs in medical products. Notably, inorganic/organic hybrid nanocomposites based on HNTs have already been evaluated as potential delivery system for nucleic acids,34 proteins,35,36 and drug molecules.37,38 Previous studies demonstrated that the combination of inorganic skeleton and organic polymers is a feasible approach to prepare nanocomposites for sustained drug delivery.
Inspired by preliminary work, the present study focused on fabrication of the novel inorganic–organic hybrid nanocomposites based on HNTs. Functionalized HNTs were proposed as the vehicles for drug delivery. The PECs of different thicknesses were encapsulated to regulate the release behavior, and eventually obtained an efficient sustained drug delivery system. Accordingly, ibuprofen (IBU) was selected as a model drug because its properties were well documented, and it was widely used as an anti-inflammatory drug.39 Primarily, HNT lumen enlargement and γ-aminopropyltriethoxysilane (APTES) functionalization were introduced to enhance loading,40 and the resultant product was named AHNTs. PECs, composed of CHI and ALG, were fabricated through stepwise adsorption of oppositely charged polyelectrolytes basing on LBL self-assembly method. The resulting nanocomposite was denoted as IBU-AHNTs@PECs, which were characterized through Fourier transform infrared spectrometer (FT-IR), transmission electron microscopy (TEM), thermal gravimetric analysis (TGA), and zeta-potential analysis. The loading capacity and sustained-release behavior of IBU were also systematically investigated.
2 Experimental
2.1 Materials
HNTs were supplied by Zhengzhou Jinyangguang Co., Ltd. (Henan, china); 3-aminopropyltriethoxysilane (APTES) was purchased from Aladdin Company (Shanghai, China); chitosan (viscosity 100–200 mpa s for a 2 mg mL−1 solution in 0.5 M NaCl at pH 5.0, Shanghai, China), and sodium alginate (low viscosity for a 2 mg mL−1 solution in 0.5 M NaCl at pH 5.0, Alfa Aescar, China) were obtained from Sigma-Aldrich company Ltd; ibuprofen (IBU) was purchased from Sinopharm Chemical Reagent Co., Ltd. (Shanghai, China). Concentrated sulfuric acid (H2SO4) was purchased from Central South University. All chemicals were of analytical reagent grade, except that methanol was of high performance liquid chromatography (HPLC) grade. Deionized water was used for all the experiments and tests.
2.2 Apparatus and conditions
The morphology and size distribution of products were obtained at 20.0 kV on a JEM-2100F TEM (JEOL, Japan). The chemical composition analysis of IBU-AHNTs@PECs was obtained from energy dispersive X-ray (EDX). Fourier transform infrared (FT-IR) spectroscopy (Nicolet, USA) was employed to obtain the infrared spectra. The samples were prepared by mixing the products with KBr, and pressing them into a compact pellet. UV-2450 Spectrophotometer (Shimadzu, Japan) was employed to determine concentration. For scanning electron microscopy (SEM, MIRA3 TESCAN, FEI) observation, the sample suspension was dropped on a glass slide, air dried, and then sputtered with gold. Zeta-potential of the materials after each adsorption was measured in 0.5 M NaCl solution with a pH value of 5.0 by a Zeta-sizer (Mastersizer 2000, UK). Each datum was averaged from 3 measurements. The thermogravimetric analysis (TGA) of samples was measured using a Diamond TG/DTA instruments (STA 449C Jupiter, Netzsch, Germany) under an argon atmosphere up to 600 °C with a heating rate of 10 °C min−1. HPLC (Unimicro Technologies Co., Ltd., Shanghai, China) with a 2010A LC liquid delivery pump and a 2010A UV detector was used to determine the retention time of IBU using the C18 column (4.6 × 150 mm). The mobile phase was methanol–water–acetic acid (75
:
25
:
0.1, v/v/v) at a flow rate of 1.0 mL min−1.
2.3 Halloysite etching by sulfuric acid
Enlarged HNTs (EHNTs) were obtained by selective etching of aluminum oxide according to the following procedures. Crude HNTs were sieved and then dried at 100 °C in an oven. The HNTs (8.0 g) were briefly suspended in 400 mL of sulfuric acid solution at a certain concentration (2 mol L−1). The suspension was magnetically stirred for 12 h at a controlled temperature of 70 °C. The resulting reaction mixture was repeatedly eluted with distilled water until the pH was in the range 6–7. The products were dried in a vacuum at 50 °C.
2.4 Halloysite functionalization and IBU loading
AHNTs were prepared as follows. APTES (2.5 mL) was dissolved in 25 mL of dry toluene. Approximately 0.7 g of EHNT powder was added, and the suspension was dispersed ultrasonically for 30 min. Evacuation treatment was performed in this process. The suspension was then refluxed at 120 °C for 24 h under constant stirring. A calcium chloride drying tube was used to maintain a dry environment. The resultant was separated via centrifugation, extensively washed several times with fresh dry toluene to remove the excess APTES, and then dried overnight at 70 °C.
IBU was loaded into AHNTs according to the following approach. An appropriate amount of AHNTs was mixed with ethanol solution of IBU (50 mg mL−1) under continuous stirring to form a suspension. After sonication, the suspension was transferred into a vacuum jar, which was then evacuated with a vacuum pump. The fizzing of the suspension indicated that air was being removed from the lumen of the AHNTs and being replaced with IBU solution. The mixture was maintained under vacuum for 5 h and was then cycled back to atmospheric pressure. This process was repeated five times to maximize the loading efficiency. Afterward, the resultant was washed, collected, and dried in vacuum at 60 °C.
2.5 Preparation of IBU-AHNTs@PECs nanocomposites
The encapsulation by PECs that consisted of polycation CHI and polyanion ALG was performed via LBL self-assembly. Solutions of CHI (2 mg mL−1, pH 3.0) and ALG (2 mg mL−1, pH 5.0) were obtained by separately dissolving them in 0.5 M NaCl solution. The IBU-AHNTs were first modified with APTES properly before assembly. In a typical fabrication process, ALG solution was added to the suspension of IBU-AHNTs (2.0 wt%, 25 mL) and stirred for 2.5 h at room temperature. The resulting particles were recovered via repeated centrifugation/washing/redispersion cycles with dilute aqueous NaCl before the next adsorption. Subsequently, the oppositely charged CHI solution was added, stirred for 2.5 h, and then centrifuged, washed, and redispersed. The process for generating the first ALG/CHI coatings was repeated three times with CHI as the outermost layer. The prepared samples were dried in a vacuum at 60 °C in a vacuum for further characterization.
2.6 Determination of drug loading capacity
A classical method was used to evaluate the amount of IBU adsorbed into AHNTs. The IBU-AHNTs (0.05 g) were immersed in 50 mL of simulated intestinal fluid (pH 7.4) for 24 h until swelling equilibrium. Afterward, IBU-AHNTs were ultrasonicated at 50 °C for 2 h to ensure the complete release of IBU from the nanotubes. The released IBU from the nanotubes was separated from the mixture through centrifugation. The IBU concentration was determined with a UV-vis spectrophotometer at 264 nm. Drug loading (%) was calculated with the following equation: |
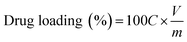 | (1) |
where C is the concentration of IBU in the supernatant (g mL−1), V is the volume of the supernatant (mL), and m is the mass of IBU-AHNTs (g).
2.7 In vitro IBU cumulative release studies
The in vitro release test was conducted on a dissolution test apparatus (RC806 Tianjin TDTF Technology Co., Ltd., China) with eight rotating paddles. Approximately 0.5 g of IBU-AHNTs@PECs were sealed in a dialysis bag and soaked in 500 mL of dissolution medium at 37 ± 0.5 °C with a rotation speed of 100 rpm. At certain time intervals, 5.0 mL of the dissolution medium was withdrawn, and equivalent volumes of fresh medium were added back to maintain a constant volume. The collected solution was passed through a 0.45 μm nylon membrane filter, and analyzed with a UV-vis spectrophotometer at a wavelength of 264 nm. Each dissolution test was performed in triplicate. The cumulative IBU released was determined with eqn (2), as follows: |
 | (2) |
where L and Rt represent the amount of IBU loaded and the cumulative amount of IBU released at time t, respectively.
3 Results and discussion
3.1 Preparation of IBU-AHNTs@PECs
The fabricating procedure of IBU-AHNTs@PECs was illustrated in Fig. 1. IBU was a safe nonsteroidal anti-inflammatory drug that was selected as a model drug. A new combination approach to increase loading capacity was introduced. Then, the PECs were formed by alternate adsorption of oppositely charged polyelectrolyte pair, beginning with the deposition of the negatively charged ALG. In the sustained release system, the ALG/CHI coatings could cap the channels, which obviously assuaged the initial burst release and regulated the release behavior.
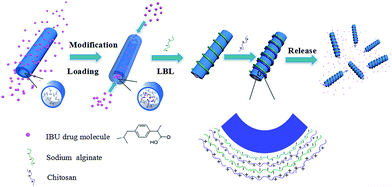 |
| Fig. 1 Schematic representation of IBU loading, modification, and drug-release process. | |
3.2 FT-IR analysis
Fig. 2 presented the FT-IR spectra of IBU (a), HNTs (b), EHNTs (c), AHNTs (d), IBU-AHNTs (e), and IBU-AHNTs@PECs (f). As shown in Fig. 2c, the absorption bands at 3693 and 3622 cm−1 were attributed to the stretching modes of hydroxyl groups for the HNTs. The characteristic peak of the inner hydroxyl group was observed around 912 cm−1. Interlayer water was indicated by the bending vibration at 1633 cm−1. The successful APTES functionalization was evidenced by the absorption, such as the stretching CH2 vibration band around 2926 cm−1 and the deformation CH2 vibration at 1492 cm−1 (Fig. 2d). Furthermore, the broad peak of water OH stretch, centered at 3450 cm−1, was further widened in the spectra of AHNTs samples, which was attributed to the overlap with the NH2 stretching vibration signal around 3360 cm−1. All of these observations showed the successful functionalization of AHNTs. As observed from IBU-AHNTs (Fig. 2e), the typical absorption peaks of IBU at 2955, 2871, 2923, and 1717 cm−1 demonstrated the successful entrapment of IBU in the products. Fig. 2f showed that the strong bands that appeared at around 1620 and 1417 cm−1 were assigned to antisymmetric and symmetric COO stretching vibrations of ALG, respectively. The band at 1524 cm−1 that corresponded to N–H bending vibrations was found, which proved the successful deposition of CHI. The presence of the band at 1559 cm−1 was also expected given the electrostatic interaction between CHI and ALG.
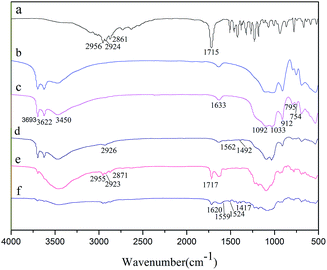 |
| Fig. 2 FT-IR spectra of IBU (a), HNTs (b), EHNTs (c), AHNTs (d), IBU-AHNTs (e), and IBU-AHNTs@PECs (f). | |
3.3 SEM and EDX analyses
Direct visualization of HNTs (a) and IBU-AHNTs@PECs (b) were depicted in Fig. 3 by SEM images. The natural HNTs contained many cylindrical-shaped nanotubes and a small amount of tiny tubes adhering to the surface (Fig. 3a). Compared with original HNTs, it showed that the walls of products appeared to be rougher and thicker, and the edges changed obscure and blurred in Fig. 3b. These characteristic were caused by the bridging of polyelectrolyte CHI and ALG on the surface during the alternating deposition.
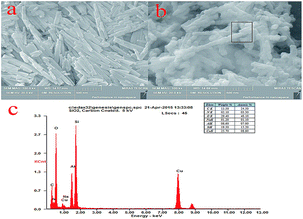 |
| Fig. 3 SEM photos of HNTs (a), IBU-AHNTs@PECs (b), and EDX spectra of the IBU-AHNTs@PECs (c). | |
In addition, the surface chemical composition of the IBU-AHNTs@PECs nanocomposite was analyzed using EDX (Fig. 3c), which was collected from the area containing tiny particles and nanocomposite (marked with a black square). The Cu signals in EDX spectra were coming from the copper screen, which was used as the carrier. The peaks of Si, Al, and O could be observed clearly, which were attributed to the characteristic elements of crude HNTs. Given that C, N, O, and particularly Na were the characteristic elements of PECs, the expected compositions were detected. Therefore, this additional presence indicated a successful functionalization, which corresponded well with other spectroscopic analyses.
3.4 TEM analysis
The morphological features of HNTs, IBU-AHNTs, and IBU-AHNTs@PECs were visualized through TEM (Fig. 4a–c). Fig. 4a showed that the original HNTs had cylindrical-shaped tubular morphology and open-ended lumen, with an inner diameter of 10–20 nm. The products still retained the tubular morphology as the as-prepared nanotubes after enlargement treatment, drug loading, and encapsulation by PECs. Apparent changes could be observed from the TEM micrograph of IBU-AHNTs (Fig. 4b) compared with that of HNTs (Fig. 4a). The hollow tubular structure of HNTs became less resolved because of containing a large number of IBU molecules. As shown in the inset of Fig. 4b, the HNT lumen enlarged to 40–50 nm after acidic treatment. Fig. 4b and c also showed comparative TEM images from nanotubes obtained before and after modifying PECs. A film with a light gray color was located on the outer surface of IBU-AHNTs, which provided direct evidence for the deposition of ALG/CHI coatings. The results indicated that IBU-AHNTs and IBU-AHNTs@PECs were successfully prepared.
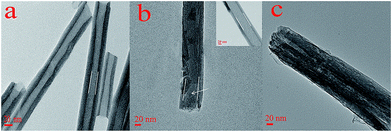 |
| Fig. 4 TEM images of HNTs (a), IBU-AHNTs (b), and IBU-AHNTs@PECs (c). | |
3.5 Zeta-potential analysis
ALG and CHI were alternately deposited on the AHNTs through the LBL self-assembly method to reduce the release rate of the loaded IBU. The encapsulation process was monitored by measuring the zeta-potential, as shown in Fig. 5. An initial value of approximately 22.7 mV corresponded to as-prepared nanotubes before deposition. The zeta-potential of the nanotubes deposited with the first ALG layer decreased by approximately 35 mV as compared with the bare nanotubes. When the first CHI was deposited, the zeta-potential value increased again by approximately 18 mV. After the second cycle of the assembly process, the regular charge reversal of the zeta-potential proved the successful deposition of polyanion ALG and polycation CHI on IBU-AHNTs alternatingly.
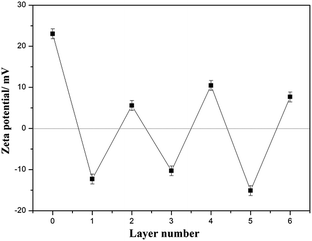 |
| Fig. 5 Zeta-potentials as a function of polyelectrolyte coatings number on IBU-AHNTs alternately deposited with ALG and CHI. | |
3.6 TGA analysis
As presented in Fig. 6, the thermal stability and degradation behavior of the HNTs (a), AHNTs (b), IBU-AHNTs (c), and IBU-AHNTs@PECs (d) were investigated using TGA under nitrogen flow. In the HNTs curves, the weight loss below 150 °C corresponded to desorption of the physically adsorbed water. One major weight loss was resolved in the temperature range of 470–550 °C, which was assigned to the dehydroxylation of the residual structural Al–OH group. In Fig. 6b, the TGA curve for HNTs modified with APTES was reported for comparison. The weight loss from approximately 250 to 460 °C was well resolved, which resulted from the decomposition of aminopropyl groups grafted to the HNTs. For the IBU-AHNT system, another rapid weight loss (about 29%) occurred between 150 and 250 °C, which demonstrated that the IBU storage capacity of the IBU-AHNT system. In the IBU-AHNT@PECs system, the equally apparent weight loss was attributed to the removal of IBU from the IBU-AHNT@PECs system, while the other weight losses occurred with the volatilization of the ALG/CHI coatings.
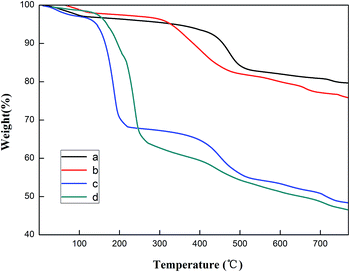 |
| Fig. 6 TGA curves of HNTs (a), AHNTs (b), IBU-AHNTs (c), and IBU-AHNTs@PECs (d). | |
3.7 HPLC analysis
IBU-AHNTs@PECs were immersed in ethanol for 24 h to prove that encapsulation by ALG/CHI PECs had no influence on IBU structure, and the solution was analyzed by HPLC. Fig. 7 showed that the retention time of the standard addition IBU (Fig. 7b) and that of IBU released from the nanocomposite (Fig. 7a) were consistent. This result suggested that IBU was stable during the encapsulation process without any chemical deterioration.
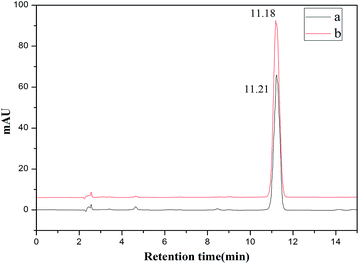 |
| Fig. 7 HPLC chromatograms of IBU released from the IBU-AHNTs@PECs (a), and pure IBU (b). | |
3.8 Loading of IBU in AHNTs
HNTs were promising nanoscale containers for IBU. Simple strategies were explored in this work to enhance the limited drug loading capacity. As shown in Fig. 8, the IBU loading capacity of AHNTs was considerably greater than that of the unmodified HNTs and EHNTs, which demonstrated the effectiveness of promoting loading capacity. APTES modification could promote IBU loading by creating an electrostatic attraction between APTES and IBU, which was stronger than the hydrogen bonding in the case of IBU loading in original HNTs. While, occupation of some of the lumen spaces of HNTs, where IBU was mainly loaded, by APTES oligomers, which could restrict the loading to some extent, was nearly inevitable. In this situation, one possibility to obtain greater loading capacity was to provide additional free lumen spaces. Therefore, the combination of HNT lumen enlargement and APTES functionalization was a potentially versatile method to optimize drug loading.
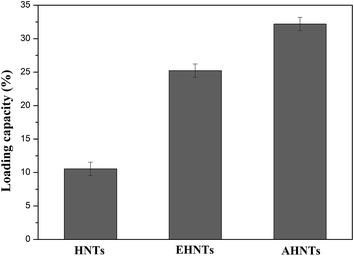 |
| Fig. 8 The loading capacity of the HNTs after modification. | |
3.9 Release studies of nanocomposites
3.9.1 Release studies of IBU-AHNTs@PECs with different number of coatings. The release profiles of IBU-AHNTs@PECs with various numbers of PECs in pH 7.4 PBS were shown in Fig. 9. IBU-AHNTs showed a relatively fast-release profile, and more than 80% of IBU were released within 30 h. This phenomenon could be explained by the dispersing effect and the hydrophilicity of HNTs, as well as the good solubility of IBU in this medium. In contrast to the almost release of IBU from IBU-AHNTs without modifying the PECs, the release of IBU from IBU-AHNTs@PECs exhibited a remarkable sustained-release pattern at pH 7.4. Less than 25% of IBU could be released from IBU-AHNTs@PEC3 over a period of 30 h. This finding primarily indicated the good storage and blocking effect of the PECs. In particular, the ALG/CHI coatings were compact, which also gave rise to an extra barrier to the molecular movement. Thus, the entrapped IBU could hardly be in contact with the PBS and dissolve in the medium favorably. The strong affinity between IBU and AHNTs was another significant factor for the sustained-release behavior. The release rate also decreased as the number of PECs increased. In conclusion, the IBU-AHNTs@PECs system possessed a considerable sustained-release property.
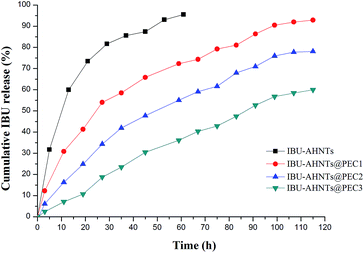 |
| Fig. 9 Release profiles of IBU from the IBUAHNTs@PEC with various number of polyelectrolyte coatings in pH 7.4 PBS. | |
3.9.2 In vitro pH-response study. pH is a key factor that influences the drug delivery. The pH is ∼1.2–2.0 in the stomach, ∼7.0 in the intestine, and as high as 8.0 in the distal part. The IBU release from the IBU-AHNTs@PEC2 was investigated at pH 7.4, 5.0, and 1.2 to study further the influence of pH on the release behavior (Fig. 10).
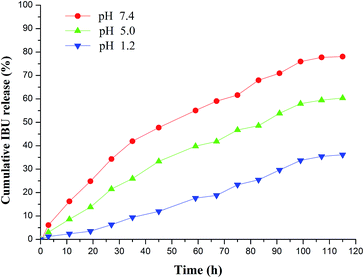 |
| Fig. 10 Release profiles of IBU from IBU-AHNTs@PEC2 at pH 7.4, 5.0, and 1.2 PBS. | |
Significant differences were observed in the cumulative release of IBU from IBU-AHNTs@PEC2 in different pH solutions. The cumulative release of IBU decreased with decreasing pH from 7.4 to 1.2. The delivery system exhibited nearly 45% release at pH 7.4 within 40 h, but <10% release at pH 1.2 over 40 h. However, the release level was maintained in pH 5.0 PBS, with the final total reaching 60%. In particular, the IBU-AHNTs@PECs system could achieve a pH-responsive sustained-release pattern by changing the medium.
These significant findings on the release of IBU could mainly be due to the influence of different pH values on the states of the PECs. At pH 7.4, the amino groups of CHI were mostly unionized, whereas the carboxylate groups on ALG were mainly ionized. In this case, counterions would be attracted into the coatings to compensate for the net excess charge of ALG, which led to an increased osmotic pressure. Subsequently, water molecules would diffuse into the nanocomposite for drug release. This architecture, together with electrostatic repulsion between IBU molecules and ALG layers, would facilitate the permeation of IBU from IBU-AHNTs@PECs at pH 7.4. Nevertheless, the decrease in pH from 7.4 to 5.0 would result in progressive ionization of amino groups on CHI. Thus, the presence of both the amino and the carboxyl groups could inevitably enhance the electrostatic interaction in the ALG/CHI coatings, which would obviously lead to poor permeability. As a result, the drug release rate was restricted to some extent. When the pH value went down to 1.2, the correlation between ALG and CHI, as well as that between IBU and IBU-AHNTs@PECs, would continuously develop and hinder the release of IBU. The different solubility of IBU at different pH values was another crucial factor for the difference in drug release behavior. IBU exhibited limited solubility in acidic solution, but it was readily soluble in alkaline solution. These results demonstrated that polyelectrolyte functionalization made pH an external trigger for the sustained release from IBU-AHNTs@PECs.
3.9.3 Statistical analysis. The release profiles were evaluated with the kinetic theoretical models to study further the release behavior and release mechanism of IBU from IBU-AHNTs@PECs. For calculations, we use the following equations: |
First-order equation Ft = 1 − e−k1t
| (4) |
|
Higuchi's model Ft = kHt1/2
| (5) |
In these models, Ft is the drug release fraction at time “t”; kp, k1, and kH are the release constants of the respective equations; t is the release time; and n is the diffusion exponent, which is a characteristic of the release mechanism.
The release profiles of IBU from IBU-AHNTs@PECs were fitted using the above models. The correlation coefficient (R2) and the release parameter values were also presented in Table 1. Table 1 showed that the first-order value of R2 toward the release from IBU-AHNTs was higher than that of the other models. Therefore, the first-order model fitted best for the IBU-AHNTs. This finding indicated that the drug release rate depended on its concentration.
Table 1 Parameters of the three fitting models of IBU-AHNTs@PECs with various number of polyelectrolyte coatings
Samples |
Power law |
First order |
Higuchi's model |
kp |
n |
R2 |
k1 |
R2 |
kH |
R2 |
IBU-AHNTs |
0.230 |
0.357 |
0.937 |
0.062 |
0.986 |
0.136 |
0.848 |
IBU-AHNTs@PEC1 |
0.112 |
0.452 |
0.987 |
0.024 |
0.979 |
0.091 |
0.982 |
IBU-AHNTs@PEC2 |
0.044 |
0.615 |
0.993 |
0.014 |
0.995 |
0.073 |
0.974 |
IBU-AHNTs@PEC3 |
0.012 |
0.814 |
0.994 |
0.008 |
0.995 |
0.051 |
0.898 |
The first-order release constant (k1) of IBU-AHNTs (0.062) was higher than that of the others, which indicated a rapid release. Furthermore, the release of IBU from IBU-AHNTs@PECs could be well evaluated with the power law kinetic model based on the high values of R2 (>0.99). The n value was in the range 0.452–0.841, which revealed a drug release mechanism for anomalous transport. Accordingly, the strong affinity and polyelectrolyte functionalization slowed down the IBU release and changed the release mechanism.
The releases of IBU from the IBU-AHNTs@PEC2 at different pH were estimated (Table 2). The R2 values of the first order were 0.997 and 0.994 for pH 5.0 and pH 7.4, respectively. The result suggested that the first order fitted well to the release mechanism at pH 5.0 and pH 7.4, whereas at pH 1.2, the release followed the power law model best. The difference in k values also indicated the pH effect on the IBU release behavior.
Table 2 Parameters of fitting models of IBU-AHNTs@PEC2 at different pH dissolution medium
Samples IBU-AHNTs@PEC2 |
Power law |
First order |
Higuchi's model |
kp |
n |
R2 |
k1 |
R2 |
kH |
R2 |
pH 1.2 |
0.001 |
1.201 |
0.994 |
0.004 |
0.968 |
0.027 |
0.782 |
pH 5.0 |
0.018 |
0.753 |
0.993 |
0.009 |
0.997 |
0.053 |
0.929 |
pH 7.4 |
0.044 |
0.615 |
0.993 |
0.014 |
0.995 |
0.073 |
0.974 |
4 Conclusions
In this study, an innovative organic–inorganic hybrid nanocomposite (IBU-AHNTs@PECs) was constructed successfully for sustained release. In particular, AHNTs were synthesized and used as drug vehicles to enhance loading capacity, which involved the enlargement of HNT lumen and APTES functionalization. A favorable ALG/CHI polyelectrolyte pair was encapsulated on IBU-AHNTs via LBL self-assembly technology to slow down the drug release rate. Therefore, a sustained delivery system for IBU was successfully achieved, and the pH was demonstrated to be an external trigger for the IBU release behavior. Additionally, the release profiles could be well described by power law kinetic model. On the basis of these results, we speculate that the combination of AHNTs and LBL self-assembly technology will widen the pharmaceutical applications of inorganic materials and organic polymers.
Acknowledgements
The authors gratefully acknowledge the financial support from the National Natural Science Foundation of China (21576295 and 21276283).
References
- L. Tian, M. P. Prabhakaran, X. Ding, D. Kai and S. Ramakrishna, J. Mater. Sci., 2012, 47, 3272–3281 CrossRef CAS.
- L. Fan, J. Zhang and A. Wang, J. Mater. Chem. B, 2013, 1, 6261–6270 RSC.
- J. V. Natarajan, C. Nugraha, X. W. Ng and S. Venkatraman, J. Controlled Release, 2014, 193, 122–138 CrossRef CAS PubMed.
- R. Qi, R. Guo, M. Shen, X. Cao, L. Zhang, J. Xu, J. Yu and X. Shi, J. Mater. Chem., 2010, 20, 10622–10629 RSC.
- S. Farzaneh, E. Asadi, M. Abdouss, A. Barghi-Lish, S. Azodi-Deilami, H. A. Khonakdar and M. Gharghabi, RSC Adv., 2015, 5, 9154–9166 RSC.
- E. Abdullayev and Y. Lvov, J. Mater. Chem., 2010, 20, 6681–6687 RSC.
- P. Bawa, V. Pillay, Y. E. Choonara and L. C. du Toit, Biomed. Mater., 2009, 4, 022001 CrossRef PubMed.
- L. Fan, B. Li, Q. Wang, A. Wang and J. Zhang, Adv. Mater. Interfaces, 2014, 1, 1300136 Search PubMed.
- S. Zhong, Y. Kong, L. Zhou, C. Zhou, X. Zhang and Y. Wang, J. Chromatogr. B: Anal. Technol. Biomed. Life Sci., 2014, 945, 39–45 CrossRef PubMed.
- E. Donath, G. B. Sukhorukov, F. Caruso, S. A. Davis and H. Möhwald, Angew. Chem., Int. Ed., 1998, 37, 2201–2205 CrossRef.
- Y. Yu, M. Yin, K. Müllen and W. Knoll, J. Mater. Chem., 2012, 22, 7880–7886 RSC.
- C. Wang, C. He, Z. Tong, X. Liu, B. Ren and F. Zeng, Int. J. Pharm., 2006, 308, 160–167 CrossRef CAS PubMed.
- J. Su, X. Tao, H. Xu and J.-F. Chen, Polymer, 2007, 48, 7598–7603 CrossRef CAS.
- C. Peng, Q. Zhao and C. Gao, Colloids Surf., A, 2010, 353, 132–139 CrossRef CAS.
- S. Ye, C. Wang, X. Liu, Z. Tong, B. Ren and F. Zeng, J. Controlled Release, 2006, 112, 79–87 CrossRef CAS PubMed.
- Y.-J. Yang, X. Tao, Q. Hou, Y. Ma, X.-L. Chen and J.-F. Chen, Acta Biomater., 2010, 6, 3092–3100 CrossRef CAS PubMed.
- X. Zhang, L. Meng, Q. Lu, Z. Fei and P. J. Dyson, Biomaterials, 2009, 30, 6041–6047 CrossRef CAS PubMed.
- L. Hu, H. Sun, Q. Zhao, N. Han, L. Bai, Y. Wang, T. Jiang and S. Wang, Mater. Sci. Eng., C, 2015, 47, 313–324 CrossRef CAS PubMed.
- S. Kango, S. Kalia, A. Celli, J. Njuguna, Y. Habibi and R. Kumar, Prog. Polym. Sci., 2013, 38, 1232–1261 CrossRef CAS.
- H. Li, X. Zhu, H. Zhou and S. Zhong, Colloids Surf., A, 2015, 487, 154–161 CrossRef CAS.
- W. O. Yah, H. Xu, H. Soejima, W. Ma, Y. Lvov and A. Takahara, J. Am. Chem. Soc., 2012, 134, 12134–12137 CrossRef CAS PubMed.
- Y. Zhang, J. Pan, Y. Yan, W. Shi and L. Yu, RSC Adv., 2014, 4, 23797–23806 RSC.
- M. H. Shamsi and K. E. Geckeler, Nanotechnology, 2008, 19, 075604 CrossRef PubMed.
- W. O. Yah, A. Takahara and Y. M. Lvov, J. Am. Chem. Soc., 2012, 134, 1853–1859 CrossRef CAS PubMed.
- H. Hang, C. Li, J. Pan, L. Li, J. Dai, X. Dai, P. Yu and Y. Feng, J. Sep. Sci., 2013, 36, 3285–3294 CAS.
- G. Cavallaro, G. Lazzara, S. Milioto, G. Palmisano and F. Parisi, J. Colloid Interface Sci., 2014, 417, 66–71 CrossRef CAS PubMed.
- D. Fix, D. V. Andreeva, Y. M. Lvov, D. G. Shchukin and H. Möhwald, Adv. Funct. Mater., 2009, 19, 1720–1727 CrossRef CAS.
- S. Zhong, C. Zhou, X. Zhang, H. Zhou, H. Li, X. Zhu and Y. Wang, J. Hazard. Mater., 2014, 276, 58–65 CrossRef CAS PubMed.
- C. Zhou, H. Li, H. Zhou, H. Wang, P. Yang and S. Zhong, J. Sep. Sci., 2015, 38, 1365–1371 CrossRef CAS PubMed.
- Q. Wang, J. Zhang, B. Mu, L. Fan and A. Wang, Carbohydr. Polym., 2014, 102, 877–883 CrossRef CAS PubMed.
- P. Yuan, P. D. Southon, Z. Liu and C. J. Kepert, Nanotechnology, 2012, 23, 375705 CrossRef PubMed.
- V. Vergaro, E. Abdullayev, R. Cingolani, Y. Lvov and S. Leporatti, Biomacromolecules, 2010, 11, 820–828 CrossRef CAS PubMed.
- N. G. Veerabadran, D. Mongayt, V. Torchilin, R. R. Price and Y. M. Lvov, Macromol. Rapid Commun., 2009, 30, 99–103 CrossRef CAS PubMed.
- Y.-F. Shi, Z. Tian, Y. Zhang, H.-B. Shen and N.-Q. Jia, Nanoscale Res. Lett., 2011, 6, 1–7 Search PubMed.
- X. Zhu, H. Li, H. Zhou and S. Zhong, RSC Adv., 2015, 5, 66147–66154 RSC.
- C. Chao, J. Liu, J. Wang, Y. Zhang, B. Zhang, Y. Zhang, X. Xiang and R. Chen, ACS Appl. Mater. Interfaces, 2013, 5, 10559–10564 CAS.
- J. Dai, X. Wei, Z. Cao, Z. Zhou, P. Yu, J. Pan, T. Zou, C. Li and Y. Yan, RSC Adv., 2014, 4, 7967–7978 RSC.
- E. Abdullayev and Y. Lvov, J. Mater. Chem. B, 2013, 1, 2894–2903 RSC.
- L. Zhou, Y. Kong, S. Zhong and X. Zhou, J. Sol-Gel Sci. Technol., 2013, 66, 59–67 CrossRef CAS.
- D. Tan, P. Yuan, F. Annabi-Bergaya, H. Yu, D. Liu, H. Liu and H. He, Microporous Mesoporous Mater., 2013, 179, 89–98 CrossRef CAS.
|
This journal is © The Royal Society of Chemistry 2016 |