DOI:
10.1039/C6RA09234A
(Paper)
RSC Adv., 2016,
6, 56647-56655
Effects of alkali and ammonium ions in the detection of poly(ethyleneglycol) by alpha-hemolysin nanopore sensor†
Received
10th April 2016
, Accepted 7th June 2016
First published on 7th June 2016
Abstract
Nanopores have emerged as molecule detectors and more studies are necessary to clarify the analyte–nanopore interaction. Here, we report the influence of cations of the Hofmeister series on a simple bimolecular complexation reaction between poly(ethyleneglycol) (PEG) and α-hemolysin (αHL) nanopore at the single-molecule level. The kinetic constants (on-rate and off-rate) of interaction of PEG molecules with αHL nanopore depend strongly on the type of cation. In the case of on-rate constant, the difference reaches several dozen times. As a consequence of this, both transition rate and detection limit of the nanopore-based sensor were changed. An inverse relationship was demonstrated for the off-rate constant and the solubility of PEG. Except lithium, the other alkali metal cations significantly affect the interaction of PEG with the nanopore and, similarly to potassium, they also bind to this polymer. Thus, the PEG/αHL nanopore interaction can be strongly influenced by the type of cation. The cation-induced specific effect on polymer detection with a single αHL nanopore appears to occur by competition of the cation and PEG by water. Finally, our results represent a guide to select the appropriate physicochemical conditions that enhance the analyte–nanopore interaction, thereby improving the sensitivity of nanopore-based sensors.
Introduction
In the late 19th century, Franz Hofmeister began systematic studies into specific ion effects.1 In these studies, employing salts with a common anion or cation, it was observed that some salts have a particular ability to precipitate proteins in aqueous solution.2,3 Such findings originated the Hofmeister series which is a ranking of the physical properties of ions in aqueous solutions.4 In this series ions can be classified into two groups: kosmotropes (structure makers) and chaotropes (structure breakers), according to their strength of hydration.5,6 The typical order of the ions from the Hofmeister series is generally for anions: SO42− > OH− > F− > Cl− > Br− > NO3− > I− > SCN− > ClO4−, and for cations: NH4+ > K+ > Na+ > Cs+ > Li+ > Rb+ > Mg2+ > Ca2+ > Ba2+.7 Interestingly, there is not a constant sequence of ions. The experimental type and conditions, electrostatic and non-electrostatic interactions can alter the Hofmeister sequence.8 For example, the effectiveness of anions to induce protein crystallization depends on the net charge of the protein; acidic proteins obey the Hofmeister series and the anion order to basic proteins is reversed.9 Additionally, the Hofmeister ions effects depend on salt concentration,10 the cloud-point temperature of the positively charged macromolecules exhibits a direct Hofmeister series at high salt concentration and an inverse Hofmeister series at low salt concentration.10,11
Since its initial discovery involving the study of protein precipitation about over century ago,1,2 the Hofmeister series has been shown to be observed in a wide range of phenomena not only related to protein chemistry but also to colloids, surfaces, membranes, and polymer chemistry.12–14 What, at a molecular level, causes the Hofmeister series? Numerous reports have been published to address this question. However, a molecular-level comprehension of the Hofmeister series is still lacking. The hypothesis proposed so far seem to fall into the following two categories. The first one relates the specific ion effect with the different influences of ions on water structure.14,15 However, there is growing the number of observations that bulk water structure is not responsible for phenomena related to the Hofmeister series.16–18 The second hypothesis suggests that the Hofmeister effects are the result of the preferential interactions ion-solute.3,19,20 Despite of that the salting-out effect has been found to be a very general phenomenon there are few systematic studies about the specific ion effects on the molecule interaction in confined spaces as protein nanopores.21–24 One of the first studies about the influence of ions on protein nanopore was performed by Grigorjev and Bezrukov in 1994.21 They investigated the halide anions (F−, Cl−, Br−, I−) effects on biophysics properties of the roflamycoin ion channels and established both conductance and ionic selectivity depend of halide type used in the bath solution. Protein nanopores have played an important role in the stochastic sensing technology.25,26 Stochastic sensing is an approach that relies on the observation of individual binding events between analyte molecules and a single receptor.25 An important example is the protein nanopore formed by α-hemolysin of Staphylococcus aureus (αHL). The αHL is a pore-forming toxin, its monomers assemble in a lipid membrane forming a heptameric nanopore.25 The αHL nanopore provides a highly confined space which it allows the detection of divalent ions,27,28 enantiomers,29,30 various types of organic molecules,31–35 peptides,36–38 proteins39–41 and DNA.42–45
In our previous studies,24,46,47 we have used a simple system composed of αHL nanopore in a planar lipid bilayer and poly(ethyleneglycol) (PEG) as a molecular probe. Initially, we investigated how the KCl salt concentration could affect the nanopore sensitivity.46 We demonstrated that the increase in KCl salt concentration from 1 M to 4 M improves the nanopore sensitivity and consequently refines analyte identification. Posteriorly, we performed studies about the effects of halogen anions on the kinetics of PEG/αHL nanopore interaction.24,47 We established that the type of anion strongly influences all kinetics constants of PEG/αHL interactions. This system proves to be helpful and allowed us to elucidate details about the ion effects in confined systems as the αHL nanopore.24,47 Thus, in the present study, we investigated the effects of alkali and ammonium ions in the PEG/αHL nanopore interaction.
Experimental
Materials and methods
The wild type of S. aureus α-hemolysin was purchased from Calbiochem (Madison, WI). 1,2-Diphytanoyl-sn-glycero-3-phosphocholine (DPhPC) was purchased from Avanti Polar Lipids (Alabaster, AL). The high quality (>99.99%) of salts (LiCl, NaCl, KCl, RbCl, CsCl, and NH4Cl) was purchased from Sigma (St. Louis, MO, USA). Monodisperse poly(ethylene glycol), PEG1294, was purchased from Polypure (Oslo, Norway). 2-Amino-2-hydroxymethyl-1,3-propanediol (TRIS) and citric acid was from Schwarz/Mann Biotech (Cleveland, OH) and Fluka (Buchs, Switzerland), respectively. All chemicals and solvents were of analytical-grade and used as received, without further purification. High-purity water was obtained after a Milli-Q plus (Billerica, USA) treatment. Solvent-free planar bilayer lipid membranes, with capacitance of 40 pF, were formed by the lipid monolayer apposition technique,48 using DPhPC in hexane (J. T. Baker, Phillipsburg, NJ) at 25 ± 1 °C. As elevated KCl concentrations considerably improve single molecule identifications by unitary protein nanopores46 in the experiment presented herein the membrane-bathing aqueous solutions contained 4 M of alkali- or ammonium-chloride, 5 mM TRIS–citric acid, pH 7.5. Single αHL nanopore incorporation and the measurements of parameters related to the molecular signature (mean duration and amplitude of the blockage), transition rate and kinetic constants of the PEG–nanopore interaction were done essentially as described elsewhere.24 αHL is the protein most frequently used to create nanopore for the development of stochastic biosensing elements. In our study αHL was added from the “cis” side of the membrane in concentration sufficient to form a unitary protein nanopore in planar lipid bilayer. We have used monodisperse PEG1294 as a representative of analyte and it was added to the “trans” compartment of the experimental chamber. Experiments were done using an Axopatch 200B amplifier (Axon Instruments, Foster City, CA) in voltage clamp mode. Membrane potential was maintained using Ag/AgCl electrodes in 3 M KCl 2% agarose bridges assembled within standard 200 μL pipette tips. Currents were filtered by a low-pass eight-pole Bessel filter (Model 9002, Frequency Devices, Haverhill, MA) at 15 kHz and directly saved into the computer memory with a sampling frequency of 100–250 kHz. The limit of PEG solubility was estimated by the modified cloud-point method as described before.46 The inaccuracy was less than 1%.
Results and discussion
αHL nanopore conductance in 4 M chloride solutions
The use of any experimental system demands knowledge of its basic properties. Thus, we started our study investigating the conductance/voltage dependence of αHL nanopore in the presence of 4 M solutions of different alkali chlorides and ammonium chloride. The voltage dependence of the nanopore conductance and of the current rectification was characterized for each salt type. Results are shown in Fig. 1a. All the curves are asymmetric with respect to the applied voltage, and the conductances are in the range 1.5–4 nS. The conductance of αHL nanopore is voltage dependent and increases continuously from −200 to 200 mV. Such behaviour results from the asymmetry in charge distribution between the channel openings and in the channel structure itself, which leads to different contributions of cation and anion to the current at positive and negative voltages.49,50 The conductance of αHL nanopore was the lowest in the presence of LiCl. Using KCl, RbCl, CsCl and NH4Cl we observed very similar large values of conductance for the channel, and in solution of NaCl the conductance presented intermediate values. However, the detailed analysis showed that the nanopore conductance (NH4+ > K+ > Cs+ ≥ Rb+ > Na+ > Li+) does not rank with the lyotropic (Li+ > Na+ > K+ > NH4+ > Rb+ > Cs+) order.51 The different sequences observed above may occur, probably, due to the different types of intermolecular interactions involving the system cation/solvent/cosolute.52
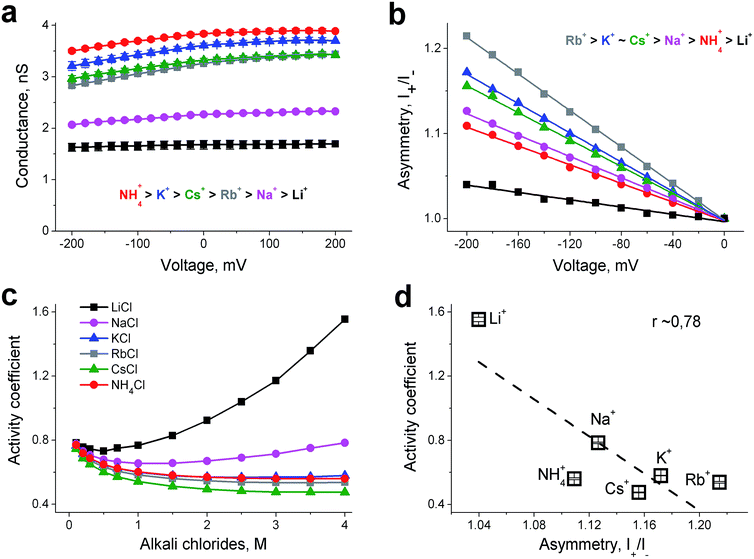 |
| Fig. 1 Voltage-dependent conductance (G–V curve) of αHL nanopore (a) and the asymmetry factor (I+)/(I−) (b) measured using 4 M solution of Li+ (black squares), Na+ (pink circles), K+ (blue triangles), Rb+ (gray squares), Cs+ (green triangles) and NH4+ (red circles) chloride. Activity coefficient of alkali- and ammonium chlorides as a function of its concentration (c) and asymmetry of G–V curves (d). Values of the activity coefficients were taken from the literature.53–58 Dashed line is a first-order regression for the data. Correlation coefficient is shown in figure. Lines were drawn to guide the eye. First-order regression was used to fit the data and the standard deviations were omitted for clarity. | |
An intrinsic feature of majority protein ion channels is their ability to rectify the ionic current and the αHL nanopore is one of its. The rectification ability of this nanopore has been the subject of extensive experimental and theoretical studies.49,50,59,60 Following these studies, we defined the current asymmetry as (I+)/(I−), where I+ and I− are the absolute values of the current at positive and negative voltages, respectively. Fig. 1b shows that the asymmetry is largest at large voltages and decreases to 1 in the limit V ∼ 0. For the LiCl electrolyte, the asymmetry factor is below 1.04 (at 200 mV), whereas it can rise to 1.2 for the RbCl electrolyte. Hence, the current rectification does not follow the lyotropic order also. Therefore, the rectifying property of the channel is modified in a nontrivial way when the type of cations is changed. We found that the asymmetry ranks as: Rb+ > K+ ∼ Cs+ > Na+ > NH4+ > Li+. As it was shown elsewhere49,50,61 the asymmetry is decreased according to KCl concentration (and conductivity of the solution) due charge screening in the nanopore wall. Thus, how the solution with lowest conductivity (LiCl) is managed to screen the charge more effectively than the solutions of other electrolytes with larger conductivities? Trying to solve the puzzle we have inspected how the properties of the alkali chloride solution change with concentration. Activity coefficient attracts our attention because it changes considerably with the ionic concentration in a nonlinear way (Fig. 1c). As a result, we have found that the activity coefficient for LiCl was several times larger than all other salts used at high 4 M concentration. It means that ions in 4 M LiCl are much more independent (uncombined) and available to screen charge at the nanopore wall than others. It created expectation to explain our asymmetry data via activity of electrolytes. The direct confronts these data with our asymmetry results is shown in Fig. 1d. Actually, the reasonably good correlation between these two parameters was found that confirming the expectation. Additionally, Bhattacharya and collaborators49 also observed that the current rectification of αHL nanopore depends on the type of cation and it was weakest in the presence of Li+. By molecular dynamics (MD) simulations, they found that there is a differential interaction of cations to the charged residues of αHL nanopore, which modulates the current properties. In the case of LiCl, Li+ ions bind to negatively charged groups localized inside of “trans” side of nanopore, shielding electrically this region.
Integral effect of PEG at αHL nanopore conductance: voltage dependence
Fig. 2 demonstrates the influence of several concentrations of PEG at the nanopore conductance in alkali- and ammonium chloride solutions. The maximal suppression of PEG-induced on the αHL nanopore conductance was always observed at 40 ± 10 mV (except for LiCl and NH4Cl). It could be seen that the αHL nanopore conductance suppression is dose-dependent of PEG concentration and that the type of cation performs considerable influence on it. Very small effect was observed when the bath solution was composed from LiCl or NH4Cl. The induced PEG conductance suppression was not very large in the presence of NaCl. On the other hand, the strong decrease in the nanopore conductance was established for KCl, RbCl and CsCl (Fig. 2). Thus the effect of monovalent ions is not only voltage- and dose-dependent, but strongly depends on type of cation. The results extend our knowledge about the specific ion influence in confined spaces indicating that not only anions,22,24 but cations possess ability to modify interaction of polymer with protein nanopores considerably.
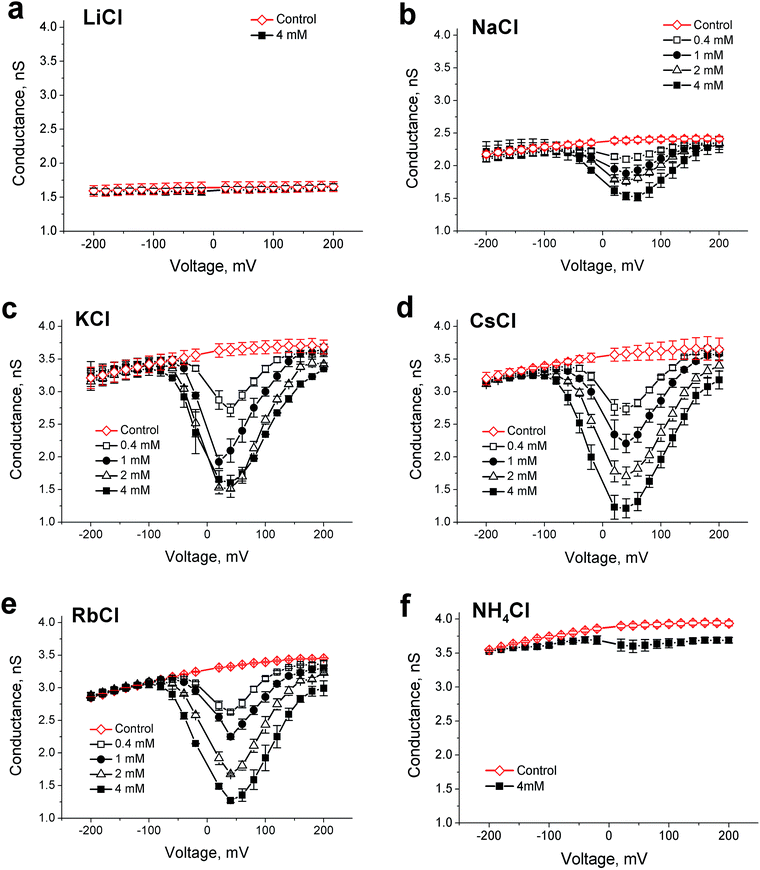 |
| Fig. 2 Effect of trans-side addition of PEG1294 to membrane-bathing solutions on nanopore conductance. PEG concentrations and type of salt are shown in figure. The result of at least three independent experiments (mean ± SE) is presented in each case. | |
Single molecule events: appearance and frequency
Typical high resolution recordings in the presence of PEG are shown in Fig. 3. A large ionic current flows through a single αHL nanopore when its aqueous lumen is free of PEG. In the presence of PEG, the ion current through the single αHL nanopore exhibits stepwise transitions between completely open and partially blocked states. Each decrease and subsequent increase in the ionic current corresponds to a single PEG molecule entering and exiting the pore, respectively. The amplitude, frequency, and duration of these transient steps depend on the electrolyte type. The all-points histograms of the ionic current (Fig. 3 right) demonstrate that level of PEG induced current blockage is practically the same (72 ± 3%) for NaCl, KCl, CsCl and RbCl while it is considerably smaller in the presence of NH4Cl and LiCl (∼66 ± 2% and ∼58 ± 3%, respectively). Other considerable difference in respect to the PEG influence on the current passing through αHL nanopore is the frequency of the events. Looking at the records, (Fig. 3) it could be easily perceived that the frequency is very small when the bath solution was consisted of LiCl, larger in NH4Cl solution, increased further in NaCl and is highest in the presence of KCl, CsCl or RbCl. The frequency ranks at 40 mV as: Cs+ > K+ ∼ Rb+ > Na+ > NH4+ > Li+ with the difference in more than two orders of magnitude.
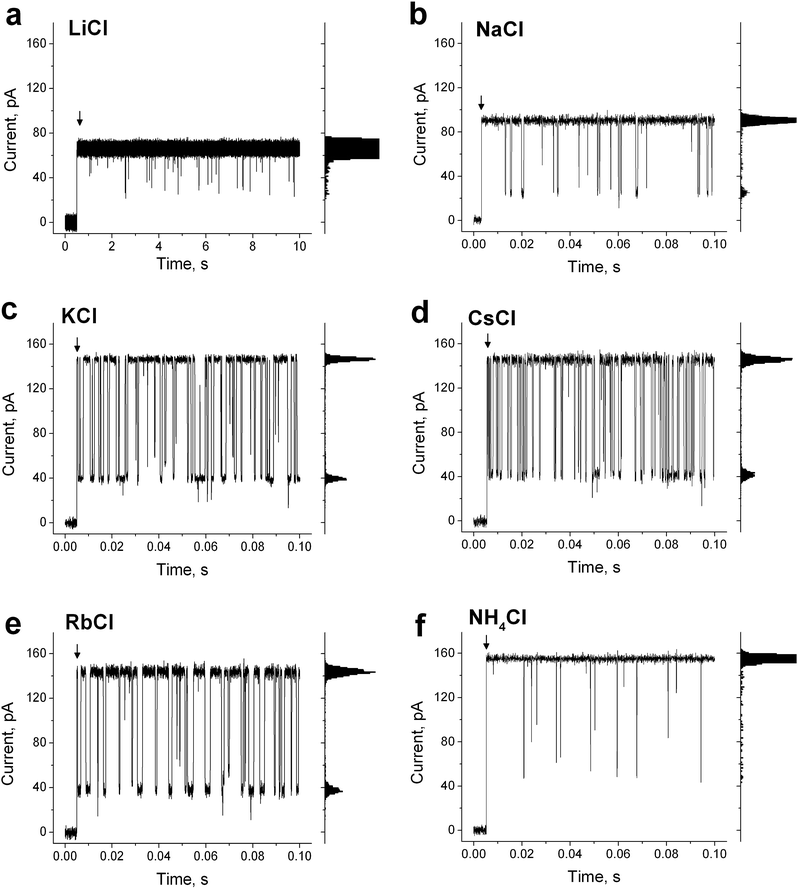 |
| Fig. 3 Typical traces of the ion current through single αHL nanopore in the presence of PEG1294 in the bath solution at a time resolution of 0.1 ms. (Arrows) Voltage shift from zero to 40 mV. Alkali- or ammonium chloride concentration was 4 M. Type of the electrolyte is shown in figure. The concentration of PEG was 400 μM. It can be seen that only in panel (a) the timescale changes in relation to the panels (b)–(f). The respective all-point histograms are shown at the right of each record and were used to give the mean value of blockage amplitudes. | |
The rate constants of PEG/αHL nanopore interaction in the presence of different alkali and ammonium chlorides
Rate constant for the association (kon) is intimately linked with the frequency of blocking events appearance. It was determined from the long record like those shown in Fig. 3. kon was calculated by assuming a simple bimolecular interaction such that kon = 1/(τon × [PEG]), where [PEG] is the PEG concentration and τon is the characteristic time between successive blockages.31,46 The rate constant for the dissociation (koff) of PEG was determined as 1/τoff, where τoff is the residence time of PEG into the pore-duration of the blockages, which was established from measuring more than 105 events as those shown in Fig. 3. Results are shown in Fig. 4, where clear dependence of on-rate (Fig. 4a) and off-rate (Fig. 4b) constants on the electrolyte type could be seen. In all cases, the rate constants were voltage dependent and rank as Cs+ > K+ ≥ Rb+ > Na+ > NH4+ > Li+ (for on-rate) and K+ < Rb+ < Na+ ≤ Cs+ < NH4+ < Li+ (for off-rate). The formation constant (KF), which is a derivate of association and dissociation constant, (KF = kon/koff), follows the same example (Fig. 4c).
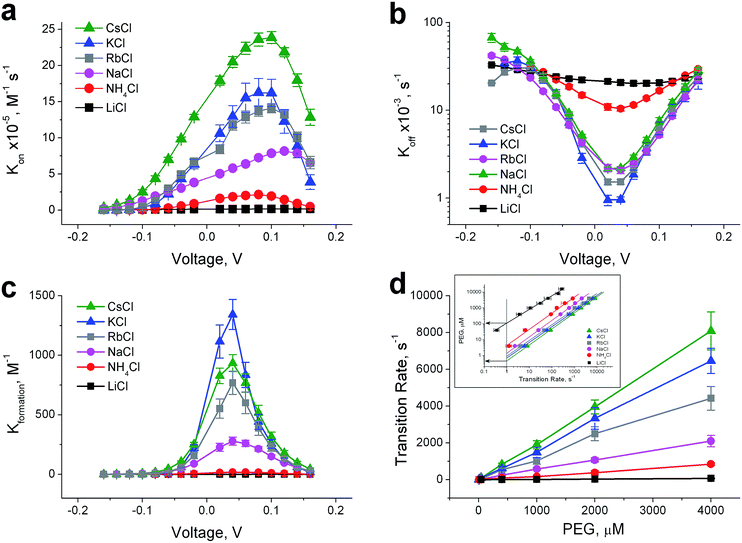 |
| Fig. 4 Voltage dependence and specific ion effect of alkali- and ammonium chlorides on PEG/αHL nanopore interaction. The average on-rate (a), off-rate (b), and PEG/αHL nanopore complex formation constant (c) are shown as a function of transmembrane voltage at different alkali- and ammonium chloride. The minimal values of koff are at 40 ± 10 mV. The data presented in (a) and (b) were used to build the dependence presented in (c). Transition rate and PEG concentration (d), the slope of the transition rate/PEG increases linearly and depends strongly on the salt type. Sensitiveness of αHL nanopore is dependent significantly on the cation type, the slope values are: 0.02 ± 0.01 (LiCl); 0.21 ± 0.01 (NH4Cl); 0.52 ± 0.01 (NaCl); 1.12 ± 0.04 (RbCl); 1.62 ± 0.02 (KCl); 2.02 ± 0.02 (CsCl). (Inset in d) Detection limit of PEG with αHL nanopore is dependent significantly on the cation type. The arrows indicate the lowest (CsCl: 0.52 ± 0.12 μM) and the highest (110.2 ± 5.2 μM) background equivalent PEG concentration at an event frequency of 1 Hz. The highest sensitivity was established for CsCl solution and the lowest for LiCl. First-order regression fitted well for the data with a slope of 1.01 ± 0.08 and the correlation coefficient is 0.99 ± 0.01. Li+ (black square), Na+ (pink circles), K+ (blue triangles), Rb+ (gray squares), Cs+ (green triangles) and NH4+ (red circles) chloride. | |
We also observed that in all cases (excepted for LiCl) there is a great dependence of the rate constants on the transmembrane voltage and this dependence demonstrates that nonionic PEG molecules bind with cations and becoming stable charged molecules in these solutions.24,46,62 The theoretical model developed by Kasianowicz's research group,63 and after supported by molecular dynamics simulations,64 indicates that the potassium binds to PEG molecule and this PEG–cation charged complex within nanopore exhibits electroosmotic flow dependent on transmembrane voltage.63,64 In the case of LiCl, it has not been observed dependence of the rate constants on the applied voltage, indicating that the PEG molecules do not form stable complexes with Li+.62 The established specific ion effects of examined salts on the association rate constant allowed comparison of the efficiency of a single αHL nanopore as a stochastic biosensor in solutions of different salts.
Stochastic αHL nanopore sensor provides beneficial features to the molecule sensing. This sensor has wide dynamic range, high sensitivity and low detection limit.65–67 The dynamic range can be defined as the range between the highest and the lowest analyte concentration which can be discriminated.68 In our case, the dynamic range was estimated at approximately 0.40 to 4000.00 μM (Fig. 4d). The sensitivity of the sensor is the slope of its calibration curve.69 In this study, from the transition rate as function of PEG concentration, it was calculated each slope to obtain the αHL nanopore sensitivity in the presence of alkali and ammonium chloride (Fig. 4d). The nanopore sensitivity values (s−1 μM−1) are: 0.02 ± 0.01 (LiCl); 0.21 ± 0.01 (NH4Cl); 0.52 ± 0.01 (NaCl); 1.12 ± 0.04 (RbCl); 1.62 ± 0.02 (KCl); 2.02 ± 0.02 (CsCl).
In any case, values of the transition rate increase linearly with PEG concentration (Fig. 4d). It permits to use the nanopore to measure the PEG concentration in solution. As demonstrated above, the sensitiveness of such sensor is considerably high in solution of 4 M CsCl than 4 M LiCl. To demonstrate this difference quantitatively, we decided to calculate the detection limit from the dependence of PEG concentration as a function of the transition rate (Fig. 4d inset). To facilitate the understanding, may be considered the lowest analyte concentration detectable by αHL nanopore sensor when the current blocking events occur at frequency of 1 Hz.24 The Fig. 4d (inset) demonstrates that the difference in the nanopore ability to detect the presence of PEG in solution (measured at practical 1 Hz of events) may achieve hundreds times: from 0.5 μM (CsCl) to >100 μM (LiCl).
Solubility of PEG
In order to find a reason for the established huge difference in the rate constants of PEG/αHL nanopore interaction and the sensitivity of this nanopore-based sensor to PEG, the solubility of PEG in 4 M of alkali chlorides was investigated. We have found that the PEG solubility in 4 M solutions of LiCl and NH4Cl was very high and difficult to achieve experimentally. The PEG solubility in solutions of NaCl, KCl, RbCl and CsCl was not very different each other: 5.1 ± 0.5 mM; 3.9 ± 0.2 mM; 3.3 ± 0.4 mM and 4.0 ± 0.2 mM, respectively. To include other two salts (LiCl and NH4Cl) in the analysis, the study of PEG solubility in 1/1 XCl/KCl mixtures was performed (where X represents Li+, Na+, NH4+, Cs+ or Rb+). The data (Fig. 5a) showed that the solubility is considerably influenced by cation type. The difference reaches several dozen times. However, the general ranking of ions, in order of effectiveness to salting-out of PEG (Rb+ ≥ Cs+ ≥ K+ > Na+ > NH4+ > Li+), is not exactly the typical Hofmeister series Li+ > Na+ > K+ > NH4+ > Rb+ > Cs+.51 The established values of the solubilities showed a significant negative correlation with the association rate constant and significant positive correlation with the dissociation rate constant (Fig. 5b and c). In accordance with the effect of halide anions,24 the alkali and ammonium cations perform their influence at both rate constants for PEG/αHL nanopore interaction through of the same salting-out phenomenon. This specific effect appears dominated by hydration–dehydration processes where cations and PEG compete for water.
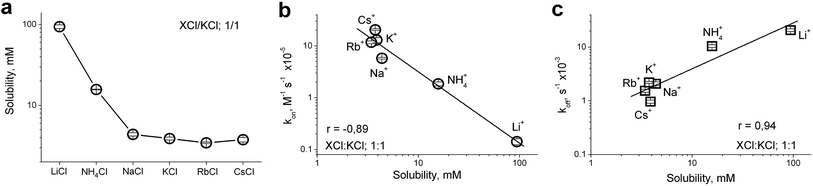 |
| Fig. 5 Solubility of PEG at 1 : 1 (M/M) XCl/KCl ratio, where X represents Li+, Na+, NH4+, Cs+ or Rb+ (a) dependence of the on-rate (b) and off-rate (c) constants of PEG/αHL nanopore interaction on solubility of PEG. The rate constant values were obtained at 40 mV of transmembrane potential. Total salt concentration was 4 M. (Data points) Means of 6–11 separate experiments ± SD. Correlation coefficient is shown in figure. | |
The absolute heat of hydration is important in the sensitivity of the nanopore to detect polymers?
The competition between ions and analyte for water is frequently noted as one of possible reasons of Hofmeister effects. However, there is not a report related to the importance of this for confined spaces as protein nanopores. We studied it and found that, except ammonium chloride, all alkali chlorides affected the associated rate constant uniformly: the larger the difference between the absolute energy of hydration Cl− and Me+ (where Me+ = Li+, Na+, K+, Rb+, Cs+, NH4+), smaller the association constant (Fig. 6). The behaviour of the dissociation constant is more complex. The dependence is biphasic with minimum at approximately to zero difference. Thus, one could choose the type of the electrolyte depending on your needs. If the goal is to increase the frequency of events (increase the sensitivity of the nanopore-based sensor), a salt with a lowest value of the difference in absolute energy of hydration should be used. In the contrast, if the aim is to increase the residence time of analyte (PEG) in the nanopore, a salt with zero difference should be utilized.
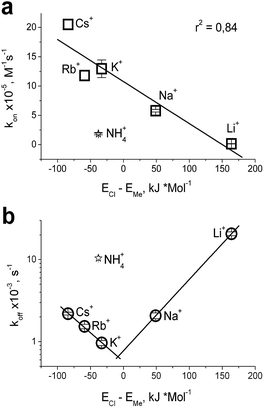 |
| Fig. 6 Dependence of associated (a) and dissociated (b) rate constants at the difference between the absolute heats of hydration anion and cation (ECl − EMe). Values of the absolute energy of hydration were obtained from literature.70 | |
Conclusions
We demonstrated that the type of ion has strong influence on all the kinetics parameters of the αHL nanopore. The alkali and ammonium cations perform their influence at both rate constants for PEG/αHL nanopore interaction through of the salting-out phenomenon. The on-rate and off-rate constants were changed and consequently the sensor sensitivity changed according to the type of salt. Furthermore, we observed that the rate constants have, in all cases (except LiCl), a great dependence on the transmembrane voltage. In the case of LiCl, it has not been observed dependence of the rate constants on the applied voltage, indicating that the PEG molecules do not form stable complexes with Li+. On the other hand, the others alkali metal cations significantly affect the interaction of PEG with nanopore and, similarly to the potassium, they also bind to this polymer. This finding indicates that neutral PEG molecules bind to the cations and becoming stable charged molecules in alkali and ammonium chloride solutions. Finally, the alkali and ammonium ions cause specific effects in confined spaces and these effects appear dominated by hydration–dehydration processes where cations and PEG compete for water. The results found in this study can help the understanding of the physicochemical conditions of molecular interactions in confined spaces. We hope that our results will advance the development of devices with sensor elements based on a single nanopore.
Acknowledgements
This work was supported by the Conselho Nacional de Desenvolvimento Científico e Tecnológico (CNPq), Fundação de Amparo à Ciência e Tecnologia do Estado de Pernambuco (FACEPE), Rede de Nanotecnologia Molecular e de Interfaces (RENAMI), Instituto Nacional de Ciência e Tecnologia de Nanotecnologia Para Marcadores Integrados (INAMI), Instituto Nacional de Ciência e Tecnologia de Fotônica (INCT INFO), Brazil.
Notes and references
- F. Hofmeister, Arch. Exp. Pathol. Pharmakol., 1888, 24, 247–260 Search PubMed.
- W. Kunz, J. Henle and B. W. Ninham, Curr. Opin. Colloid Interface Sci., 2004, 9, 19–37 CrossRef CAS.
- Y. Zhang, S. Furyk, D. E. Bergbreiter and P. S. Cremer, J. Am. Chem. Soc., 2005, 127, 14505–14510 CrossRef CAS PubMed.
- Y. J. Zhang and P. S. Cremer, Annu. Rev. Phys. Chem., 2010, 61, 63–83 CrossRef CAS PubMed.
- T. Lopez-Leon, M. J. Santander-Ortega, J. L. Ortega-Vinuesa and D. Bastos-Gonzalez, J. Phys. Chem. C, 2008, 112, 16060–16069 CAS.
- P. Jungwirth and P. S. Cremer, Nat. Chem., 2014, 6, 261–263 CrossRef CAS PubMed.
- D. F. Parsons, M. Bostrom, P. Lo Nostro and B. W. Ninham, Phys. Chem. Chem. Phys., 2011, 13, 12352–12367 RSC.
- J. Lyklema, Chem. Phys. Lett., 2009, 467, 217–222 CrossRef CAS.
- M. G. Cacace, E. M. Landau and J. J. Ramsden, Q. Rev. Biophys., 1997, 30, 241–277 CrossRef CAS PubMed.
- Y. J. Zhang and P. S. Cremer, Proc. Natl. Acad. Sci. U. S. A., 2009, 106, 15249–15253 CrossRef CAS PubMed.
- M. Boström, D. F. Parsons, A. Salis, B. W. Ninham and M. Monduzzi, Langmuir, 2011, 27, 9504–9511 CrossRef PubMed.
- P. Jungwirth, Faraday Discuss., 2009, 141, 9–30 RSC.
- W. Kunz, Curr. Opin. Colloid Interface Sci., 2010, 15, 34–39 CrossRef CAS.
- Y. Zhang and P. S. Cremer, Curr. Opin. Chem. Biol., 2006, 10, 658–663 CrossRef CAS PubMed.
- R. Leberman and A. K. Soper, Nature, 1995, 378, 364–366 CrossRef CAS PubMed.
- J. D. Batchelor, A. Olteanu, A. Tripathy and G. J. Pielak, J. Am. Chem. Soc., 2004, 126, 1958–1961 CrossRef CAS PubMed.
- A. W. Omta, M. F. Kropman, S. Woutersen and H. J. Bakker, Science, 2003, 301, 347–349 CrossRef CAS PubMed.
- J. D. Smith, R. J. Saykally and P. L. Geissler, J. Am. Chem. Soc., 2007, 129, 13847–13856 CrossRef CAS PubMed.
- L. M. Pegram and M. T. Record, J. Phys. Chem. B, 2008, 112, 9428–9436 CrossRef CAS PubMed.
- S. N. Timasheff, Adv. Protein Chem., 1998, 51, 355–432 CrossRef CAS PubMed.
- P. A. Grigorjev and S. M. Bezrukov, Biophys. J., 1994, 67, 2265–2271 CrossRef CAS PubMed.
- P. A. Gurnev, D. Harries, V. A. Parsegian and S. M. Bezrukov, ChemPhysChem, 2009, 10, 1445–1449 CrossRef CAS PubMed.
- O. V. Krasilnikov, R. Z. Sabirov and B. A. Tashmukhamedov, Biol. Membr., 1986, 3, 1057–1061 CAS.
- C. G. Rodrigues, D. C. Machado, A. M. da Silva, J. J. Junior and O. V. Krasilnikov, Biophys. J., 2011, 100, 2929–2935 CrossRef CAS PubMed.
- J. P. Aguiar, J. J. S. Junior, D. C. Machado, M. C. A. Melo and C. G. Rodrigues, Quim. Nova, 2015, 38, 817–827 CAS.
- H. Bayley, Clin. Chem., 2015, 61, 25–31 CAS.
- O. Braha, L. Q. Gu, L. Zhou, X. F. Lu, S. Cheley and H. Bayley, Nat. Biotechnol., 2000, 18, 1005–1007 CrossRef CAS PubMed.
- C. Yang, L. Liu, T. Zeng, D. W. Yang, Z. Y. Yao, Y. L. Zhao and H. C. Wu, Anal. Chem., 2013, 85, 7302–7307 CrossRef CAS PubMed.
- X. F. Kang, S. Cheley, X. Y. Guan and H. Bayley, J. Am. Chem. Soc., 2006, 128, 10684–10685 CrossRef CAS PubMed.
- A. J. Boersma and H. Bayley, Angew. Chem., Int. Ed., 2012, 51, 9606–9609 CrossRef CAS PubMed.
- O. V. Krasilnikov, C. G. Rodrigues and S. M. Bezrukov, Phys. Rev. Lett., 2006, 97 Search PubMed.
- J. W. F. Robertson, C. G. Rodrigues, V. M. Stanford, K. A. Rubinson, O. V. Krasilnikov and J. J. Kasianowicz, Proc. Natl. Acad. Sci. U. S. A., 2007, 104, 8207–8211 CrossRef CAS PubMed.
- H. C. Wu and H. Bayley, J. Am. Chem. Soc., 2008, 130, 6813–6819 CrossRef CAS PubMed.
- D. Wang, Q. Zhao, R. S. S. de Zoysa and X. Guan, Sens. Actuators, B, 2009, 139, 440–446 CrossRef CAS.
- A. J. Boersma, K. L. Brain and H. Bayley, ACS Nano, 2012, 6, 5304–5308 CrossRef CAS PubMed.
- T. C. Sutherland, Y. T. Long, R. I. Stefureac, I. Bediako-Amoa, H. B. Kraatz and J. S. Lee, Nano Lett., 2004, 4, 1273–1277 CrossRef CAS.
- L. Movileanu, J. P. Schmittschmitt, J. M. Scholtz and H. Bayley, Biophys. J., 2005, 89, 1030–1045 CrossRef CAS PubMed.
- R. Stefureac, Y. T. Long, H. B. Kraatz, P. Howard and J. S. Lee, Biochemistry, 2006, 45, 9172–9179 CrossRef CAS PubMed.
- L. Movileanu, Trends Biotechnol., 2009, 27, 333–341 CrossRef CAS PubMed.
- D. Rotem, L. Jayasinghe, M. Salichou and H. Bayley, J. Am. Chem. Soc., 2012, 134, 2781–2787 CrossRef CAS PubMed.
- J. Nivala, D. B. Marks and M. Akeson, Nat. Biotechnol., 2013, 31, 247–250 CrossRef CAS PubMed.
- S. Howorka, S. Cheley and H. Bayley, Nat. Biotechnol., 2001, 19, 636–639 CrossRef CAS PubMed.
- D. Branton, D. W. Deamer, A. Marziali, H. Bayley, S. A. Benner, T. Butler, M. Di Ventra, S. Garaj, A. Hibbs, X. H. Huang, S. B. Jovanovich, P. S. Krstic, S. Lindsay, X. S. S. Ling, C. H. Mastrangelo, A. Meller, J. S. Oliver, Y. V. Pershin, J. M. Ramsey, R. Riehn, G. V. Soni, V. Tabard-Cossa, M. Wanunu, M. Wiggin and J. A. Schloss, Nat. Biotechnol., 2008, 26, 1146–1153 CrossRef CAS PubMed.
- J. Clarke, H. C. Wu, L. Jayasinghe, A. Patel, S. Reid and H. Bayley, Nat. Nanotechnol., 2009, 4, 265–270 CrossRef CAS PubMed.
- D. Stoddart, L. Franceschini, A. Heron, H. Bayley and G. Maglia, Nanotechnology, 2015, 26 Search PubMed.
- C. G. Rodrigues, D. C. Machado, S. F. Chevtchenko and O. V. Krasilnikov, Biophys. J., 2008, 95, 5186–5192 CrossRef CAS PubMed.
- D. C. Machado, C. G. Rodrigues, A. M. B. da Silva, J. J. S. Junior and O. Krasilnikov, Biophys. J., 2010, 98, 332A CrossRef.
- M. Montal and P. Mueller, Proc. Natl. Acad. Sci. U. S. A., 1972, 69, 3561–3566 CrossRef CAS.
- S. Bhattacharya, L. Muzard, L. Payet, J. Mathe, U. Bockelmann, A. Aksimentiev and V. Viasnoff, J. Phys. Chem. C, 2011, 115, 4255–4264 CAS.
- P. G. Merzlyak, M. F. P. Capistrano, A. Valeva, J. J. Kasianowicz and O. V. Krasilnikov, Biophys. J., 2005, 89, 3059–3070 CrossRef CAS PubMed.
- J. M. Broering and A. S. Bommarius, J. Phys. Chem. B, 2005, 109, 20612–20619 CrossRef CAS PubMed.
- P. Lo Nostro and B. W. Ninham, Chem. Rev., 2012, 112, 2286–2322 CrossRef CAS PubMed.
- J. I. Partanen, J. Chem. Eng. Data, 2009, 54, 882–889 CrossRef CAS.
- J. I. Partanen, J. Chem. Eng. Data, 2010, 55, 249–257 CrossRef CAS.
- J. I. Partanen and A. K. Covington, J. Chem. Eng. Data, 2009, 54, 208–219 CrossRef CAS.
- R. A. Robinson, Trans. Faraday Soc., 1939, 35, 1217–1220 RSC.
- R. A. Robinson and D. A. Sinclair, J. Am. Chem. Soc., 1934, 56, 1830–1835 CrossRef CAS.
- B. F. Wishaw and R. H. Stokes, Trans. Faraday Soc., 1952, 49, 27–31 RSC.
- O. V. Krasilnikov, M. F. P. Capistrano, L. N. Yuldasheva and R. A. Nogueira, J. Membr. Biol., 1997, 156, 157–172 CrossRef CAS PubMed.
- O. V. Krasilnikov and R. Z. Sabirov, Gen. Physiol. Biophys., 1989, 8, 213–222 CAS.
- O. V. Krasilnikov, P. G. Merzlyak, L. N. Yuldasheva and M. F. Capistrano, Eur. Biophys. J. Biophys. Lett., 2005, 34, 997–1006 CrossRef CAS PubMed.
- M. F. Breton, F. Discala, L. Bacri, D. Foster, J. Pelta and A. Oulchaled, J. Phys. Chem. Lett., 2013, 4, 2202–2208 CrossRef CAS.
- J. E. Reiner, J. J. Kasianowicz, B. J. Nablo and J. W. F. Robertson, Proc. Natl. Acad. Sci. U. S. A., 2010, 107, 12080–12085 CrossRef CAS PubMed.
- A. Balijepalli, J. W. F. Robertson, J. E. Reiner, J. J. Kasianowicz and R. W. Pastor, J. Am. Chem. Soc., 2013, 135, 7064–7072 CrossRef CAS PubMed.
- H. Bayley, O. Braha and L. Q. Gu, Adv. Mater., 2000, 12, 139–142 CrossRef CAS.
- H. Bayley and P. S. Cremer, Nature, 2001, 413, 226–230 CrossRef CAS PubMed.
- G. H. Wang, L. Wang, Y. J. Han, S. Zhou and X. Y. Guan, Acc. Chem. Res., 2013, 46, 2867–2877 CrossRef CAS PubMed.
- E. W. Visser, L. J. van IJzendoorn and M. W. Prins, ACS Nano, 2016, 10, 3093–3101 CrossRef CAS PubMed.
- D. R. Thévenot, K. Toth, R. A. Durst and G. S. Wilson, Biosens. Bioelectron., 2001, 16, 121–131 CrossRef.
- Y. Marcus, Ion properties, Marcel Dekker, New York, 1997 Search PubMed.
Footnote |
† The authors dedicate this work to Dr Oleg Vladimirovich Krasilnikov (in memoriam). |
|
This journal is © The Royal Society of Chemistry 2016 |
Click here to see how this site uses Cookies. View our privacy policy here.