DOI:
10.1039/C6RA08283A
(Review Article)
RSC Adv., 2016,
6, 90739-90753
Non-enzymatic glycation mediated structure–function changes in proteins: case of serum albumin
Received
31st March 2016
, Accepted 5th September 2016
First published on 7th September 2016
Abstract
Albumin, a major plasma protein with extraordinary ligand binding properties, transports various ligands ranging from drugs, hormones, fatty acids, and toxins to different tissues and organs in the body. Albumin can undergo glycation on reacting with circulating reducing sugars, and this modification can occur even more frequently under hyperglycaemic conditions. Glycation is a non-enzymatic reaction of proteins and sugars, which gives rise to the formation of early and advanced glycation end products (AGEs). AGEs are a fundamental factor in the development and progression of severe diabetic complications such as nephropathy, neuropathy, retinopathy and cardiovascular disorders. The pathological impact of AGEs is mainly attributed to the structural modifications of proteins due to their cross-linking properties and the modification of lysine and arginine side chains. Herein, we review the structure–function effects of glycation on albumin, specifically focusing on its drug binding functions. Considering its physiological significance, there is a need to focus current research on determining the structure–function impacts of non-enzymatic glycation on serum albumin.
Introduction
Despite the efforts that have been made towards understanding and managing diabetes, this metabolic disorder and its complications are increasing unabated.1–3 Blood glucose levels are regulated by various factors affecting the digestion and absorption of dietary carbohydrates, hepatic glucose control, and the storage, production, and efficiency of insulin to stimulate glucose uptake.4 Considering the number of deaths associated with hyperglycaemia, there is a need to develop a utile approach for the prevention of diabetes as well as to understand its underlying pathophysiology.5,6 Improvements in lifestyle involving a healthy diet, weight loss and increased physical activity have been observed to have long lasting effects in the prevention of type 2 diabetes.7–9 In addition to being the leading cause of disease-related deaths, excessive sugar levels in diabetes could also accelerate the process of non-enzymatic glycation of various body proteins, nucleic acids and lipids.10,11
Glycation of proteins has been identified as a key player in the development of various complications in diabetes, such as neuropathy,12–15 nephropathy,16–20 retinopathy,21–24 and cardiovascular disorders.18,25–27 Due to the complexity and the physiological impact of glycation, for several decades, current diabetes research has focused on determining the physiological role of glycation end products (AGEs) and their biological effects. Louis Camille Maillard (1878–1936) first demonstrated the sugar amino acid reaction on heating. He proposed that the browning reaction involved the formation of an intermediate Schiff base intermediate; later, this reaction was named the “Maillard reaction”. Non-enzymatic glycation occurs even under normal conditions over the course of a lifetime, and accumulation of advanced glycation end products (AGEs) has been proposed to be the hallmark of the aging process.28 Glycation is a multistep, slow reaction between free amino groups in proteins and reducing sugars, as shown in Fig. 1. The glycation reaction leads to the formation of heterogeneous AGEs involving complex reactions such as oxidation, dehydration, and cyclization. Reducing sugars react with free amino groups in proteins to form a Schiff base which undergoes structural changes to form the Amadori product.29,30 Glyoxal, a product of glucose autoxidation, could react with proteins to form CML and GOLD, whereas methylglyoxal, which is a breakdown product of the Amadori product, can give rise to the formation of imidazolium cross links and MOLD. Glyoxallic acid, glyoxal and methylglyoxal are considered to be AGE intermediates because they can give rise to the formation of AGEs through glucose protein reactions. Since the discovery of the glycation reaction, extensive research has been carried out to analyse the properties of advanced glycation end products (AGEs). AGEs have been classified based on their fluorescence and cross-linking properties.31–33 The chemical process of glycation is sensitive to pH, temperature, hydration, the type of sugar, and buffer conditions.34
 |
| Fig. 1 Schematic of non-enzymatic glycation reactions and the formation of advanced glycation end products (AGEs). | |
Fig. 2 shows the structures of different sugars (glucose, fructose, ribose, etc.) as well as early (fructosamine), intermediate (glyoxal, methylglyoxal, 3-deoxygluconosone, 3-deoxygluconosone, etc.) and advanced glycation end products. Most of the AGEs identified to date are derivatives of either lysine or arginine. AGEs have also been found to form cross-links between two lysine residues or between a lysine and an arginine residue.35,36 Hyperglycaemic conditions, where blood glucose increases above normal levels, are known to result in an increased flux of glucose and its metabolic intermediates towards different pathways, contributing to the formation of highly toxic pro-oxidants called AGEs.37,38 The excess glucose present under hyperglycaemic conditions can enter the polyol pathway and can lead to increased accumulation of di-carbonyl compounds.39 Fig. 3 shows the different pathways involved in the formation and accumulation of AGEs.31,37,40 It has been observed that the metabolic intermediates of glucose exhibit comparatively higher reactivity with free amino groups in proteins than glucose itself.41 Glyoxyllic acid (GA), pyruvic acid (PA) and methylglyoxal (MG) are examples of the metabolic intermediates of glucose formed through different pathways.42 Methylglyoxal is a highly reactive oxaldehyde formed in vivo by the glycolytic pathway. Methylglyoxal leads to the modification of arginine residues, resulting in the formation of highly toxic fluorescent AGEs, i.e. argpyrimidine (Arg-P).43,44 Among the various heterogeneous AGEs analyzed to date and shown in Fig. 2, carboxymethyllysine (CML), carboxyethyllysine (CEL), argpyrimidine (Arg-P) and pentosidine have been observed to be the most predominant AGEs formed in the body under pathological conditions.43,45–49
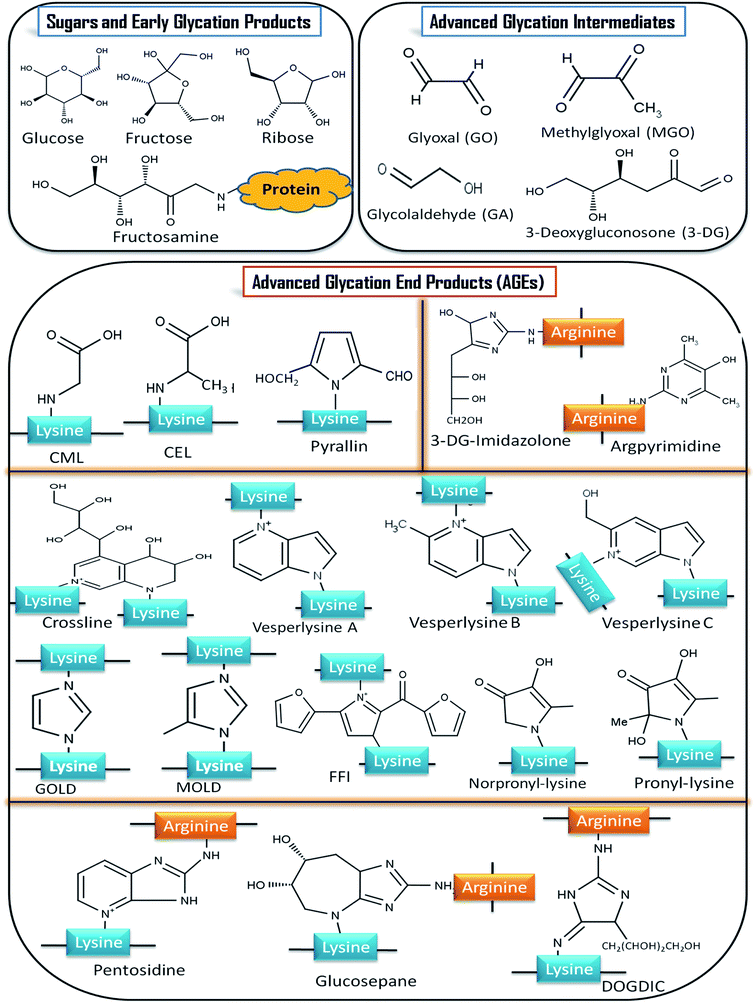 |
| Fig. 2 The structures of different sugars and early, intermediate and advanced glycation end products. | |
 |
| Fig. 3 Schematic of the different pathways involved in the formation and accumulation of AGEs. | |
Role of AGEs in diabetic complications
The current focus of diabetes research is moving away from the control of acute metabolic imbalances and towards the prevention of chronic complications such as neuropathy, nephropathy, retinopathy and cardiovascular complications. Non-enzymatic glycation and AGE accumulation have been proposed to be the main causes of the pathogenesis of these severe diabetic complications. AGEs are pro-oxidants with high reactivities and cross-linking properties, and they can activate a cascade of inflammatory pathways upon interaction with receptors to generate advanced glycation end products (RAGE).50–53
Diabetic retinopathy
Diabetic retinopathy results in poor vision or complete vision loss.54 Glycation and AGEs play key roles in the pathogenesis of retinopathy. Diabetic cataract, characterized by opacification of the lens, can also eventually result in vision loss. Non-enzymatic glycation of lens crystalline has been found to cause conformational changes leading to cross-linking and aggregation.55–57 The formation of AGEs and the accumulation of glycated crystalline aggregates have been found to be responsible for opacification, as determined from both in vitro and in vivo studies.58–61
Neurological disorders
The accumulation of AGEs under hyperglycaemic conditions has been linked with the development of diabetic neuropathy.62 Glycated myelin has been found to be more susceptible to the activation of macrophages and phagocytosis, which could lead to demyelination.63 The activation of the polyol pathway and the development of oxidative stress have been proposed to be the factors responsible for the pathogenesis of diabetic neuropathy.14,64 Glycation induces amyloid aggregation of proteins65 and also enhances the neuronal toxicity of β-amyloid aggregates.66 The role of AGEs in the progression of neurological disorders such as Alzheimer's disease67 and in the development of diabetic neuropathy have been well established. AGE-mediated toxicity can result in the development of pathological conditions such as Alzheimer's disease and Parkinson's disease.68,69
Micro and macro vascular complications
Both early glycation and AGEs are known to be responsible for the development and progression of vascular complications in diabetes patients.70–72 AGEs accumulation results in the development of cardiovascular complications, mainly due to structure–function modification and impairment of the mechanical properties of tissues through cross-linking of matrix proteins.73 AGEs can modulate cellular processes by interacting with cell surface receptors (receptors for AGEs, i.e. RAGE) and activating inflammatory pathways.50–53
Diabetic nephropathy
AGEs exhibit inflammatory and fibrotic effects on retinal tubular epithelial cells and are involved in the development of diabetic nephropathy.74,75 AGE accumulation can cause damage to the peritoneum and result in the loss of ultrafiltration capacity.76 Elevated levels of AGEs in the plasma, amyloid fibrils and skin of haemodialysis patients have been shown in recent studies.77 AGEs have been implicated in the progression of chronic kidney disease (CKD), specifically in diabetic nephropathy.78
Other complications
Non-enzymatic glycation and accumulation of AGEs is also involved in the progression and development of diabetic atherosclerosis,79,80 skin aging,81 liver disease,82 etc. It was found that AGEs enhance proliferation, migration and invasion of breast cancer cell lines.83 Also, it has been proposed that mitochondrial glycation could contribute to the development of diabetes, ageing, cancer, and neurodegeneration.84
Non-enzymatic glycation of proteins in vivo
Under both normal and hyperglycaemic conditions, many proteins have been found to be greatly affected by non-enzymatic glycation.85,86 In addition to the N-terminal amino group, proteins also contain a large number of lysine and arginine residues with side chains containing free amino groups. Proteins which have been reported to be modified due to non-enzymatic glycation include serum proteins, membrane proteins, enzymes and both intra- and extracellular structural proteins. Fig. 4 shows a schematic of proteins that are modified by glycation. Glycation leads to cross-linking of proteins, resulting in loss of their functions. Glycation-mediated cross-linking of collagen has been observed to be a key factor in skin aging.81,87 Non-enzymatic glycation of red blood cell (RBC) membrane proteins has been observed in diabetes.88 Glycation of insulin has been found to result in the impairment of its ability to regulate blood glucose.89 The restrained allosteric transition in hemoglobin due to glycation results in loss of its oxygen-carrying function and development of oxidative stress.90 Glycation of the milk protein casein has been shown to induce its oligomerization and affect its functional properties.91
 |
| Fig. 4 Different proteins modified by glycation. | |
The micro-heterogeneity and non-specificity of non-enzymatic glycation are major limitations in structural studies of glycated proteins using X-ray crystallography or nuclear magnetic resonance (NMR). A large number of structural studies of glycated proteins are limited to the study of secondary structural changes using circular dichroism. With the advent of mass spectroscopic analysis, new approaches have been proposed towards the quantitative analysis of glycation adducts and the identification of types of AGEs.92–96 The heterogeneity associated with non-enzymatic glycation has added to the complexity of the problem and has limited the possibilities of exploring the crystal structures of modified proteins.
Structure of albumin
Albumins are globular proteins with high water solubility; they are mainly involved in transport. The structure of serum albumin has been extensively studied based on X-ray crystallography, and it has been shown to exhibit a heart shape in its three-dimensional form. The molecular weight of serum albumin is 66.5 kDa. It is a single chain protein consisting of 585 residues. The three-dimensional crystal structure of serum albumin is shown in Fig. 5. The structure of albumin is broadly divided into three domains (I, II, III), each of which is further divided into two subdomains, A and B. The native structure of albumin is predominantly helical.97–101 Albumin contains 35 cysteine residues; 34 of them form disulfides and stabilize the structure. Also, human serum albumin contains only a single tryptophan residue (Trp-214), whereas its bovine homologue contains two tryptophan residues (Trp134 and Trp213).102 The fluorescence behaviour of these tryptophan residues has been extensively used to study ligand interactions and the structural changes in albumin.
 |
| Fig. 5 Three-dimensional structure of serum albumin and its ligand binding sites. | |
Albumin contains seven fatty acid binding sites103 (represented by spheres in Fig. 5) and two major drug binding sites, named Sudlow's site I and II.104–106 The bovine homologue of human serum albumin has been extensively used due to its high sequence similarity, low cost and high availability to understand the structure–functional changes upon ligand binding and the mechanisms of carrier functions.107,108 Due to its extraordinary ligand binding properties for a broad range of small molecules, such as fatty acids, bilirubin, thyroxine, bile acids and steroid hormones, albumin serves as a transporter for these compounds in the body.109–111
Multiple functions of albumin
In addition to its carrier function in the body, serum albumin performs multiple physiological functions.112–114 Albumin forms a major part of the soluble protein constituent in blood, where it performs various functions, such as a carrier for drugs, hormones, ions, bilirubin, etc.110,115–117 Due to its high solubility and high plasma concentration, albumin also plays a protective role for blood cells by maintaining osmotic pressure.118 The multiple functions performed by serum albumin are shown in Fig. 6. Due to its very good ligand binding properties, albumin is of great importance in the pharmacokinetics of many vital drugs.110,119–121 The two major drug-binding regions in albumin interact with a wide range of drugs for various pathological conditions. The three-dimensional structure of albumin is very well studied; its drug-binding sites have been characterized and confirmed based on co-crystallization studies using X-ray crystallography.102,103,106,122–124 A new, third drug site has been reported to be located in the IB subdomain of albumin.125
 |
| Fig. 6 Multiple functions of albumin. | |
Albumin also serves as a major fatty acid carrier of the body, with seven fatty acid binding sites. Curry et al. (1998),103 using an X-ray crystallographic approach, revealed the asymmetric distribution of fatty acid binding sites in albumin. Different studies have also evaluated the binding affinity of various hormones to albumin. Watanabe et al.126 determined the affinity of BSA for six steroid hormones, i.e. alpha-estradiol, ethynylestradiol, progesterone, androsterone, dehydroisoandrosterone and testosterone; they concluded that BSA accelerates the transport of hormones. Albumin also serves a carrier for thyroxin, which is an important thyroid hormone.123,127,128 Albumin plays a crucial role as a physiological transporter of the metal ions Cu2+ and Zn2+ in the bloodstream. Albumin also exhibits affinity for Ni2+, Co2+, and Cd2+ in vivo, which is of toxicological importance.129 It binds with calcium in a pH-dependent manner, and its affinity increases with increasing pH due to electrostatic effects.130
Recently, many studies have been carried out to determine the interaction of albumin with a wide range of ligands, ranging from drugs to natural phytochemicals from plants.109,131–144 In addition to its role as a drug carrier, albumin exhibits esterase activity; extensive studies have been carried out to determine the enzymatic properties of albumin.145–151 Based on site-directed mutagenesis studies, Arg410 and Tyr411 have been shown to be the key residues responsible for the esterase activity of albumin.152,153
Glycation of albumin
Because it is the major plasma protein, and also because it contains many lysine and arginine residues, albumin is highly prone to non-enzymatic glycation.154 Different studies have identified lysine residues in albumin to be the most frequently glycated residues based on both in vitro and in vivo analysis. All the lysine and arginine residues in albumin are shown in Fig. 7. Among the 59 lysine residues, 34 have been reported to be glycated in different studies based on mass spectroscopic analysis.149,155–160 In addition to lysine residues, there are 24 arginine residues in albumin, which can also give rise to the formation of AGEs and cross-links on glycation.
 |
| Fig. 7 All the lysine and arginine residues in albumin. | |
Hyperglycaemic conditions resulting in excessive blood sugar levels and the high plasma concentration of albumin also make it highly susceptible to glycation.161,162 Prolonged exposure to glucose under hyperglycaemic conditions is known to worsen the situation by accelerating the process of non-enzymatic glycation, leading to excessive formation of highly reactive intermediates of AGEs.
In spite of recent continuous efforts, information about the structural and functional impacts of specific AGEs on various proteins remains poorly understood. Glycation-induced structural modifications in albumin can impact its functions.163 Because albumin is the major drug carrier of the body, the glycation of albumin can largely impact the pharmacokinetics and pharmacodynamics of many drugs and other ligands that interact with albumin.164,165 Arginine and lysine residues are the most common glycation sites in albumin both in vivo and in vitro. The major drug binding sites in albumin are located in subdomain II A (Sudlow's site I), subdomain III A (Sudlow's site II) and the newly studied subdomain IB. The seven fatty acid binding sites located in three different domains of albumin make it a major depot for fatty acids and hormones in the body.
Site I in albumin is the preferred binding site for anionic heterocyclic drugs, whereas site II is preferentially occupied by ligands having aromatic carboxylate groups with extended conformations.125,166–168 Recently, a number of studies have been carried out using both HSA and BSA to determine the impact of glycation on the structure and ligand binding properties of albumin;163,169–174 however, the impact of individual AGEs remains undefined.
Glycation-mediated conformational transitions in albumin can eventually influence its ligand binding properties.175 Glycated albumin has been extensively characterized by gel electrophoresis and by UV-Vis, fluorescence and infrared spectroscopy. Glycation does not occur in a site-specific manner and results in heterogeneous end products with varying extents of modification, types of modification (AGEs) and sites of modification.
Structural changes in albumin upon glycation
The high heterogeneity associated with glycation has limited structural studies of glycated proteins; to date, there is only one crystal structure of a glycated protein i.e. glycated hemoglobin (3B75)176 in the Protein Data Bank (PDB). Other biophysical methods, such as circular dichroism (CD),177 differential scanning colorimetry,178 fluorescence,169 and scanning and transmission electron microscopy (SEM and TEM),65 have been mainly used to study the effect of glycation on proteins. Based on CD studies, it is well established that glycation induces structural changes in albumin, specifically resulting in increased beta sheet content.173,179–181 Also, it is evident from studies using both in vitro and in vivo glycation models that glycation is associated with significant structural changes.163
In particular, the conformational changes in albumin have been probed based on tryptophan fluorescence, which is directly affected by glycation.182 Human serum albumin contains only one tryptophan residue (i.e. Trp-214), located in domain II, whereas its bovine analogue contains two tryptophan residues, i.e. Trp-134 and Trp213. It has been shown in several studies that glycation results in the quenching of tryptophan fluorescence compared to the native conformation, suggesting changes in the microenvironment of these residues.170,183 Based on in vitro experiments, it has been shown that unfolding due to glycation results in opening of the hydrophobic regions of albumin during prolonged glycation.173,183
In a comparative study, Guerin-Dubourg et al.184 determined the structure–function changes in glucose and methylglyoxal-modified albumin with methylglyoxal using in vivo glycated albumin purified from diabetic patients. Enhanced oxidative modification was observed in the cases of both in vitro and in vivo glycated albumin. They also determined the differences in the structural and functional features of in vivo albumin compared to the in vitro models. Chesne et al.185 reported oxidative modifications of albumin using pathophysiological concentrations of glucose. Based on the amounts of free energy change and unfolding, it was demonstrated that glucose induces fine structural changes in human serum albumin; it was also suggested that 175 mg dL−1 glucose concentration is a critical point for structure and function alteration in albumin.186 The changes in helicity, AGE fluorescence, lysine modification, surface tension value and formation of Amadori products were observed to be similar in both in vitro and in vivo conditions. Also, it was noticed that arginine modification by glucose occurred only in vivo; it was not observed under in vitro conditions.187 The observed difference was proposed to be due to the presence of a glucose-derived di-carbonyl compound under physiological conditions.
Glycation induced aggregation and fibrillation
Glycated albumin has been observed to form fibrillar or amorphous aggregates with high affinity to amyloid specific dyes such as Congo red (CR) and Thioflavin T (ThT). In contrast to the native helical structure of albumin, its glycated form contains residues in β-sheeted conformations, as observed with circular dichroism spectropolarimetry. From X-ray fiber diffraction studies, the glycated form of albumin was found to exhibit a cross-β structure. Based on these findings, it was concluded that glycation can induce refolding of native helical albumin into amyloid fibrils comprising cross-β structures.65 In 2004, Obrenovich and Monnier179 reported a finding from the Dutch group of Gebbink and colleagues which showed that the posttranslational process of non-enzymatic glycation is a key mechanism in amyloid formation. It was shown that glycation induces a conformational transition in albumin from the native helical conformation to the cross-beta conformation. Based on these observations, they also proposed glycation as a predisposing factor for amyloidosis.
Based on a study to determine the effects of temperature on the aggregation of albumin using FTIR spectroscopy along with polyacrylamide gel electrophoresis (PAGE), it was concluded that glycation also plays a role in protein aggregation.188 Rondeau et al.183 further studied the thermal aggregation process of glycated albumin at different glucose concentrations; they found that glycation alters the native structure of BSA. They showed that the partial unfolding of the tertiary structure was similar in the case of both native and glycated BSA, and the aggregation was found to be progressively restrained with increasing glucose concentration. Ribosylation of BSA also has been reported to induce rapid misfolding and form globular amyloid-like aggregates with high cytotoxicity to neural cells.189 In another study, fructosylation of HSA was shown to result in the generation of neo-epitopes. Also, it was observed that fructosylation resulted in protein aggregation based on gel electrophoresis.190 The prolonged glycation of human serum albumin (HSA) results in significant structural changes, and 20 days of incubation with sugars induces significant amyloid fibril formation. It was proposed that prolonged glycation of HSA is associated with a conformational transition from the native helical structure to a beta-sheeted amyloid form.191
Glycation is known to differentially modulate the structure and function of proteins.164,192 The structural impact of glycation has been found to differ with respect to the type of sugar, the extent of modification, the type of AGEs, buffer conditions, temperature, pH, type of protein, etc. The residues involved in the glycation mainly determine the functional impact of glycation on proteins.
Effect of glycation on albumin functions
Effect on drug transport function
Albumin is the major drug carrier in the body. In addition to drugs, albumin also interacts with various ligands such as flavonoids,125,193–195 dyes and pigments,196–198 synthetic compounds, ions, and many other bioactive phytochemicals.142,199,200 Extensive studies have been carried out, including both in vitro drug binding studies using absorbance or fluorescence-based methods, isothermal calorimetry (ITC), and X-ray crystallography in order to determine the binding sites of many drugs in albumin. Fig. 8 presents an overview of the overall impact of glycation on the structure and function of albumin.
 |
| Fig. 8 The overall impact of glycation on albumin. | |
Glycation is known to induce conformational changes in albumin which can affect its functions, mainly its drug binding properties. The binding affinity of HSA has been reported to decrease due to conformational changes or steric hindrance upon non-enzymatic glycation.201 Significant impairments in the binding affinity of albumin have been observed using both in vitro and in vivo glycated albumin based on estimations of dissociation constant values and free drug fractions. Loss of drug interaction has been observed in the case of both glycated albumin purified from diabetic patients and that obtained from in vitro glycation.202
The two major drug-binding regions in albumin interact with a wide range of drugs for various pathological conditions. The three-dimensional structure of albumin is very well studied, and its ligand-binding sites have been confirmed based on co-crystallization studies using X-ray crystallography.102,103,106,122,124,128 A third drug site has been newly reported to be located in the subdomain IB of albumin.125 Based on studies using methylglyoxal (MGO) modified albumin, it was found that glycation involved the arginine residues responsible for the ligand binding and enzyme activity (esterase) of albumin. Furthermore, simulation and molecular modelling results suggested that hydroimidazolone formation upon MGO modification caused structural changes that resulted in the disruption of arginine-mediated h-bonding and loss of electrostatic contacts.149 The clinical relevance and the pathophysiologic importance of non-enzymatic glycation of albumin in diabetes, where glycation occurs at an accelerated rate, and the development of diabetic complications have been extensively reviewed by Cohen (2013).203
In our work on structural changes mediated by the functional impact of specific AGE modifications on BSA, we demonstrated changes in the drug-binding properties of albumin upon specific AGE modifications.164 It was evident that glycation results in asymmetric conformational changes in BSA, leading to drug binding sites with reduced pocket volumes, depths and accessible surface areas. The Arg-P modification of arginine and the CEL modification of lysine residues in drug sites I and II resulted in comparatively shallow binding pockets. AGE-modified albumin exhibited lower affinities for drugs in both sites I and II.
Based on the crystal structures of HSA complex with glucose or fructose, Wang et al.204 showed that glucose covalently binds with Lys195 and Lys199 in its linear forms. Glucose was found to be covalently bonded to Lys195; however, this was not seen in the case of fructose, which bound in cyclic form. From the crystal structure observations, they proposed a ring opening mechanism for glucose involving Lys-195 and Lys-199 residues. Because Lys195 and Lys199 lie at the entrance of drug site I in albumin, their glycation can affect the interaction of drugs and can result in steric hindrance of drug binding.
The molecular interaction of different antibiotics with albumin showed that the capacity of antibiotics to interact with albumin is mainly determined by their chemical structures. Changes in the pharmacokinetics of teicoplanin, an antibiotic drug used against gram-positive bacteria, were observed in patients with hyperglycaemic hypoalbuminaemia. The patients showed comparatively lower serum levels of drugs following drug administration, and non-enzymatic glycation of albumin under hyperglycaemic conditions was proposed to be responsible for the poor affinity of albumin for teicoplanin.205
Effects on fatty acid carrier function
Albumin with seven fatty acid binding sites facilitates the uptake of fatty acids by organs in need of these substrates.206,207 The structural changes upon glycation could affect the fatty acid carrier functions of albumin. It was observed that glycation of albumin impairs fatty acid affinity, which results in enhanced arachidonate oxygenation and also hyper-activation of platelets, as seen in type 2 diabetes patients.208 The impaired lipid binding properties also affect the fatty acid carrier function of albumin to required locations.209
Changes in the affinities of glycated albumin toward fatty acids such as linoleic acid, oleic acid, caproic acid, and lauric acid were investigated based on kinetic studies. Significant decreases were observed in the binding affinities of linoleic acid (84%), oleic acid (84%), caproic acid (90%), and lauric acid (87%). The decrease was found to be in correlation with the extent of lysine modification in glycated albumin; this also suggested that albumin loses its fatty acid binding ability due to the glycation of lysine residues involved in fatty acid binding.210
Effects on antioxidant function
Changes in the antioxidant and radical scavenging properties of albumin have been observed both in patients with diabetes and using in vitro glycation models. In an in vitro study using BSA, it was observed that glycation results in impairment of the antioxidant functions of albumin. The conformational changes in albumin were found to be in good agreement with the loss of its antioxidant function. Based on studies with in vivo glycated albumin, poor glycemic control and non-enzymatic glycation in diabetic patients were proposed to be responsible for the loss of the antioxidant function of albumin.211 In another study with methylglyoxal modified albumin, it was suggested that the deleterious effects induced by carbonyl stress in the case of diabetic patients could also be due to impairment of the antioxidant capacity of albumin upon di-carbonyl modification.212 In addition to this, Faure et al. also compared the antioxidant properties and structural changes in albumin purified from patients with diabetes treated with metformin or sulfonylurease and in healthy subjects. In case of patients treated with metformin and sulfonylurease, both the oxidative stress and non-enzymatic glycation were found to be significantly lower in patients treated with metformin. They suggested that a decrease in the antioxidant capacity of albumin in patients with diabetes could lead to oxidative stress development, resulting in vascular and metabolic complications.
Glycated albumin with impaired antioxidant functions has been shown to enhance LDL oxidation, mainly by the formation of superoxide radicals. Also, BSA modified by conjugating carboxyl groups with p-aminophenyl α-D-glucopyranoside was found to exhibit membrane-perturbing activities.213
Effects on esterase function of albumin
Glycation-induced conformational transitions could also affect the enzymatic properties of albumin. Studies using single and double recombinant mutants of HSA suggested that the consequences of non-enzymatic glycation on albumin can be partially explained based on the blockage of the positive charges of lysine residues at Lys199, Lys439 and Lys525. Also, the decrease in the esterase activity of modified albumin was proposed to be due to conformational changes resulting in an increased distance between Arg-410 and Tyr-411, the two residues responsible for the enzyme activity of albumin.165,171,214 In a recent report, a dose-dependent decrease in the esterase activity of albumin was found with respect to the levels of glycation and protein aggregation.
Interaction of phytochemicals with albumin
A decrease in the binding affinity was also observed for various phytochemicals, such as quercetin, rutin, fisetin, morin and genistein, based on a multi-spectroscopic approach with glycated human serum albumin (HSA). When compared to other polyphenols, the maximum decrease in affinity was observed for quercetin.215 It has been also proposed that non-enzymatic glycation of albumin could result in impaired binding affinities for dietary polyphenols and phenolic acids.216 Also, in our attempt to determine the effects of methylglyoxal modification of albumin on sinigrin interaction, we observed that MGO-modified BSA showed comparatively lower affinity than native BSA.142
Effects of glycated albumin on cells
Amadori adducts in glycated albumin have been found to be responsible for the development of diabetic nephropathy.217,218 AGE-modified albumin has been shown to exhibit eryptotic properties on RBCs and is suggested to be responsible for the occurrence of abnormal morphologies in pathological conditions such as diabetes and chronic kidney disease.219 Glycated albumin has been found to stimulate smooth muscle cell growth and migration. Also, it was proposed that glycated albumin may play a role in the development of diabetic atherogenesis.220,221 Amadori adducts have been found to increase nitric oxide (NO) synthase activity in endothelial cells.222 Also, it was observed that Amadori adducts modulate NO synthase activity; also, these effects of glycated albumin were found to be fundamental in the development of diabetic nephropathy.223
Structural perturbation upon Amadori modification of lysine residues has been found to be responsible for the overall immunogenicity of a glycated serum protein; this may lead to the recognition of Amadori-glycated proteins by serum autoantibodies in diabetes patients.224–226
Conclusion
Despite the discovery of the Maillard reaction by Louis Camille Maillard in 1912, research in the area of glycation has been limited. With increasing research and the advent of new techniques such as mass spectrometry, there is increasing interest in this area. Despite the complexities of glycation and heterogeneity in the products of glycation reactions, extensive research has been performed in this area in the past two decades. The crystal structure of glycated hemoglobin, solved by our group, and glucose and fructose-linked structures of human serum albumin are the only three dimensional structures of glycated proteins determined using X-ray crystallography to date. The multifunctional albumin is a very important protein due to its physiological role in the transport of various drugs, hormones, and metal ions, maintenance of oncotic pressure, esterase activity and antioxidant functions. Due to its short half-life and high exposure to blood glucose fluctuations, glycated albumin has also been suggested to be a better marker of the glycemic index in diabetic patients. Non-enzymatic glycation of albumin affects its structure and functions, and there is a need to adopt an alternative approach to improve the efficacy of drugs in patients with hyperglycaemic conditions, where glycation occurs at an accelerated rate. Furthermore, future studies in clinical and pathophysiological scenarios will enhance our understanding of the glycation of proteins and its structure–function impacts.
Acknowledgements
The authors thank SASTRA University for providing the infrastructure to carry out the research and to the Department of Biotechnology, India for a research grant (102/IFD/SAN/1524/2016-2017).
References
- N. Unwin, J. Shaw, P. Zimmet and K. G. Alberti, Diabetic Med., 2002, 19, 708–723 CrossRef CAS PubMed.
- A. Accurso, R. K. Bernstein, A. Dahlqvist, B. Draznin, R. D. Feinman, E. J. Fine, A. Gleed, D. B. Jacobs, G. Larson, R. H. Lustig, A. H. Manninen, S. I. McFarlane, K. Morrison, J. V. Nielsen, U. Ravnskov, K. S. Roth, R. Silvestre, J. R. Sowers, R. Sundberg, J. S. Volek, E. C. Westman, R. J. Wood, J. Wortman and M. C. Vernon, Nutr. Metab., 2008, 5, 9 Search PubMed.
- T. Tuomi, N. Santoro, S. Caprio, M. Cai, J. Weng and L. Groop, Lancet, 2013, 383, 1084–1094 CrossRef.
- P. Zimmet, K. G. Alberti and J. Shaw, Nature, 2001, 414, 782–787 CrossRef CAS PubMed.
- R. G. McCoy, H. K. Van Houten, J. Y. Ziegenfuss, N. D. Shah, R. A. Wermers and S. A. Smith, Diabetes Care, 2012, 35, 1897–1901 CrossRef PubMed.
- D. K. Tobias, A. Pan, C. L. Jackson, E. J. O'Reilly, E. L. Ding, W. C. Willett, J. E. Manson and F. B. Hu, N. Engl. J. Med., 2014, 370, 233–244 CrossRef CAS PubMed.
- F. B. Hu, J. E. Manson, M. J. Stampfer, G. Colditz, S. Liu, C. G. Solomon and W. C. Willett, N. Engl. J. Med., 2001, 345, 790–797 CrossRef CAS PubMed.
- J. Tuomilehto, J. Lindstrom, J. G. Eriksson, T. T. Valle, H. Hamalainen, P. Ilanne-Parikka, S. Keinanen-Kiukaanniemi, M. Laakso, A. Louheranta, M. Rastas, V. Salminen and M. Uusitupa, N. Engl. J. Med., 2001, 344, 1343–1350 CrossRef CAS PubMed.
- J. Lindstrom, M. Peltonen, J. G. Eriksson, P. Ilanne-Parikka, S. Aunola, S. Keinanen-Kiukaanniemi, M. Uusitupa and J. Tuomilehto, Diabetologia, 2013, 56, 284–293 CrossRef CAS PubMed.
- D. Daneman, Diabetes Care, 2001, 24, 801–802 CrossRef CAS PubMed.
- H. Vlassara and M. R. Palace, J. Intern. Med., 2002, 251, 87–101 CrossRef CAS PubMed.
- P. J. Thornalley, in International Review of Neurobiology, Academic Press, 2002, vol. 50, pp. 37–57 Search PubMed.
- I. Misur, K. Zarkovic, A. Barada, L. Batelja, Z. Milicevic and Z. Turk, Acta Diabetol., 2004, 41, 158–166 CrossRef CAS PubMed.
- R. Wada and S. Yagihashi, Ann. N. Y. Acad. Sci., 2005, 598–604 CrossRef CAS PubMed.
- K. Sugimoto, M. Yasujima and S. Yagihashi, Curr. Pharm. Des., 2008, 14, 953–961 CrossRef CAS PubMed.
- J. M. Forbes and M. E. Cooper, Amino Acids, 2012, 42, 1185–1192 CrossRef CAS PubMed.
- M. Lassila, K. K. Seah, T. J. Allen, V. Thallas, M. C. Thomas, R. Candido, W. C. Burns, J. M. Forbes, A. C. Calkin, M. E. Cooper and K. A. Jandeleit-Dahm, J. Am. Soc. Nephrol., 2004, 15, 2125–2138 CrossRef CAS PubMed.
- S. Yamagishi, S. Maeda, T. Matsui, S. Ueda, K. Fukami and S. Okuda, Biochim. Biophys. Acta, 2012, 5, 663–671 CrossRef PubMed.
- J. M. Forbes and M. E. Cooper, Amino Acids, 2010, 42, 1185–1192 CrossRef PubMed.
- P. J. Beisswenger, S. K. Howell, G. B. Russell, M. E. Miller, S. S. Rich and M. Mauer, Diabetes Care, 2013, 36, 3234–3239 CrossRef CAS PubMed.
- A. W. Stitt, Exp. Mol. Pathol., 2003, 75, 95–108 CrossRef CAS PubMed.
- Y. Sharma, S. Saxena, A. Mishra, A. Saxena and S. M. Natu, Journal of Ocular Biology, Diseases, and Informatics, 2012, 5, 63–69 CrossRef PubMed.
- M. Chen, T. M. Curtis and A. W. Stitt, Curr. Med. Chem., 2013, 20, 3234–3240 CrossRef CAS PubMed.
- R. Milne and S. Brownstein, Amino Acids, 2013, 44, 1397–1407 CrossRef CAS PubMed.
- S.-i. Yamagishi, K. Nakamura and T. Imaizumi, Curr. Diabetes Rev., 2005, 1, 93–106 CrossRef CAS PubMed.
- M. Peppa and S. A. Raptis, Curr. Diabetes Rev., 2008, 4, 92–100 CrossRef CAS PubMed.
- Z. Hegab, S. Gibbons, L. Neyses and M. A. Mamas, World J. Cardiol., 2012, 4, 90–102 CrossRef PubMed.
- O. Nedic, S. I. Rattan, T. Grune and I. P. Trougakos, Free Radical Res., 2013, 1, 28–38 CrossRef PubMed.
- K. Sztanke and K. Pasternak, Ann. Univ. Mariae Curie-Sklodowska, Sect. D, 2003, 58, 159–162 Search PubMed.
- F. J. Tessier, Pathol. Biol., 2010, 58, 214–219 CrossRef CAS PubMed.
- R. Singh, A. Barden, T. Mori and L. Beilin, Diabetologia, 2001, 44, 129–146 CrossRef CAS PubMed.
- M. Peppa, J. Uribarri and H. Vlassara, Clin. Diabetes, 2003, 21, 186–187 CrossRef.
- N. Ahmed, Diabetes Res. Clin. Pract., 2005, 67, 3–21 CrossRef CAS PubMed.
- H. Vlassara and G. E. Striker, Nat. Rev. Endocrinol., 2011, 7, 526–539 CrossRef CAS PubMed.
- K. M. Biemel, O. Reihl, J. Conrad and M. O. Lederer, J. Biol. Chem., 2001, 276, 23405–23412 CrossRef CAS PubMed.
- P. T. B. Bullock, D. G. Reid, W. Ying Chow, W. P. W. Lau and M. J. Duer, Biosci. Rep., 2014, 34, e00096 CrossRef PubMed.
- M. Brownlee, Nature, 2001, 414, 813–820 CrossRef CAS PubMed.
- D. Aronson, Adv. Cardiol., 2008, 45, 1–16 CAS.
- S. S. Chung, E. C. Ho, K. S. Lam and S. K. Chung, J. Am. Soc. Nephrol., 2003, 14, S233–S236 CrossRef CAS PubMed.
- S. F. Yan, R. Ramasamy and A. M. Schmidt, Nat. Clin. Pract. Endocrinol. Metab., 2008, 4, 285–293 CrossRef CAS PubMed.
- Y. Hamada, N. Araki, N. Koh, J. Nakamura, S. Horiuchi and N. Hotta, Biochem. Biophys. Res. Commun., 1996, 228, 539–543 CrossRef CAS PubMed.
- C. Henning, K. Liehr, M. Girndt, C. Ulrich and M. A. Glomb, J. Biol. Chem., 2014, 289, 28676–28688 CrossRef CAS PubMed.
- R. Gomes, M. Sousa Silva, A. Quintas, C. Cordeiro, A. Freire, P. Pereira, A. Martins, E. Monteiro, E. Barroso and A. Ponces Freire, Biochem.
J., 2005, 385, 339–345 CrossRef CAS PubMed.
- J. Kim, O. S. Kim, C.-S. Kim, E. Sohn, K. Jo and J. S. Kim, Exp. Mol. Med., 2012, 44, 167–175 CrossRef PubMed.
- S. Reddy, J. Bichler, K. J. Wells-Knecht, S. R. Thorpe and J. W. Baynes, Biochemistry, 1995, 34, 10872–10878 CrossRef CAS PubMed.
- K. Ikeda, T. Higashi, H. Sano, Y. Jinnouchi, M. Yoshida, T. Araki, S. Ueda and S. Horiuchi, Biochemistry, 1996, 35, 8075–8083 CrossRef CAS PubMed.
- M. L. M. Lieuw-A-Fa, V. W. M. van Hinsbergh, T. Teerlink, R. Barto, J. Twisk, C. D. A. Stehouwer and C. G. Schalkwijk, Nephrol., Dial., Transplant., 2004, 19, 631–636 CrossRef CAS.
- S. S. Sivan, E. Tsitron, E. Wachtel, P. Roughley, N. Sakkee, F. van der Ham, J. Degroot and A. Maroudas, Biochem. J., 2006, 399, 29–35 CrossRef CAS PubMed.
- Y. Koyama, Y. Takeishi, T. Arimoto, T. Niizeki, T. Shishido, H. Takahashi, N. Nozaki, O. Hirono, Y. Tsunoda, J. Nitobe, T. Watanabe and I. Kubota, J. Card. Failure, 2007, 13, 199–206 CrossRef CAS PubMed.
- S. F. Yan, R. Ramasamy, Y. Naka and A. M. Schmidt, Circ. Res., 2003, 93, 1159–1169 CrossRef CAS PubMed.
- R. Ramasamy, S. J. Vannucci, S. S. Yan, K. Herold, S. F. Yan and A. M. Schmidt, Glycobiology, 2005, 15, 10 CrossRef PubMed.
- C. Ott, K. Jacobs, E. Haucke, A. Navarrete Santos, T. Grune and A. Simm, Redox Biol., 2014, 2, 411–429 CrossRef CAS PubMed.
- R. Ramasamy, S. F. Yan and A. M. Schmidt, in Atherosclerosis, John Wiley & Sons, Inc, 2015, pp. 27–41 Search PubMed.
- A. W. Stitt, Exp. Mol. Pathol., 2003, 75, 95–108 CrossRef CAS PubMed.
- R. E. Perry, M. S. Swamy and E. C. Abraham, Exp. Eye Res., 1987, 44, 269–282 CrossRef CAS PubMed.
- M. S. Swamy and E. C. Abraham, Invest. Ophthalmol. Visual Sci., 1987, 28, 1693–1701 CAS.
- M. S. Swamy, C. Tsai, A. Abraham and E. C. Abraham, Exp. Eye Res., 1993, 56, 177–185 CrossRef CAS PubMed.
- M. S. Swamy and E. C. Abraham, Invest. Ophthalmol. Visual Sci., 1989, 30, 1120–1126 CAS.
- H.-P. Hammes, A. Alt, T. Niwa, T. J. Clausen, G. R. Bretzel, M. Brownlee and D. E. Schleicher, Diabetologia, 1999, 42, 728–736 CrossRef CAS PubMed.
- B. O. Boehm, S. Schilling, S. Rosinger, G. E. Lang, G. K. Lang, R. Kientsch-Engel and P. Stahl, Diabetologia, 2004, 47, 1376–1379 CrossRef CAS PubMed.
- D. K. Karumanchi, N. Karunaratne, L. Lurio, J. P. Dillon and E. R. Gaillard, Amino Acids, 2015, 47, 2601–2608 CrossRef CAS PubMed.
- K. Sugimoto, M. Yasujima and S. Yagihashi, Curr. Pharm. Des., 2008, 14, 953–961 CrossRef CAS PubMed.
- H. Vlassara, M. Brownlee and A. Cerami, J. Exp. Med., 1984, 160, 197–207 CrossRef CAS PubMed.
- A. M. Vincent, J. W. Russell, P. Low and E. L. Feldman, Endocr. Rev., 2004, 25, 612–628 CrossRef CAS PubMed.
- B. Bouma, L. M. Kroon-Batenburg, Y. P. Wu, B. Brunjes, G. Posthuma, O. Kranenburg, P. G. de Groot, E. E. Voest and M. F. Gebbink, J. Biol. Chem., 2003, 278, 41810–41819 CrossRef CAS PubMed.
- X. H. Li, L. L. Du, X. S. Cheng, X. Jiang, Y. Zhang, B. L. Lv, R. Liu, J. Z. Wang and X. W. Zhou, Cell Death Dis., 2013, 4, e673 CrossRef CAS PubMed.
- N. Sasaki, R. Fukatsu, K. Tsuzuki, Y. Hayashi, T. Yoshida, N. Fujii, T. Koike, I. Wakayama, R. Yanagihara, R. Garruto, N. Amano and Z. Makita, Am. J. Pathol., 1998, 153, 1149–1155 CrossRef CAS PubMed.
- G. Münch, B. Westcott, T. Menini and A. Gugliucci, Amino Acids, 2010, 42, 1221–1236 CrossRef PubMed.
- E. Guerrero, P. Vasudevaraju, M. L. Hegde, G. B. Britton and K. S. Rao, Mol. Neurobiol., 2012, 47, 525–536 CrossRef PubMed.
- V. Jakus and N. Rietbrock, Physiol. Res., 2004, 53, 131–142 CAS.
- S. Yamagishi, K. Nakamura and T. Imaizumi, Curr. Diabetes Rev., 2005, 1, 93–106 CrossRef CAS PubMed.
- C. G. Schalkwijk and T. Miyata, Amino Acids, 2012, 42, 1193–1204 CrossRef CAS PubMed.
- A. Simm, J. Proteomics, 2013, 92, 248–259 CrossRef CAS PubMed.
- Z. Makita, S. Radoff, E. J. Rayfield, Z. Yang, E. Skolnik, V. Delaney, E. A. Friedman, A. Cerami and H. Vlassara, N. Engl. J. Med., 1991, 325, 836–842 CrossRef CAS PubMed.
- M. C. Thomas, J. M. Forbes and M. E. Cooper, Am. J. Therapeut., 2005, 12, 562–572 CrossRef.
- M. Kalousova, T. Zima, P. Popov, P. Spacek, M. Braun, J. Soukupova, K. Pelinkova and R. Kientsch-Engel, Alcohol Alcohol., 2004, 39, 316–320 CrossRef CAS PubMed.
- T. Miyata, Y. Wada, Z. Cai, Y. Iida, K. Horie, Y. Yasuda, K. Maeda, K. Kurokawa and C. van Ypersele de Strihou, Kidney Int., 1997, 51, 1170–1181 CrossRef CAS PubMed.
- S. K. Mallipattu and J. Uribarri, Curr. Opin. Nephrol. Hypertens., 2014, 23, 547–554 CrossRef CAS PubMed.
- H. Vlassara, Ann. Med., 1996, 28, 419–426 CrossRef CAS PubMed.
- G. Basta, A. M. Schmidt and R. De Caterina, Cardiovasc. Res., 2004, 63, 582–592 CrossRef CAS PubMed.
- P. Gkogkolou and M. Böhm, Derm.-Endocrinol., 2012, 4, 259–270 CrossRef CAS PubMed.
- H. Hideyuki and Y. Sho-ichi, Curr. Pharm. Des., 2008, 14, 969–972 CrossRef.
- H. Sharaf, S. Matou-Nasri, Q. Wang, Z. Rabhan, H. Al-Eidi, A. Al Abdulrahman and N. Ahmed, Biochim. Biophys. Acta, Mol. Basis Dis., 2015, 1852, 429–441 CrossRef CAS PubMed.
- P. B. Pun and M. P. Murphy, Int. J. Cell Biol., 2012, 843505, 21 Search PubMed.
- P. A. Cloos and S. Christgau, Matrix Biol., 2002, 21, 39–52 CrossRef CAS PubMed.
- P. C. Cloos and S. Christgau, Biogerontology, 2004, 5, 139–158 CrossRef CAS PubMed.
- F. W. Danby, Clin. Dermatol., 2010, 28, 409–411 CrossRef PubMed.
- J. A. Miller, E. Gravallese and H. F. Bunn, J. Clin. Invest., 1980, 65, 896–901 CrossRef CAS PubMed.
- A. C. Boyd, Y. H. A. Abdel-Wahab, A. M. McKillop, H. McNulty, C. R. Barnett, F. P. M. O'Harte and P. R. Flatt, Biochim. Biophys. Acta, Gen. Subj., 2000, 1523, 128–134 CrossRef CAS.
- S. Sen, M. Kar, A. Roy and A. S. Chakraborti, Biophys. Chem., 2005, 113, 289–298 CrossRef CAS PubMed.
- B. Hiller and P. C. Lorenzen, Food Res. Int., 2010, 43, 1155–1166 CrossRef CAS.
- A. Lapolla, D. Fedele, R. Reitano, N. C. Aricò, R. Seraglia, P. Traldi, E. Marotta and R. Tonani, J. Am. Soc. Mass Spectrom., 2004, 15, 496–509 CrossRef CAS PubMed.
- M. Wuhrer, A. M. Deelder and C. H. Hokke, J. Chromatogr. B: Anal. Technol. Biomed. Life Sci., 2005, 825, 124–133 CrossRef CAS PubMed.
- A. Lapolla, D. Fedele, R. Seraglia and P. Traldi, Mass Spectrom. Rev., 2006, 25, 775–797 CrossRef CAS PubMed.
- Q. Zhang, J. M. Ames, R. D. Smith, J. W. Baynes and T. O. Metz, J. Proteome Res., 2009, 8, 754–769 CrossRef CAS PubMed.
- O. S. Barnaby, C. Wa, R. L. Cerny, W. Clarke and D. S. Hage, Clin. Chim. Acta, 2010, 411, 1102–1110 CrossRef CAS PubMed.
- D. C. Carter, X. M. He, S. H. Munson, P. D. Twigg, K. M. Gernert, M. B. Broom and T. Y. Miller, Science, 1989, 244, 1195–1198 CAS.
- D. C. Carter and J. X. Ho, Adv. Protein Chem., 1994, 45, 153–203 CrossRef CAS PubMed.
- X. M. He and D. C. Carter, Nature, 1992, 358, 209–215 CrossRef CAS PubMed.
- T. Peters Jr, in All About Albumin, Academic Press, San Diego, 1995, pp. 9–75 Search PubMed.
- A. Bujacz, Acta Crystallogr., Sect. D: Biol. Crystallogr., 2012, 68, 1278–1289 CrossRef CAS PubMed.
- S. Sugio, A. Kashima, S. Mochizuki, M. Noda and K. Kobayashi, Protein Eng., 1999, 12, 439–446 CrossRef CAS PubMed.
- S. Curry, H. Mandelkow, P. Brick and N. Franks, Nat. Struct. Biol., 1998, 5, 827–835 CrossRef CAS PubMed.
- G. Sudlow, D. J. Birkett and D. N. Wade, Mol. Pharmacol., 1975, 11, 824–832 CAS.
- G. Sudlow, D. J. Birkett and D. N. Wade, Mol. Pharmacol., 1976, 12, 1052–1061 CAS.
- J. Ghuman, P. A. Zunszain, I. Petitpas, A. A. Bhattacharya, M. Otagiri and S. Curry, J. Mol. Biol., 2005, 353, 38–52 CrossRef CAS PubMed.
- H. Lin, J. Lan, M. Guan, F. Sheng and H. Zhang, Spectrochim. Acta, Part A, 2009, 73, 936–941 CrossRef PubMed.
- P. N. Naik, S. A. Chimatadar and S. T. Nandibewoor, Spectrochim. Acta, Part A, 2009, 73, 841–845 CrossRef CAS PubMed.
- A. Sułkowska, J. Mol. Struct., 2002, 614, 227–232 CrossRef.
- M. Fasano, S. Curry, E. Terreno, M. Galliano, G. Fanali, P. Narciso, S. Notari and P. Ascenzi, IUBMB Life, 2005, 57, 787–796 CrossRef CAS PubMed.
- S. Curry, Drug Metab. Pharmacokinet., 2009, 24, 342–357 CrossRef CAS PubMed.
- V. M. Rosenoer, in Albumin: Structure, Function and Uses, Pergamon, 1977, pp. 345–367 Search PubMed.
- J. Figge, T. H. Rossing and V. Fencl, J. Lab. Clin. Med., 1991, 117, 453–467 CAS.
- G. Fanali, A. di Masi, V. Trezza, M. Marino, M. Fasano and P. Ascenzi, Mol. Aspects Med., 2012, 33, 209–290 CrossRef CAS PubMed.
- I. Matei, S. Ionescu and M. Hillebrand, Spectrochim. Acta, Part A, 2012, 96, 709–715 CrossRef CAS PubMed.
- S. Tabassum, W. M. Al-Asbahy, M. Afzal and F. Arjmand, J. Photochem. Photobiol., B, 2012, 114, 132–139 CrossRef CAS PubMed.
- Y. Sameena, N. Sudha, S. Chandrasekaran and I. M. V. Enoch, J. Biol. Phys., 2014, 40, 347–367 CrossRef CAS PubMed.
- P. Caironi, T. Langer and L. Gattinoni, Curr. Opin. Crit. Care, 2015, 21, 302–308 CrossRef PubMed.
- I. Nowak and L. M. Shaw, Clin. Chem., 1995, 41, 1011–1017 CAS.
- Z. M. Wang, J. X. Ho, J. R. Ruble, J. Rose, F. Ruker, M. Ellenburg, R. Murphy, J. Click, E. Soistman, L. Wilkerson and D. C. Carter, Biochim. Biophys. Acta, 2013, 12, 6 Search PubMed.
- K. Yamasaki, V. T. Chuang, T. Maruyama and M. Otagiri, Biochim. Biophys. Acta, 2013, 12, 10 Search PubMed.
- A. A. Bhattacharya, S. Curry and N. P. Franks, J. Biol. Chem., 2000, 275, 38731–38738 CrossRef CAS PubMed.
- I. Petitpas, C. E. Petersen, C. E. Ha, A. A. Bhattacharya, P. A. Zunszain, J. Ghuman, N. V. Bhagavan and S. Curry, Proc. Natl. Acad. Sci. U. S. A., 2003, 100, 6440–6445 CrossRef CAS PubMed.
- P. A. Zunszain, J. Ghuman, T. Komatsu, E. Tsuchida and S. Curry, BMC Struct. Biol., 2003, 3, 7 CrossRef PubMed.
- F. Zsila, Mol. Pharm., 2013, 10, 1668–1682 CrossRef CAS PubMed.
- S. Watanabe, T. Tani and M. Seno, Biochim. Biophys. Acta, 1991, 4, 275–284 CrossRef.
- G. L. Tritsch, C. E. Rathke, N. E. Tritsch and C. M. Weiss, J. Biol. Chem., 1961, 236, 3163–3167 CAS.
- I. Petitpas, A. A. Bhattacharya, S. Twine, M. East and S. Curry, J. Biol. Chem., 2001, 276, 22804–22809 CrossRef CAS PubMed.
- W. Bal, M. Sokołowska, E. Kurowska and P. Faller, Biochim. Biophys. Acta, Gen. Subj., 2013, 1830, 5444–5455 CrossRef CAS PubMed.
- H. A. Saroff and M. S. Lewis, J. Phys. Chem., 1963, 67, 1211–1216 CrossRef CAS.
- C. B. Berde, B. S. Hudson, R. D. Simoni and L. A. Sklar, J. Biol. Chem., 1979, 254, 391–400 CAS.
- B. Sengupta and P. K. Sengupta, Biochem. Biophys. Res. Commun., 2002, 299, 400–403 CrossRef CAS PubMed.
- J. Kang, Y. Liu, M.-X. Xie, S. Li, M. Jiang and Y.-D. Wang, Biochim. Biophys. Acta, Gen. Subj., 2004, 1674, 205–214 CrossRef CAS PubMed.
- J. Min, X. Meng-Xia, Z. Dong, L. Yuan, L. Xiao-Yu and C. Xing, J. Mol. Struct., 2004, 692, 71–80 CrossRef CAS.
- M.-X. Xie, X.-Y. Xu and Y.-D. Wang, Biochim. Biophys. Acta, Gen. Subj., 2005, 1724, 215–224 CrossRef CAS PubMed.
- H.-X. Zhang, X. Huang, P. Mei, K.-H. Li and C.-N. Yan, J. Fluoresc., 2006, 16, 287–294 CrossRef CAS PubMed.
- Y.-Q. Wang, H.-M. Zhang, G.-C. Zhang, W.-H. Tao, Z.-H. Fei and Z.-T. Liu, J. Pharm. Biomed. Anal., 2007, 43, 1869–1875 CrossRef CAS PubMed.
- J. Xiao, J. Shi, H. Cao, S. Wu, F. Ren and M. Xu, J. Pharm. Biomed. Anal., 2007, 45, 609–615 CrossRef CAS PubMed.
- S. N. Khan, B. Islam, R. Yennamalli, A. Sultan, N. Subbarao and A. U. Khan, Eur. J. Pharm. Sci., 2008, 35, 371–382 CrossRef CAS PubMed.
- N. Wang, L. Ye, F. Yan and R. Xu, Int. J. Pharm., 2008, 351, 55–60 CrossRef CAS PubMed.
- Y.-J. Hu, H.-L. Yue, X.-L. Li, S.-S. Zhang, E. Tang and L.-P. Zhang, J. Photochem. Photobiol., B, 2012, 112, 16–22 CrossRef CAS PubMed.
- S. Awasthi and N. T. Saraswathi, J. Biomol. Struct. Dyn., 2015, 1–9 Search PubMed.
- V. Sinisi, C. Forzato, N. Cefarin, L. Navarini and F. Berti, Food Chem., 2015, 168, 332–340 CrossRef CAS PubMed.
- S. Tayyab, M. S. Zaroog, S. R. Feroz, S. B. Mohamad and S. N. A. Malek, Int. J. Pharm., 2015, 491, 352–358 CrossRef CAS PubMed.
- Y. Kurono, I. Kushida, H. Tanaka and K. Ikeda, Chem. Pharm. Bull., 1992, 40, 2169–2172 CrossRef CAS PubMed.
- N. Dubois-Presle, F. Lapicque, M. H. Maurice, S. Fournel-Gigleux, J. Magdalou, M. Abiteboul, G. Siest and P. Netter, Mol. Pharmacol., 1995, 47, 647–653 CAS.
- A. Salvi, P. A. Carrupt, J. M. Mayer and B. Testa, Drug Metab. Dispos., 1997, 25, 395–398 CAS.
- Y. Sakurai, S.-F. Ma, H. Watanabe, N. Yamaotsu, S. Hirono, Y. Kurono, U. Kragh-Hansen and M. Otagiri, Pharm. Res., 2004, 21, 285–292 CrossRef CAS.
- N. Ahmed, D. Dobler, M. Dean and P. J. Thornalley, J. Biol. Chem., 2005, 280, 5724–5732 CrossRef CAS PubMed.
- F. Yang, C. Bian, L. Zhu, G. Zhao, Z. Huang and M. Huang, J. Struct. Biol., 2007, 157, 348–355 CrossRef CAS PubMed.
- Y. Wang, S. Wang and M. Huang, Curr. Pharm. Des., 2015, 21, 1831–1836 CrossRef CAS PubMed.
- J. Córdova, J. D. Ryan, B. B. Boonyaratanakornkit and D. S. Clark, Enzyme Microb. Technol., 2008, 42, 278–283 CrossRef.
- H. Watanabe, S. Tanase, K. Nakajou, T. Maruyama, U. Kragh-Hansen and M. Otagiri, Biochem. J., 2000, 3, 813–819 CrossRef.
- N. G. Watkins, S. R. Thorpe and J. W. Baynes, J. Biol. Chem., 1985, 260, 10629–10636 CAS.
- R. L. Garlick and J. S. Mazer, J. Biol. Chem., 1983, 258, 6142–6146 CAS.
- N. Iberg and R. Fluckiger, J. Biol. Chem., 1986, 261, 13542–13545 CAS.
- C. Wa, R. L. Cerny, W. A. Clarke and D. S. Hage, Clin. Chim. Acta, 2007, 385, 48–60 CrossRef CAS PubMed.
- A. Frolov and R. Hoffmann, Anal. Bioanal. Chem., 2010, 397, 2349–2356 CrossRef CAS PubMed.
- O. S. Barnaby, R. L. Cerny, W. Clarke and D. S. Hage, Clin. Chim. Acta, 2011, 412, 277–285 CrossRef CAS PubMed.
- J. Anguizola, R. Matsuda, O. S. Barnaby, K. S. Hoy, C. Wa, E. DeBolt, M. Koke and D. S. Hage, Clin. Chim. Acta, 2013, 425, 64–76 CrossRef CAS PubMed.
- C. E. Guthrow, M. A. Morris, J. F. Day, S. R. Thorpe and J. W. Baynes, Proc. Natl. Acad. Sci. U. S. A., 1979, 76, 4258–4261 CrossRef CAS.
- K. F. McFarland, E. W. Catalano, J. F. Day, S. R. Thorpe and J. W. Baynes, Diabetes, 1979, 28, 1011–1014 CrossRef CAS PubMed.
- P. Rondeau and E. Bourdon, Biochimie, 2011, 93, 645–658 CrossRef CAS PubMed.
- S. Awasthi, N. A. Murugan and N. T. Saraswathi, Mol. Pharm., 2015, 12, 3312–3322 CrossRef CAS PubMed.
- J. Baraka-Vidot, C. Planesse, O. Meilhac, V. Militello, J. van den Elsen, E. Bourdon and P. Rondeau, Biochemistry, 2015, 54, 3051–3062 CrossRef CAS PubMed.
- F. Yang, C. Bian, L. Zhu, G. Zhao, Z. Huang and M. Huang, J. Struct. Biol., 2007, 157, 348–355 CrossRef CAS PubMed.
- D. Buttar, N. Colclough, S. Gerhardt, P. A. MacFaul, S. D. Phillips, A. Plowright, P. Whittamore, K. Tam, K. Maskos, S. Steinbacher and H. Steuber, Bioorg. Med. Chem., 2010, 18, 7486–7496 CrossRef CAS PubMed.
- A. J. Ryan, J. Ghuman, P. A. Zunszain, C. W. Chung and S. Curry, J. Struct. Biol., 2011, 174, 84–91 CrossRef CAS PubMed.
- N. Shaklai, R. L. Garlick and H. F. Bunn, J. Biol. Chem., 1984, 259, 3812–3817 CAS.
- P. J. Coussons, J. Jacoby, A. McKay, S. M. Kelly, N. C. Price and J. V. Hunt, Free Radical Biol. Med., 1997, 22, 1217–1227 CrossRef CAS PubMed.
- K. Nakajou, H. Watanabe, U. Kragh-Hansen, T. Maruyama and M. Otagiri, Biochim. Biophys. Acta, 2003, 13, 2–3 Search PubMed.
- R. Kisugi, T. Kouzuma, T. Yamamoto, S. Akizuki, H. Miyamoto, Y. Someya, J. Yokoyama, I. Abe, N. Hirai and A. Ohnishi, Clin. Chim. Acta, 2007, 382, 59–64 CrossRef CAS PubMed.
- N. Sattarahmady, A. A. Moosavi-Movahedi, F. Ahmad, G. H. Hakimelahi, M. Habibi-Rezaei, A. A. Saboury and N. Sheibani, Biochim. Biophys. Acta, 2007, 6, 933–942 CrossRef PubMed.
- R. Khodarahmi, S. A. Karimi, M. R. Ashrafi Kooshk, S. A. Ghadami, S. Ghobadi and M. Amani, Spectrochim. Acta, Part A, 2012, 89, 177–186 CrossRef CAS PubMed.
- H. Cao, T. Chen and Y. Shi, Curr. Med. Chem., 2015, 22, 4–13 CrossRef CAS PubMed.
- V. E. Syakhovich, N. T. Saraswathi, M. Ruff, S. B. Bokut and D. Moras, Acta Crystallogr., Sect. F: Struct. Biol. Cryst. Commun., 2006, 62, 106–109 CrossRef CAS PubMed.
- M. W. Khan, Z. Rasheed, W. A. Khan and R. Ali, Biochemistry, 2007, 72, 146–152 Search PubMed.
- A. Mohamadi-Nejad, A. A. Moosavi-Movahedi, S. Safarian, M. H. Naderi-Manesh, B. Ranjbar, B. Farzami, H. Mostafavi, M. B. Larijani and G. H. Hakimelahi, Thermochim. Acta, 2002, 389, 141–151 CrossRef CAS.
- M. E. Obrenovich and V. M. Monnier, Sci. Aging Knowl. Environ., 2004, 2, pe3 Search PubMed.
- R. GhoshMoulick, J. Bhattacharya, S. Roy, S. Basak and A. K. Dasgupta, Biochim. Biophys. Acta, 2007, 2, 233–242 CrossRef PubMed.
- S. W. Vetter and V. S. Indurthi, Clin. Chim. Acta, 2011, 412, 2105–2116 CrossRef CAS PubMed.
- D. L. Mendez, R. A. Jensen, L. A. McElroy, J. M. Pena and R. M. Esquerra, Arch. Biochem. Biophys., 2005, 444, 92–99 CrossRef CAS PubMed.
- P. Rondeau, G. Navarra, F. Cacciabaudo, M. Leone, E. Bourdon and V. Militello, Biochim. Biophys. Acta, 2010, 4, 789–798 CrossRef PubMed.
- A. Guerin-Dubourg, A. Catan, E. Bourdon and P. Rondeau, Diabetes Metab., 2012, 38, 171–178 CAS.
- S. Chesne, P. Rondeau, S. Armenta and E. Bourdon, Biochimie, 2006, 88, 1467–1477 CrossRef CAS PubMed.
- M. Shahani, F. Daneshi-Mehr, R. Tadayon, B. Hoseinzade Salavati, A. R. Akbar Zadeh-Baghban, A. Zamanian and M. Rezaei-Tavirani, Iran. J. Pharm. Res., 2013, 12, 185–191 CAS.
- X. Bai, Z. Wang, C. Huang and L. Chi, Molecules, 2012, 17, 8782–8794 CrossRef CAS PubMed.
- P. Rondeau, S. Armenta, H. Caillens, S. Chesne and E. Bourdon, Arch. Biochem. Biophys., 2007, 460, 141–150 CrossRef CAS PubMed.
- Y. Wei, L. Chen, J. Chen, L. Ge and R. Q. He, BMC Cell Biol., 2009, 10, 1471–2121 CrossRef PubMed.
- S. Allarakha, P. Ahmad, M. Ishtikhar, M. S. Zaheer, S. S. Siddiqi, Moinuddin and A. Ali, IUBMB Life, 2015, 67, 338–347 CrossRef CAS PubMed.
- N. Sattarahmady, A. A. Moosavi-Movahedi, M. Habibi-Rezaei, S. Ahmadian, A. A. Saboury, H. Heli and N. Sheibani, Carbohydr. Res., 2008, 343, 2229–2234 CrossRef CAS PubMed.
- C. Iannuzzi, G. Irace and I. Sirangelo, Front. Mol. Biosci., 2014, 1, 9 Search PubMed.
- C. Dufour and O. Dangles, Biochim. Biophys. Acta, 2005, 18, 1–3 Search PubMed.
- H. G. Mahesha, S. A. Singh, N. Srinivasan and A. G. Rao, FEBS J., 2006, 273, 451–467 CrossRef CAS PubMed.
- A. Bolli, M. Marino, G. Rimbach, G. Fanali, M. Fasano and P. Ascenzi, Biochem. Biophys. Res. Commun., 2010, 398, 444–449 CrossRef CAS PubMed.
- M. Pesavento and A. Profumo, Talanta, 1991, 38, 1099–1106 CrossRef CAS PubMed.
- S. M. T. Shaikh, J. Seetharamappa, P. B. Kandagal, D. H. Manjunatha and S. Ashoka, Dyes Pigm., 2007, 74, 665–671 CrossRef CAS.
- N. Barbero, E. Barni, C. Barolo, P. Quagliotto, G. Viscardi, L. Napione, S. Pavan and F. Bussolino, Dyes Pigm., 2009, 80, 307–313 CrossRef CAS.
- D. V. Suresh, H. G. Mahesha, A. G. Rao and K. Srinivasan, Biopolymers, 2007, 86, 265–275 CrossRef CAS PubMed.
- Y. Q. Wang, H. M. Zhang, J. Cao and B. P. Tang, J. Photochem. Photobiol., B, 2014, 138, 182–190 CrossRef CAS PubMed.
- K. Koizumi, C. Ikeda, M. Ito, J. Suzuki, T. Kinoshita, K. Yasukawa and T. Hanai, Biomed. Chromatogr., 1998, 12, 203–210 CrossRef CAS PubMed.
- J. Baraka-Vidot, A. Guerin-Dubourg, E. Bourdon and P. Rondeau, Biochimie, 2012, 94, 1960–1967 CrossRef CAS PubMed.
- M. P. Cohen, Biochim. Biophys. Acta, 2013, 12, 23 CrossRef PubMed.
- Y. Wang, H. Yu, X. Shi, Z. Luo, D. Lin and M. Huang, J. Biol. Chem., 2013, 288, 15980–15987 CrossRef CAS PubMed.
- T. Enokiya, Y. Muraki, T. Iwamoto and M. Okuda, Int. J. Antimicrob. Agents, 2015, 46, 164–168 CrossRef CAS PubMed.
- J. Sabah, E. McConkey, R. Welti, K. Albin and L. J. Takemoto, Exp. Eye Res., 2005, 80, 31–36 CrossRef CAS PubMed.
- G. J. van der Vusse, Drug Metab. Pharmacokinet., 2009, 24, 300–307 CrossRef CAS PubMed.
- D. Blache, E. Bourdon, P. Salloignon, G. Lucchi, P. Ducoroy, J. M. Petit, B. Verges and L. Lagrost, Diabetes, 2015, 64, 960–972 CrossRef CAS PubMed.
- B. G. Stegmayr, New insight in impaired binding capacity for albumin in uraemic patients, Acta Physiol., 2015, 215(1), 5–8 CrossRef CAS PubMed.
- E. Yamazaki, M. Inagaki, O. Kurita and T. Inoue, J. Biosci., 2005, 30, 475–481 CrossRef CAS PubMed.
- E. Bourdon, N. Loreau and D. Blache, FASEB J., 1999, 13, 233–244 CAS.
- P. Faure, N. Wiernsperger, C. Polge, A. Favier and S. Halimi, Clin. Sci., 2008, 114, 251–256 CrossRef CAS PubMed.
- S. Y. Yang, Y. J. Chen, P. H. Kao and L. S. Chang, Arch. Biochem. Biophys., 2014, 564, 43–51 CrossRef CAS PubMed.
- C. M. Zheng, W. Y. Ma, C. C. Wu and K. C. Lu, Clin. Chim. Acta, 2012, 413, 1555–1561 CrossRef CAS PubMed.
- A. Singha Roy, P. Ghosh and S. Dasgupta, J. Biomol. Struct. Dyn., 2015, 30, 1–8 Search PubMed.
- W. Xu, L. Chen and R. Shao, Curr. Drug Metab., 2014, 15, 116–119 CrossRef CAS PubMed.
- F. N. Ziyadeh and M. P. Cohen, Mol. Cell. Biochem., 1993, 125, 19–25 CrossRef CAS PubMed.
- M. P. Cohen and F. N. Ziyadeh, Kidney Int., 1994, 45, 475–484 CrossRef CAS PubMed.
- S. Awasthi, S. K. Gayathiri, R. Ramya, R. Duraichelvan, A. Dhason and N. T. Saraswathi, Appl. Biochem. Biotechnol., 2015, 177, 1013–1024 CrossRef CAS PubMed.
- Y. Hattori, H. Kakishita, K. Akimoto, M. Matsumura and K. Kasai, Biochem. Biophys. Res. Commun., 2001, 281, 891–896 CrossRef CAS PubMed.
- Y. Hattori, M. Suzuki, S. Hattori and K. Kasai, Hypertension, 2002, 39, 22–28 CrossRef CAS PubMed.
- A. Amore, P. Cirina, S. Mitola, L. Peruzzi, B. Gianoglio, I. Rabbone, C. Sacchetti, F. Cerutti, C. Grillo and R. Coppo, Kidney Int., 1997, 51, 27–35 CrossRef CAS PubMed.
- A. M. Schmidt, C. Esposito, J. Brett, S. Ogawa, M. Clauss, M. Kirstein, S. Radoff, H. Vlassara and D. Stern, in Mononuclear Phagocytes, ed. R. van Furth, Springer, Netherlands, 1992, ch. 27, pp. 202–207 Search PubMed.
- N. A. Ansari, Moinuddin, K. Alam and A. Ali, Hum. Immunol., 2009, 70, 417–424 CrossRef CAS PubMed.
- N. A. Ansari and D. Dash, Aging and Disease, 2013, 4, 50–56 Search PubMed.
- N. A. Ansari, Moinuddin, A. R. Mir, S. Habib, K. Alam, A. Ali and R. H. Khan, Cell Biochem. Biophys., 2014, 70, 857–865 CrossRef CAS PubMed.
|
This journal is © The Royal Society of Chemistry 2016 |
Click here to see how this site uses Cookies. View our privacy policy here.