DOI:
10.1039/C6RA06532E
(Paper)
RSC Adv., 2016,
6, 42649-42655
Facile synthesis of superparamagnetic magnetite nanoflowers and their applications in cellular imaging†
Received
11th March 2016
, Accepted 21st April 2016
First published on 25th April 2016
Abstract
Clustering of biocompatible magnetite nanocrystals into secondary structures not only allows the combination of properties of individual crystals but also creates new collective properties by taking advantage of their electromagnetic interactions. In this work, we describe the synthesis of water-soluble biocompatible superparamagnetic magnetite nanoflowers (MNFs) by thermal decomposition of iron oleate with polyethylene glycol diacid as a capping ligand. These nanoflowers, dispersed in water at physiological pH, present particularly interesting magnetic properties. The structure and morphology of the MNFs were characterized by X-ray diffraction and transmission electron microscopy. In addition, confocal fluorescence microscopy was used to study the cellular uptake of the dye-functionalized nanoflowers in vitro. The combination of superparamagnetism, high magnetization, and high stability makes these MNFs ideal candidates for biomedical applications.
Introduction
There has been considerable interest in the development of a variety of magnetite (Fe3O4) nanomaterials that can be applied in biomedical applications, such as magnetic separation, drug delivery, cancer hyperthermia, and magnetic resonance imaging (MRI).1–5 In particular, superparamagnetic magnetite nanocrystals can be well stabilized in biological systems and be used as contrast agents for MRI, as they are not subject to strong magnetic interactions in dispersion.6,7 Exciting progress has been made in synthesizing magnetite nanocrystals with controlled sizes and shapes by thermal decomposition. However, these hydrophobic small magnetite nanocrystals have a low magnetization per particle after transformed into hydrophilic ones, so that it is difficult to separate them effectively from solution or control their movement in blood. Although the increase in the crystal size results in the increase of saturation magnetization, it induces superparamagnetic–ferromagnetic transition (at a domain size of 30 nm for Fe3O4) at the same time,8–10 so that nanocrystals are no longer suitable for biomedical applications. Recent efforts in nanocrystal synthesis have focused on development of the secondary structure manipulation, such as magnetite nanocrystal clusters (MNCs), which can upgrade the properties of individual nanocrystals due to interactions between the subunits.11–15 The common goal of these strategies was to control the size of MNCs because magnetic property and relaxivity increases are proportional to the size of nanocrystals.16,17 Generally, MNCs have been grown either by one-step direct solution growth11,12,18–23 or by multi-step synthetic paths utilizing pre-synthesized magnetite nanocrystals.24,25 The direct one-step processes seems to be a more simple, efficient and attractive approach, compared with those multi-step processes by assembling pre-synthesized nanocrystals into clusters of designed configurations in separate steps via methods such as solvent evaporation, electrostatic attraction or interfacial tension. However, the reaction conditions are difficult to control. An alternative approach was developed to achieve water-soluble magnetite nanocrystals by pyrolyzing iron(III) acetylacetonate (Fe(acac)3) in 2-pyrrolidone or phenyl ether in the presence of polyethylene glycol (PEG) via ‘one-pot’ reactions.26–30 Recently, functionalized magnetite nanoflowers as potential MRI contrast agents were synthesized using a rapid one-step continuous hydrothermal process.31 Though the iron sources were quite diverse, we hypothesized that at the high temperatures where pyrolysis occurred, iron carboxylate salts were the actual precursors to prepare water-soluble Fe3O4 nanomaterials via one-pot reactions.
Herein, we report a novel one-pot reaction to achieve highly water-soluble biocompatible superparamagnetic MNCs by thermal decomposition of inexpensive and non-toxic iron carboxylate precursors (iron oleate complex) with polyethylene glycol diacid (HOOC–PEG–COOH) as a capping ligand. Therefore, this approach follows the green chemistry principle by providing an environmentally and economically preferable alternative for preparing water-soluble superparamagnetic magnetite nanoflowers (MNFs). The grain size and cluster size of MNFs can be tuned by changing the amounts of iron oleate and PEG diacid or controlling the reaction time. Moreover, the carboxyl group on the surface of the MNFs makes them amenable to binding with fluorescent dyes for various biomedical applications.
Experimental
Chemicals
The following reagents were purchased from Sigma-Aldrich and were used as received: 1-ethyl-3-(3-dimethylaminopropyl) carbodiimide hydrochloride (EDC), N-hydroxysuccinimide (98%; NHS), poly(ethylene glycol) bis(carboxymethyl) ether (HOOC–PEG–COOH, PEG diacid, average Mn = 600), phenyl ether, iron chloride (98%), and rhodamine 123 (Rh123). Sodium oleate (95%) was purchased from TCI.
Synthesis of the superparamagnetic magnetite nanoflowers (MNFs)
The iron oleate precursor was prepared by reacting sodium oleate and iron chloride hexahydrate according to the reported methods.32 Typically, the 62 nm MNFs were synthesized by heating a mixture of 1 mmol iron-oleate complex, 3 mmol PEG diacid (Mn = 600 Da), and 5 g phenyl ether at 200 °C for 30 min and then at 260 °C for another 2 h. Upon addition of a mixture of ether and ethanol (vol/vol = 5
:
1) into the reaction solution at room temperature, the resultant nanoflowers were precipitated and isolated. By being redispersed in ethanol and subsequently precipitated with ether for three cycles, the nanocrystals were purified and collected for further characterizations. The mean diameter of the resultant MNFs decreases to 25 nm when the ratio of iron-oleate to PEG diacid changes to 1
:
4, and heated at 260 °C for 5 h. Subsequently, the 25 nm MNFs were obtained after a set of post-processing procedures that were the same as those for the 62 nm MNFs.
Surface functionalization of the MNFs with fluorescent dye rhodamine 123
The fluorescent dye Rh123 was bound to the surface of the MNFs for linking this dye to a carboxyl group. Briefly, 1 mL of as-synthesized MNFs in aqueous solution (2 mg mL−1) was mixed with 76 mg (0.4 mmol) of EDC and 26 mg (0.2 mmol) of NHS followed by 0.5 mL DMSO solution containing 9 mg (0.025 mmol) of Rh123 dye, and the reaction vial was vortexed for 4 h. The resulting dye-conjugated nanoflowers were washed several times through alternate cycles of sonication and spin filtration (Mw cutoff of 10 kDa, 5000 × g, 10 min, Millipore) to remove any unbound Rh123 dye.
In vitro cytotoxicity assay
HeLa cells were cultured in Dulbecco's modified eagle's medium supplemented with 10% (v/v) fetal bovine serum (BioWhittaker, Walkersville, MD, USA) at 37 °C in a humidified atmosphere containing 5% CO2. The cell viability was assessed using a 3-(4,5-dimethylthiazol-2-yl)-2,5-diphenyltetrazolium bromide (MTT) assay. The cells were seeded into 96-well plates for 12 h at a density of 8 × 103 cells per well at 37 °C in 5% CO2 atmosphere. Samples at various concentrations were added to the cells and incubated for 24 h. The cells were washed twice with PBS and replenished with fresh medium. Subsequently, the cells were examined by a MTT assay.
Confocal laser scanning microscopy (CLSM) characterization
HeLa cells in culture medium were incubated with Rh123-conjugated MNFs (30 μL MNFs/1 mL culture medium) at 37 °C for 30 min. After removal of the media with Rh123-conjugated MNFs, fresh culture medium was added. The specimens were then examined by confocal laser scanning microscopy using a 488 nm laser (FV5-LAMAR, Olympus, Japan). The fluorescence of Rh123-conjugated MNFs was highlighted in green. The MitoTracker red stained cells were examined by CLSM using a 559 nm laser.
Characterization
Fourier transformed infrared (FTIR) spectra were recorded on a Thermo IR200 spectrometer. Transmission electron microscopy (TEM) images were acquired using a JEOL JEM-2100 operating at 100 kV. Samples for TEM and high resolution TEM analysis were prepared by spreading a drop of the sample solution on copper grids coated with a carbon film followed by evaporation under ambient conditions. Powder X-ray diffraction (XRD) patterns were measured using a XRD-6100 diffractometer (Shimadzu) with Cu Kα radiation (λ = 1.5406 Å). Dynamic light scattering (DLS) measurements were carried out at 25 °C with a Nano ZS (Malvern) after the MNFs aqueous solutions were filtered through a 220 nm syringe filter. Thermogravimetric analysis (TGA) of the dried sample was carried out on a DTG-60AH (Shimadzu). The sample weight was monitored in the temperature range of 25 to 900 °C with a heating rate of 10 °C min−1 under air flow. Magnetic properties were measured using a vibrating sample magnetometer (VSM, HH-20, Nanjing Nanda instrument). UV-vis absorption spectra and fluorescent spectra were recorded at room temperature with a Cary 60 UV-vis spectrophotometer and a Cary Eclipse fluorescence spectrophotometer, respectively.
Results and discussion
Scheme 1 summarizes the synthetic route from the iron oleate precursor to the magnetite nanoflowers through the thermal decomposition process. The iron-oleate complex was prepared by reacting environmentally friendly compounds, namely iron chloride and sodium oleate. PEG diacid was selected because of the strong coordination of carboxylate groups with iron cations on the Fe3O4 surfaces and its anti-biofouling properties.
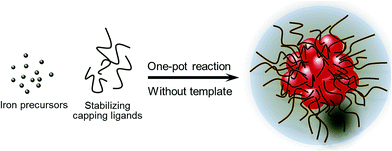 |
| Scheme 1 Schematic illustration of the preparation strategies for highly water-soluble biocompatible superparamagnetic magnetite nanoflowers. | |
The structure and morphology of MNFs were investigated by TEM. Fig. 1 shows the representative TEM images of MNFs with different grain sizes and cluster sizes. The secondary structure of the MNFs can be observed more clearly in Fig. 1a and b (inset) for isolated MNFs approximately 25 ± 5 and 62 ± 12 nm in size. In particular, the rough surface of clusters and interspace among the primary nanocrystals suggested that the secondary structure was built up by subunit grains. The 25 and 62 nm MNFs is composed of small primary magnetite nanocrystals with sizes of about 4.7 and 6.3 nm, respectively. Lattice fringes were recorded for a small nanoflower with size of 25 nm, as shown in the high resolution TEM (HRTEM) image in Fig. 1c. The lattice spacing between two adjacent planes is 0.485 nm, corresponding to the distance between two (111) planes in magnetite.17 The selected area electron diffraction (SAED) pattern recorded on the 62 nm MNFs reveals single-crystal-like diffraction (Fig. 1d). The diffraction spots are widened into narrow arcs that indicate slight misalignments among the primary nanocrystals. When the amount of stabilizing ligand increased, more PEG will bind to the surface of primary Fe3O4 nanocrystals through the strong coordinative interactions. This results in a decrease of the grain size of Fe3O4. Consequently, the main driving force for the aggregation of primary magnetite nanocrystals is generally attributed to the reduced high surface energy through both the attachment the primary nanocrystals and PEG. The aggregation of primary magnetite nanocrystals led to the formation of larger magnetite nanoflowers under the condition of high nucleus concentration and by reducing the amount of stabilizing capping ligands. In addition, the intermediate clusters underwent compaction and gradually became denser in 2 h, which finally evolved into more regular and monodisperse MNFs (Fig. S1, ESI†).
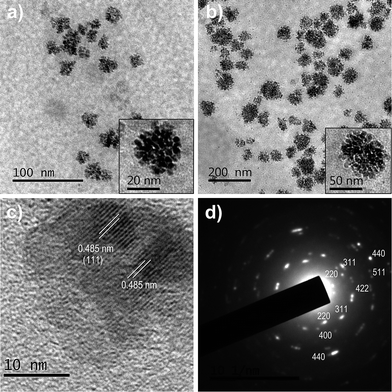 |
| Fig. 1 TEM images of (a) 25 nm and (b) 62 nm MNFs. Insets are high-magnification TEM images. (c) Typical HRTEM image of a 25 nm nanoflower. (d) SAED pattern of the nanoflower in (b). | |
The crystalline structure of 25 and 62 nm MNFs was also characterized by the powder X-ray diffraction (XRD) measurements. As shown in Fig. 2, position and relative intensity of all diffraction peaks match well with those from the JCPDS card (19-0629) for magnetite. The average sizes of the primary magnetite nanocrystals of 25 and 62 nm MNFs deduced from Debye–Scherrer formula are 4.8 and 6.6 nm, respectively, which are in good agreement with the result obtained from the TEM observation of the same samples.
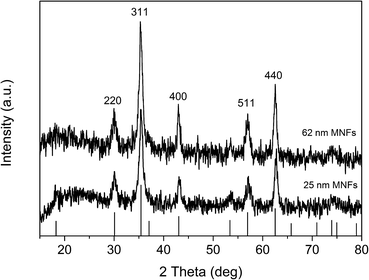 |
| Fig. 2 X-ray diffraction pattern of 25 nm and 62 nm MNFs. Bars: JCPDS card (19-0629) data for magnetite. | |
The unique and complex structure allows the MNFs to retain superparamagnetic behavior at room temperature even though their size exceeds 30 nm, whereas single-crystalline magnetite particle within the same size range would exhibit ferromagnetic behaviour. Fig. 3 shows hysteresis loops of all the MNFs measured at 300 K, respectively. The MNFs show no remanence or coercivity at 300 K, that is, superparamagnetic behavior. The saturation magnetization (Ms) was determined to be 21.9 and 56.6 emu g−1 for 25 and 62 nm MNFs, respectively. The values decrease noticeably for the MNFs prepared under a 1
:
4 molar ratio of iron-oleate and PEG diacid, which may be attributed to the higher weight fraction of PEG coating and/or a surface related effect such as surface disorder. By taking into account that these samples contain 55.9 and 30.8% organic composition according to the TGA results (Fig. 4), the Ms values of 25 and 62 nm MNFs without organic composition are calculated as 49.8 and 81.8 emu g−1 of pure Fe3O4, respectively. This trend is also consistent with that of the size of primary nanocrystals in the MNFs. The Ms value of both nanoflower types is larger than that of the MNFs prepared by decomposition of Fe(acac)3.
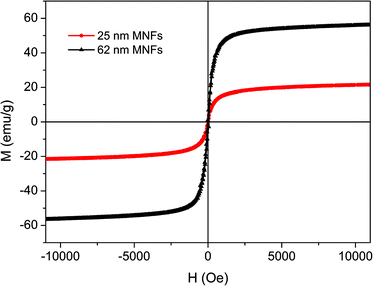 |
| Fig. 3 Magnetization (M) loops measured at room temperature for 25 nm and 62 nm MNFs. | |
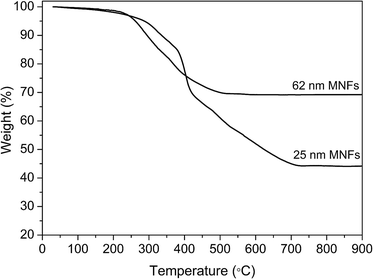 |
| Fig. 4 TGA curves of 25 nm and 62 nm MNFs. | |
The surface chemical structure of the MNFs was characterized by Fourier-transform infrared (FTIR) spectroscopy (Fig. 5). The characteristic bands of PEG diacid at 1107 cm−1 (C–O–C stretch) and 1455 cm−1 (CH2 scissor) appear in the spectrum of the MNFs, confirming the existence of PEG on the Fe3O4 surface.33 The intensity of the absorption peak at 1737 cm−1 originating from the stretching vibration of the COOH groups decreased, and that of the absorption peak at around 1630 cm−1 increased significantly in the spectrum of the MNFs compared to the spectrum of pure PEG diacid. The absorption peak of the C
O band of carboxyl group at 1737 cm−1 is shifted to a lower wavenumber, 1630 cm−1, when the binding between the carboxylate group and metal on Fe3O4 occurred, transforming a carboxylic acid functionality to a carboxylate functionality with both oxygen atoms interacting with the metal on the surface.30,34,35 It indicates that the one carboxyl group on PEG diacid is intimately bound to Fe3O4 surface, leaving another carboxyl end group exposed to the environment. The strong band at 580 cm−1 is the characteristic absorption of the Fe–O bond of magnetite.
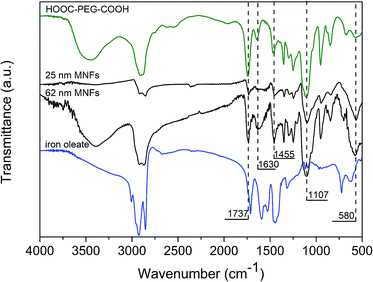 |
| Fig. 5 FT-IR spectra of 25 nm MNFs, 62 nm MNFs, PEG diacid, and iron-oleate complex. | |
The MNFs are highly water dispersible, even after washing three times with ethanol/ether, attributing to the robust surface coating of PEG. Dynamic light scattering (DLS) measurements of 25 and 62 nm MNFs show that their hydrodynamic sizes are 37 ± 9 and 88 ± 2 nm, respectively. Fig. 6 reveals that the nanoflowers in water exhibit a single scattering peak that remained unchanged after stored at 5 °C for 20 days. The zeta potentials of 25 and 62 nm MNFs are −21.7 and −26.5 mV, respectively, which indicates the existence of carboxylic groups on the surface of MNFs. The MNFs were also shown to be stable at pH 4–12 and at a high NaCl salt concentration (2.5 M).
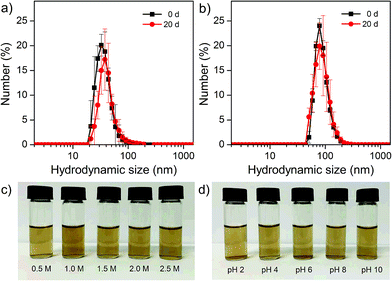 |
| Fig. 6 Hydrodynamic size profiles of (a) 25 nm and (b) 62 nm MNFs and the same samples after being stored over 20 days. Colloidal stability tests of 62 nm MNFs in NaCl solutions (c) and at several pH (d). | |
The presence of the terminal carboxyl groups on the surface of MNFs makes this material a suitable substrate for conjugation with biomarkers and bioactive molecules, as demonstrated by linking rhodamine-123 (Rh123) to the surface. The absorption and fluorescence spectra of Rh123-tagged 62 nm MNFs are presented in Fig. 7 and 8. The absorbance of Rh123 is roughly superimposed on that of the MNFs, revealing an enhanced absorbance for the Rh123-conjugated nanoflowers. With 500 nm excitation, a clear peak from the Rh123 conjugated MNFs is observed at 522 nm. In this case, the Rh123 conjugated MNFs retain their fluorescence after washing (by adding a mixture of deionized water and DMSO followed by centrifugation, and dialysis). By this washing procedure, all the free Rh123 dye has been removed. For the purpose of control, when the Rh123 was simply mixed with a MNFs sample, it also exhibited fluorescence because of the free Rh123 in the environment of the MNFs dispersion. However, the free Rh123 is washed away by spin filtering and dialysis, and the washed sample does not show any fluorescence (Fig. S2 and S3, ESI†). The binding of the Rh123 to the MNFs surface causes a minor blue shift (∼4 nm) of the maximum of the fluorescence band.36 This test demonstrates that the dye is indeed bound to the surface of MNFs.
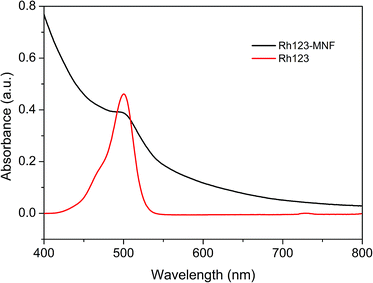 |
| Fig. 7 UV-vis absorption spectra of Rh123 and Rh123-conjugated MNFs after purified by spin filtering. | |
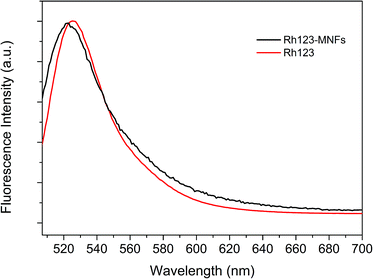 |
| Fig. 8 Fluorescence spectra of Rh123 and Rh123-conjugated MNFs after purified by spin filtering. | |
Biocompatibility of the obtained MNFs is a very important factor in biomedical applications. The cytotoxicity was investigated by incubating the MNFs with HeLa cells and was assessed by means of a 3-(4,5-dimethylthiazol-2-yl)-2,5-diphenyltetrazolium bromide (MTT) assay. As shown in Fig. 9, the MNFs showed no appreciable toxicity toward the cells, confirming that the MNFs are compatible for biomedical applications.
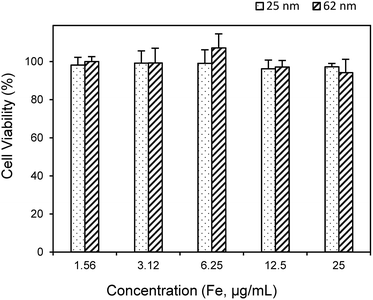 |
| Fig. 9 Cytotoxicity of 25 and 62 nm MNFs toward the HeLa cells after 12 h incubation. | |
To demonstrate the imaging capability of the fluorescently labeled MNFs for optical tracking in vitro, we incubated HeLa cells with Rh123-conjugated MNFs. The fluorescence images of the Rh123-conjugated MNFs (Fig. 4a) demonstrate that the fluorescent MNFs are taken up efficiently by the cells. Moreover, the fluorescence image of HeLa cells stained with Rh123-conjugated MNFs is similar to that of cells stained with free Rh123 and MitoTracker red (Fig. 10). Rh123 and MitoTracker red are well-known stains for mitochondria. Thus, the Rh123 molecules conjugated to the surface of the MNFs retain their ability to interact with the surface of mitochondria, as free dyes do. Therefore, the obtained MNFs could be used as biomaterials simultaneously possessing MR and fluorescence imaging ability. Furthermore, this suggests that other molecules can be bound to the surface of the MNFs without significant degradation of their functionality.
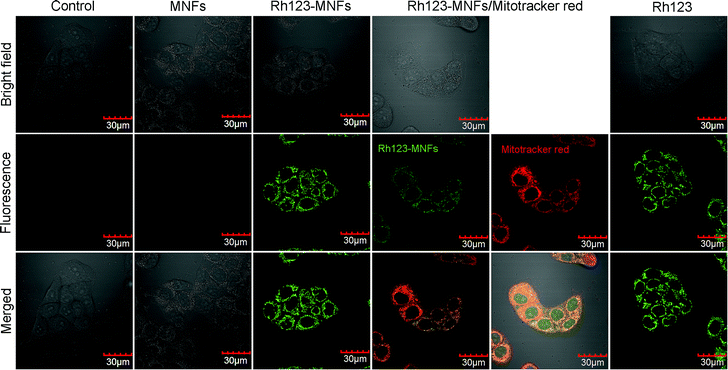 |
| Fig. 10 Confocal micrographs of HeLa cells stained with MNFs, Rh123-conjugated MNFs, mitochondria dye MitoTracker red, and Rh123. The cells in culture medium were incubated with samples at 37 °C for 30 min. The specimens were examined by CLSM using a 488 nm laser for Rh123 and Rh123-conjugated MNFs, and 559 nm for MitoTracker red. | |
Conclusions
In conclusion, water-soluble, biocompatible, and superparamagnetic magnetite nanoflowers were synthesized via a one-pot reaction by thermal decomposition iron-oleate with PEG diacid as a surfactant. The abundant carboxylic groups on the MNFs surface provide an avenue to extend bond formation with fluorescent dyes and other bioactive molecules so that specific targeting within biological systems can be facilitated. We have shown that Rh123 dye can be conjugated to the MNFs surface and still retains its fluorescence. These fluorescent MNFs can be taken up by cultured cells in vitro and optically tracked using confocal laser scanning fluorescence microscopy. Thus the prepared MNFs exhibit high potential as MRI and fluorescence imaging materials. The overall synthetic process is quite simple and inexpensive, and is readily applicable to the large-scale preparation of water-soluble and biocompatible MNFs for biomedical applications.
Acknowledgements
This work was supported by National Natural Science Foundation of China (21401008), Beijing Excellent Talent Training Project (2013D005003000007), BMEC (SQKM201610011007), and the Project-sponsored by SRF for ROCS, SEM.
Notes and references
- H. Zeng, J. Li, J. P. Liu, Z. L. Wang and S. Sun, Nature, 2002, 420, 395 CrossRef CAS PubMed.
- E. Amstad, M. Textora and E. Reimhult, Nanoscale, 2011, 3, 2819 RSC.
- O. L. Gobbo, K. Sjaastad, M. W. Radomski, Y. Volkov and A. Prina-Mello, Theranostics, 2015, 5, 1249 CrossRef PubMed.
- B. H. Kim, N. Lee, H. Kim, K. An, Y. I. Park, Y. Choi, K. Shin, Y. Lee, S. G. Kwon, H. B. Na, J.-G. Park, T.-Y. Ahn, Y.-W. Kim, W. K. Moon, S. H. Choi and T. Hyeon, J. Am. Chem. Soc., 2011, 133, 12624 CrossRef CAS PubMed.
- H. Marie, L. Lemaire, F. Franconi, S. Lajnef, Y.-M. Frapart, V. Nicolas, G. Frébourg, M. Trichet, C. Ménager and S. Lesieur, Adv. Funct. Mater., 2015, 25, 1258 CrossRef CAS.
- J. Zeng, L. Jing, Y. Hou, M. Jiao, R. Qiao, Q. Jia, C. Liu, F. Fang, H. Lei and M. Gao, Adv. Mater., 2014, 26, 2694 CrossRef CAS PubMed.
- C. Lu, M. K. Park, C. Lu, Y. H. Lee and K. Y. Chai, J. Mater. Chem. B, 2015, 3, 3730 RSC.
- J. Lee, T. Isobe and M. Senna, J. Colloid Interface Sci., 1996, 177, 490 CrossRef CAS.
- J. Gass, P. Poddar, J. Almand, S. Srinath and H. Srikanth, Adv. Funct. Mater., 2006, 16, 71 CrossRef CAS.
- C. Lu, Z.-S. Quan, J. C. Sur, S.-H. Kim, C. H. Lee and K. Y. Chai, New J. Chem., 2010, 34, 2040 RSC.
- Y. Zhu, W. Zhao, H. Chen and J. Shi, J. Phys. Chem. C, 2007, 111, 5281 CAS.
- M. Lin, H. Huang, Z. Liu, Y. Liu, J. Ge and Y. Fang, Langmuir, 2013, 29, 15433 CrossRef CAS PubMed.
- H. Deng, X. Li, Q. Peng, X. Wang, J. Chen and Y. Li, Angew. Chem., Int. Ed., 2005, 44, 2782 CrossRef CAS PubMed.
- W. Ma, Y. Zhang, L. Li, L. You, P. Zhang, Y. Zhang, J. Li, M. Yu, J. Guo, H. Lu and C. Wang, ACS Nano, 2012, 6, 3179 CrossRef CAS PubMed.
- Z. Lu and Y. Yin, Chem. Soc. Rev., 2012, 41, 6874 RSC.
- S. B. Seo, J. Yang, T. I. Lee, C. H. Chung, Y. J. Song, J.-S. Suh, H. G. Yoon, Y. M. Huh and S. Haam, J. Colloid Interface Sci., 2008, 319, 429 CrossRef CAS PubMed.
- J. Ge, Y. Hu, M. Biasini, W. P. Beyermann and Y. Yin, Angew. Chem., Int. Ed., 2007, 46, 4342 CrossRef CAS PubMed.
- J. Liu, Z. Sun, Y. Deng, Y. Zou, C. Li, X. Guo, L. Xiong, Y. Gao, F. Li and D. Zhao, Angew. Chem., Int. Ed., 2009, 48, 5875 CrossRef CAS PubMed.
- J. Zhou, L. Meng, X. Feng, X. Zhang and Q. Lu, Angew. Chem., Int. Ed., 2010, 49, 8476 CrossRef CAS PubMed.
- F. Dong, W. Guo, J. Bae, S. Kim and C. Ha, Chem.–Eur. J., 2011, 17, 12802 CrossRef CAS PubMed.
- S. Xuan, Y.-X. J. Wang, J. C. Yu and K. C.-F. Leung, Chem. Mater., 2009, 21, 5079 CrossRef CAS.
- D. Li, J. Tang, J. Guo, S. Wang, D. Chaudhary and C. Wang, Chem.–Eur. J., 2012, 18, 16517 CrossRef CAS PubMed.
- A. Narayanaswamy, H. Xu, N. Pradhan and X. Peng, Angew. Chem., Int. Ed., 2006, 45, 5361 CrossRef CAS PubMed.
- J. Yang, C.-H. Lee, H.-J. Ko, J.-S. Suh, H.-G. Yoon, K. Lee, Y.-M. Huh and S. Haam, Angew. Chem., Int. Ed., 2007, 46, 8836 CrossRef CAS PubMed.
- A. Bakandritsos, G. Mattheolabakis, R. Zboril, N. Bouropoulos, J. Tucek, D. G. Fatouros and K. Avgoustakis, Nanoscale, 2010, 2, 564 RSC.
- Z. Li, L. Wei, M. Gao and H. Lei, Adv. Mater., 2005, 17, 1001 CrossRef CAS.
- Q. Jia, J. Zeng, R. Qiao, L. Jing, L. Peng, F. Gu and M. Gao, J. Am. Chem. Soc., 2011, 133, 19512 CrossRef CAS PubMed.
- F. Hu, L. Wei, Z. Zhou, Y. Ran, Z. Li and M. Gao, Adv. Mater., 2006, 18, 2553 CrossRef CAS.
- L. Wang, Q. Wu, S. Tang, J. Zeng, R. Qiao, P. Zhao, Y. Zhang, F. Hu and M. Gao, RSC Adv., 2013, 3, 23454 RSC.
- F. Hu, K. W. MacRenaris, E. A. Waters, E. A. Schultz-Sikma, A. L. Eckermann and T. J. Meade, Chem. Commun., 2010, 46, 73 RSC.
- G. Thomas, F. Demoisson, R. Chassagnon, E. Popva and N. Millot, Nanotechnology, 2016, 27, 135604 CrossRef CAS PubMed.
- J. Park, K. An, Y. Hwang, J. G. Park, H. J. Noh, J. Y. Kim, J. H. Park, N. M. Hwang and T. Hyeon, Nat. Mater., 2004, 3, 891 CrossRef CAS PubMed.
- C. Lu, L. R. Bhatt, H. Y. Jun, S. H. Park and K. Y. Chai, J. Mater. Chem., 2012, 22, 19806 RSC.
- Q. Liu and Z. Xu, Langmuir, 1995, 11, 4617 CrossRef CAS.
- J. Ge, Y. Hu, M. Biasini, C. Dong, J. Guo, W. P. Beyermann and Y. Yin, Chem.–Eur. J., 2007, 13, 7153 CrossRef CAS PubMed.
- Y. Sahoo, A. Goodarzi, M. T. Swihart, T. Y. Ohulchanskyy, N. Kaur, E. P. Furlani and P. N. Prasad, J. Phys. Chem. B, 2005, 109, 3879 CrossRef CAS PubMed.
Footnote |
† Electronic supplementary information (ESI) available: TEM images of MNFs at different reaction times, absorption and fluorescence spectra of Rh123/MNFs mixture. See DOI: 10.1039/c6ra06532e |
|
This journal is © The Royal Society of Chemistry 2016 |
Click here to see how this site uses Cookies. View our privacy policy here.