DOI:
10.1039/C6RA04445J
(Communication)
RSC Adv., 2016,
6, 38447-38453
Preparation and characterization of room temperature vulcanized silicone rubber using α-amine ketoximesilanes as auto-catalyzed cross-linkers†
Received
19th February 2016
, Accepted 30th March 2016
First published on 6th April 2016
Abstract
A series of α-amine ketoximesilanes were prepared and used as auto-catalyzed cross-linkers in one component room temperature vulcanized (RTV) silicone rubber. The synthesized α-amine ketoximesilanes were characterized by Fourier transform infrared spectroscopy (FT-IR) and nuclear magnetic resonance (NMR). Better performances of RTV silicone rubber including tack free time, viscosity, mechanical performances and thermal stability using α-amine ketoximesilanes as cross-linkers were successfully obtained, in comparison to those performances of RTV silicone rubber using commercial methyltris(methylethylketoxime)silane (MOS) as a cross-linker and dibutyltin dilaurate as a catalyst. Further, we applied density function theory (DFT) method to confirm α-amine ketoximesilanes plays a autocatalytic effect in the hydrolytic reaction of α-amine ketoximesilanes with H2O. The experimental results showed that using α-amine ketoximesilanes as cross-linkers is a practical way to obtain RTV silicone rubber with excellent properties, as well as avoiding using high chemical toxicology of organic metal catalyst in curing system.
Introduction
Silicone rubber has attracted considerable research interest because of the high thermal stability of poly(dimethylsiloxane)s (PDMS), their resistance to oxidative degradation, good low-temperature performance as well as good non-stick properties, low toxicity and low chemical reactivity.1–3 Thus, the commercial silicon rubber has been widely used in many fields, such as aviation, electrical industry, medical treatment, and automobile manufacturing.4,5 It is also worth noticed that polysiloxane is derived from non-petrochemical route and could be widely applied to promote the low carbon economy.6,7 Higher performance silicone rubber requires vulcanization process because this process could build highly three dimensional networks in the materials, making them more tougher than linear PDMS.2–6,8–16
Vulcanization of silicone rubber can be carried out at both elevated and ambient temperatures, and the silicone rubber obtained is called high temperature vulcanization (HTV) silicone rubber and room temperature vulcanization (RTV) silicone rubber, respectively.6,8,16 Although RTV silicon rubber have poorer mechanical properties compared to HTV silicones, they are easier to make and hence widely used. Popularly, RTV silicon rubber is mainly composed of PDMS, cross-linker containing a hydrolysis group, catalysts and reinforcing filler.8 The main factor that affects the mechanic performance is cross-linker. The crosslinking mechanism is commonly thought that hydrolyze groups on the silicon of cross-linker react readily with atmosphere moisture forming silanol groups, and the silanol groups further condense with the silanol groups of PDMS forming three-dimensional network structure.6,14,16 Metal catalyst is necessary for RTV silicon rubber because of the slow crosslinking rate.14 Two kinds of metal catalysts have been given priority for consideration.15–17 One is Karstedt's catalyst with platinum source, which is the most versatile and established catalyst for industrial hydrosilylation for providing access to organofunctional silanes and silicones and remains the benchmark system for new hydrosilylation catalysts.15 Nakatani et al.16 reported that platinum catalyst can accelerate the hydrosilylation of carbonyl groups and Si–H bonds. The other is tin or titanium catalyst, which successfully applied in the condensation reaction of alkoxysilane.17 However, the use of tin catalysts are currently under scrutiny by regulatory agencies in many parts of the world.18 In addition, the presences of catalyst in the PDMS networks will limit the application of RTV silicon in medicine and biology because of their high chemical toxicology.4,19,20 Therefore, it is necessary to develop a new general, simple, mild and catalyst-free curing system to prepare RTV rubber.21–23 Grande et al.21 and Thompson et al.22 have prepared RTV silicon elastomer using the dehydrocarbonative coupling of alkoxysilanes with the catalyst of B(C6F5)3. Further, Chojnowski et al.23 reported the synthesis of highly branched alkoxysiloxane-dimethylsiloxane copolymer using the same catalyst. This reaction consumes lower catalyst concentrations than Sn-based systems and the simplicity with which the 3D structures can be controlled by appropriate use of simple starting materials. It seemed likely that this reaction could similarly offer benefits to the preparation of silicone polymers with the metal-free catalyst system.
In this work, a series of α-amine ketoximesilanes were synthesized. Without the use of metal catalysts, a new type of RTV silicon rubber by the use of polysiloxanes as gums and α-amine ketoximesilanes as cross-linkers was obtained for the first time. It is found that the silicone rubber in new curing system has excellent properties compared with that in the commercial methyltris(methylethylketoxime)silane (MOS) rubber. In addition, we used density functional theory (DFT) methods to confirm the mechanism of this catalyst-free curing system.
Results and discussion
Synthesis and characterization
The synthesis route for the preparation of α-(N,N-diethyl)aminomethyltri(methylethylketoxime)silane (DEMOS) was shown in Scheme 1. The organic compound DEMOS was characterized by FT-IR, 1H NMR, 13C NMR (Fig. 1 and S2†). The strong broad peaks at 1101.23–1080.10 cm−1 indicated Si–O group stretching in Si–ONC groups. The peak at 1161.08 cm−1 was caused by the stretching vibration of C–N bands. They were O–N and C
N groups stretching in ketoxime that induced the moderate peaks at 1460.68–1376.51 cm−1. The peak at about 2800 cm−1 was due to C–H stretching of alkanes. The broad peak at 3247.17 cm−1 corresponded to N–OH bands from the hydrolyzation of ketoxime silane. 1H NMR and 13C NMR spectrum, characteristic signals of DEMOS were found as following: 1H NMR (CDCl3, ppm) δH: 2.78–2.74 (q, 4H), 2.50–2.41 (t, 6H), 2.34–2.24 (q, 6H), 1.89–1.87 (s, 9H), 1.06 (s, 2H), 1.09 (t, 9H); 13CNMR (CDCl3, ppm) δC: 166.71, 43.63, 29.00, 19.14, 15.24, 13.94, 10.84, 1.76.
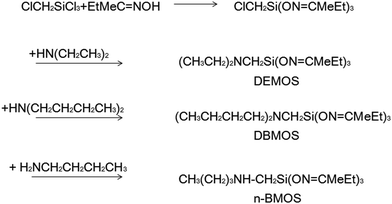 |
| Scheme 1 Synthetic routes of DEMOS, DBMOS and n-BMOS. | |
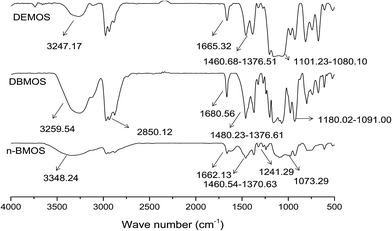 |
| Fig. 1 FT-IR spectrums of DEMOS, DBMOS and n-BMOS. | |
The α-(N,N-di-n-butyl)aminomethyltri(methylethylketoxime)silane (DBMOS) was synthesized from the chloromethyltris(methylethylketoxime)silane by using diethylamine and acetonitrile at room temperature (Scheme 1). In comparison to the FT-IR spectrum of DEMOS, the FT-IR spectrum of DBMOS displays the stronger absorption hydroxy peak stretching at 3259.54 cm−1, as shown in Fig. 1. The 1HNMR and 13CNMR spectra of DBMOS are shown in Fig. S2.† A new signal at δ = 2.2 ppm assigned to the N(CH3CH2)2, in consistent with the FT-TR results, suggesting the amine-functionalization process successfully happened. The resonances arising from DBMOS were described as following: 1H NMR (CDCl3, ppm) δH: 2.67 (t, 4H), 2.48–2.40 (m, 4H), 2.26–2.18 (m, 4H), 1.92–1.85 (t, 6H), 2.34–2.24 (q, 6H), 1.92 (s, 9H), 1.05 (s, 2H), 1.08 (t, 9H). 13CNMR (CDCl3, ppm) δC: 162.05, 48.01, 30.48, 29.00, 22.59, 20.75, 14.11, 10.81, 0.00.
The α-(N-n-butyl)aminomethyl tri(methylethylketoxime)silane (n-BMOS) was synthesized by adduction reactions of chloromethyltris(methylethylketoxime)silane with n-butylamine (Scheme 1). As shown in Fig. 1, the strong stretching peak at 1073.29 cm−1 belongs to Si–O bands of n-BMOS. The ketoxime O–N bands and C
H bands peaked at 1460.54–1370.63 cm−1 and 1662.13 cm−1, respectively. The great interplay between N–H bands in secondary amine and OH from hydrolysed ketoxime made the obvious broad peak at 3348.24 cm−1. The 1HNMR and 13CNMR spectra of n-BMOS are shown in Fig. S3.† The 1H NMR spectra showed new signals at δ = 2.39–2.29, which could be attributed to the introduction of CH3(CH2)3NH group. Moreover, resonances from the integral of 1H NMR and 13C NMR were exhibited as following: 1H NMR (CDCl3, ppm) δH: 2.55 (t, 2H), 1.75 (s, 1H), 1.43 (m, 2H), 1.33 (m, 2H), 2.39–2.29 (q, 6H), 1.83 (s, 9H), 1.02 (t, 9H), 0.99 (t, 3H), 0.81 (s, 2H). 13C NMR (CDCl3, ppm) δC: 163.38, 39.66, 31.43, 29.20, 20.80, 19.84, 12.29, 9.37, 0.00.
Tack free time
Table 1 shows the tack free time of cross-linked PDMS by using different ketoximesilanes. It was obvious that the cross-linked PDMS by using MOS as a cross-linker could not be surface cured within 72 h in the absence of dibutyltin dilaurate (Table 1). In contrast, after adding 0.2% (m/m) dibutyltin dilaurate into the MOS, the PDMS could solidify within 180 s. Moreover, cross-linked PDMS could be more easily formed by using three kinds of α-amine ketoximesilanes (i.e., DEMOS, DBMOS and n-BMOS). The tack free time of cross-linked PDMS by using MOS as a cross-linker showed a remarkable time elevation in comparison to those by using α-amine ketoximesilanes as a cross-linker, suggesting that α-amine ketoximesilanes could be used as an auto-catalyzed cross-linkers. The tack free time of cross-linked PDMS with α-amine ketoximesilanes and dibutyltin dilaurate was in the range of 90 s to 180 s. Similarly, in the α-amine ketoximesilanes system, the tack free time of cross-linked PDMS by using dibutyltin dilaurate were considerably shorter than those of cross-linked PDMS without the use of dibutyltin dilaurate (Table 1).
Table 1 Tack free time of cross-linked PDMS by using α-amine ketoximesilanes as a cross-linkera
Products (without catalyst) |
Tack free time |
Products (with catalyst) |
Tack free time |
The mass ratios of PDMS : cross-linker : catalyst = 100 : 5 : 0.2. |
PDMS + MOS |
>72 h |
PDMS + MOS |
360 s |
PDMS + DEMOS |
265 s |
PDMS + DEMOS |
164 s |
PDMS + DBMOS |
256 s |
PDMS + DBMOS |
180 s |
PDMS + n-BMOS |
150 s |
PDMS + n-BMOS |
90 s |
The changes of tack free time with different α-amine ketoximesilanes indicate faster curing of these systems in comparison with MOS system. The acceleration of curing, assessed by the decrease of tack free time, is proportional to the contents of cross-linker.4,6 Probably, some interactions between α-amine ketoximesilanes system and PDMS matrix are responsible for the significant changes of curing rate as compared with MOS system. Therefore, it is important to evaluate the optimal parameters would influence the performance of curing system. The effects of cross-linker contents on the tack free time were studied from 1% to 6% (Table 2). The individual lowest tack free time for each system was 265 s (DEMOS), 256 s (DBMOS) and 150 s (n-BMOS) with 5% contents of cross-linker in PDMS matrix, and followed by those with 6% contents of cross-linker (DEMOS, 280 s; DBMOS, 275 s; n-BMOS, 162 s) in PDMS matrix. Longer tack free time was observed by increasing the cross-linker content in the examined cross-linked PDMS, which might be due to the restriction of the elastomeric chain mobility thus preventing acceleration.24
Table 2 Tack free time for the examined cross-linked PDMS with different mass ratios of cross-linker
DEMOS content in PDMS matrix |
Tack free time |
DBMOS content in PDMS matrix |
Tack free time |
n-BMOS content in PDMS matrix |
Tack free time |
0 |
>72 h |
0 |
>72 h |
0 |
>72 h |
1% |
352 s |
1% |
340 s |
1% |
210 s |
2% |
320 s |
2% |
300 s |
2% |
193 s |
3% |
308 s |
3% |
297 s |
3% |
184 s |
4% |
290 s |
4% |
280 s |
4% |
172 s |
5% |
265 s |
5% |
256 s |
5% |
150 s |
6% |
280 s |
6% |
275 s |
6% |
162 s |
The differences in tack free time of cross-linked PDMS by using MOS versus α-amine ketoximesilanes appear to be influenced by their cross-linking mechanism, which is discussed by DFT calculation.
Chemical mechanism of crosslink reaction
The mainly factor affected the performance of room temperature vulcanized silicone rubber is the hydrolytic reaction rate of cross-linking agent. General structure for silane cross-linking agent is Y(CH2)nSiX3, where X is the hydrolysable group, Y is the amino and thiol group, etc. Y is attached to the γ-substituted silane group when n = 3, the β-substituted silane group when n = 2, and the α-substituted silane group, respectively. In order to further investigate the autocatalytic mechanism of α-amine ketoximesilanes in the preparation of cross-linked PDMS, we selected the reactions of α, β and γ-amine ketoximesilanes with H2O as simple proxies in the DFT calculation.
The calculation results at the B3LYP/6-311G (d, p) levels showed that there was only one pathways for the reaction of α-amine ketoximesilanes with H2O, as seen in Fig. 2. In the reaction of α-amine ketoximesilanes with H2O, the hydrogen on α-carbon atom was replaced by electronegative ethylenediamine when the reaction occurs, resulting in the p electrons on N atom could enter the 3d orbit of Si to form conjugated p–π orbit, which made the negative charge on Si increases, breaking the Si–ON bond successively (Fig. S4†). In contrast, in the reactions of β,γ-amine ketoximesilanes with H2O, the p electrons on N atom could not conjugated with Si because the amino group is far from the Si group, resulting in the hydrolysis reaction difficult to occur (Fig. S5 and S6†). These DFT calculation support our experimental results that the formation of cross-linked PDMS could occur much faster and easily when α-amine ketoximesilanes are present in this system.
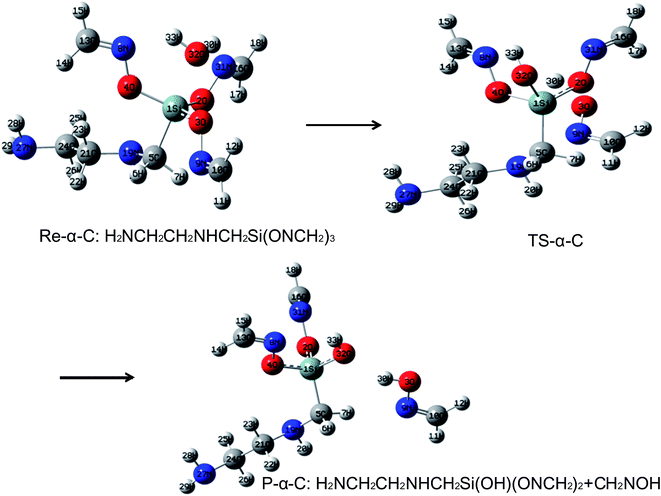 |
| Fig. 2 Stationary points for the reaction of α-ethylenediaimetriketoximesilane and H2O calculated at B3LYP/6-311G**. | |
Viscosity
Viscosity variation speed can directly reflect the internal cross-linking degree of the mixing glue, indicating the activity of different cross-linking agents. To further investigate the internal cross-linking degree differences in cross-linked PDMS with MOS as a cross-linker versus those with α-amine ketoximesilanes as cross-linkers, viscosity variations of cross-linked PDMS with different ketoximesilanes (i.e., DEMOS, DBMOS, n-BMOS) were tested during the cross-linking process. The viscosity was studied in the range of 0–50 min at the room temperature. An linear equation was fitted to the viscosity–time data with very good accuracy for all the examined systems: y = y0 + kx. Fig. 3 shows plots of the experimental data points together with the calculated data from the above equation (dashed black lines). Because R2 values for those data are in the range of 0.966 to 0.978, a very close fit can be observed. From the above fitting slope, which is related to the viscosity increase rate,24 the viscosity increase rates for α-amine ketoximesilanes system were in the range of 33.89–35.49, considerably higher than that of cross-linked PDMS with MOS (31.74). Our results suggest that α-amine ketoximesilanes could be provided satisfactory cross-linking and curing performance for cross-linked PDMS even without metal catalyst.
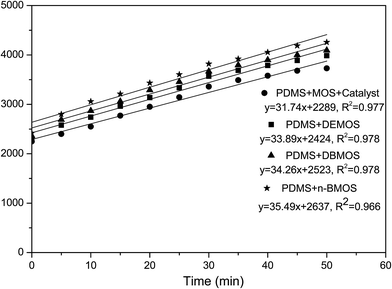 |
| Fig. 3 Viscosity (cp) versus time (min) measurements during vulcanization with different α-amine ketoximesilanes. The mass ratios of PDMS : cross-linker : catalyst = 100 : 5 : 0.2. | |
Mechanical performance and thermal stability
The chain flexibility of polymers is a result of the molecular motions available at a given temperature and is affected by the stiffness matrix in the chain.25 The tensile strength and elongation at break results of PDMS with α-amine ketoximesilanes system in comparison to MOS system are presented in Table S1.† Because of the metal catalyst confining the motions of the main chain, the tensile strengths of metal catalyzed-PDMS (1.28 Mpa) were considerably higher than metal-free system (DEMOS, 1.26 Mpa; DBMOS, 0.56 Mpa; n-BMOS, 0.85 Mpa), while the elongations at break has increased obviously from 0.76% to 3.87%, which could be deduced that the better mechanic performances of RTV rubber were obtained due to the introduction of α-amine ketoximesilanes system into the curing system.
To investigate the thermal stability of the novel RTV silicon rubber, the thermal properties with optimal conditions were evaluated by TGA and DTG. As shown in Fig. 4, the decomposition temperatures of 10% weight loss (T10) for cross-linked PDMS with α-amine ketoximesilanes were ∼530 °C, significantly higher than that of cross-linked PDMS with MOS (440 °C). In addition, the maximum weight loss rate for the cross-linked PDMS with α-amine ketoximesilanes peaked at a temperature of 600 °C, contributing about 1.3 times higher than that of cross-linked PDMS with MOS (450 °C). These results demonstrated that α-amine ketoximesilanes had much more advantage in promoting thermal stability of PDMS.
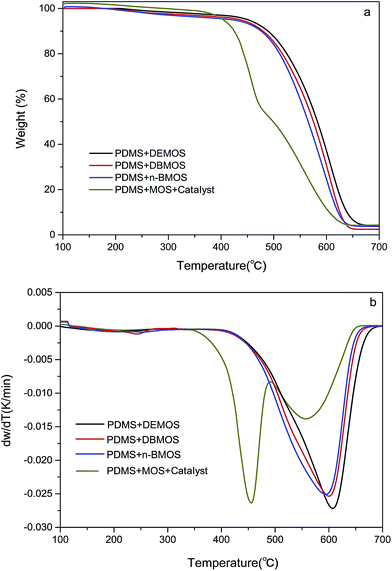 |
| Fig. 4 TGA (a) and DTG (b) curves of cross-linked PDMS with different α-amine ketoximesilanes. | |
To clarify the potential effects of catalyst on the thermal stability of cross-linked PDMS in the presence of α-amine ketoximesilanes, the thermal decomposition characteristics of the materials were determined and shown in Fig. S7.† We did not find significant differences in thermal decomposition temperature of α-amine ketoximesilanes with catalyst versus that without catalyst, which indicates that α-amine ketoximesilanes could cure PDMS successfully without the use of catalyst.
Conclusions
A series of α-amine ketoximesilanes were prepared and used as auto-catalyzed cross-linkers in one component room temperature vulcanized (RTV) silicone rubber. We used density function theory (DFT) method to confirm α-amine ketoximesilanes plays a autocatalytic effect in the hydrolytic reaction of α-amine ketoximesilanes with H2O. In the reaction of α-amine ketoximesilanes with H2O, the hydrogen on α-carbon atom was replaced by electronegative ethylenediamine when the reaction occurs, resulting in the p electrons on N atom could enter the 3d orbit of Si to form conjugated p–π orbit, which made the negative charge on Si increases, breaking the Si–ON bond successively. Better performances of RTV silicone rubber including tack free time, viscosity, mechanical performances and thermal stability using α-amine ketoximesilanes as cross-linkers were successfully obtained, in comparison to the those performances of RTV silicone rubber using commercial MOS as a cross-linker and dibutyltin dilaurate as a catalyst. The tack free time of cross-linked PDMS with α-amine ketoximesilanes were significant shorter than those of MOS system. Meanwhile, the thermal stability of α-amine ketoximesilanes system enhanced greatly than those of MOS system. The experimental results demonstrated that α-amine ketoximesilanes could provide more advantage in preparation of cross-linked PDMS with excellent properties.
Experimental
Materials
Chloromethyltrichlorosilane (industrial grade, Huai'an Debang Chemical Co. Ltd., China) were received without further purification. The following reagents were of analytical grade and used as supplied: methylethylketoxime (MOS, Aladdin Reagent Co., China); n-hexane (Tianjin Fuyu Fine Chemical Co. Ltd., China); triethylamine (Tianjin Fuyu Fine Chemical Co. Ltd., China); diacetylmonoxime (Tianjin Fuyu Fine Chemical Co. Ltd., China); dichloromethane (Tianjin Fuyu Fine Chemical Co. Ltd., China); tetrahydrofuran (Tianjin Fuyu Fine Chemical Co. Ltd., China); diethylamine (Tianjin Kemiou Chemical Reagent Co. Ltd., China); n-butylamine (Chengdu Kelong Chemical Co. Ltd., China); di-n-butylamine (Chengdu Kelong Chemical Co. Ltd., China); acetonitrile (Tianjin Guangcheng Chemical Reagent Co. Ltd., China); poly(dimethylsiloxane) (PDMS, Mw = 23
700, Jinan Hailan Chemical Co. Ltd., China); dibutyltin dilaurate (DBTDL, Aladdin Reagent Co., China); methyltris(methylethylketoxime)silane (Chengdu Xiya Reagent Co. Ltd., China).
Instruments
The Fourier transform infrared spectroscopy (FT-IR) of KBr pellets of the materials were obtained with a Bruker Tensor-27 spectrometer with the range of 400 to 4000 cm−1 at a resolution of 4 cm−1. 1H NMR and 13CNMR were conducted on Bruker AV300 MHz and Bruker AV400 MHz Ultra Shield TM magnet spectrometer using CDCl3 as a solvent, respectively. Tetramethylsilane were selected as a internal standard (δ = 0.00 ppm). The tack free time was measured according to GB/T 13447.5-2002 method at the temperature of 25 °C and humidity of 50%. The viscosity was measured by Brookfield DV-E Viscometer at a rotation speed of 12 rpm and ambient temperature. Weight-loss measurement was conducted in a Mettler Toledo SDTA-854 thermo gravimetric analyzer (TGA) under a nitrogen atmosphere from 100 °C to 700 °C at a heating rate of 20 °C min−1. The tensile strength and elongation at break were measured by tension test at the rate of 20 mm min−1 and at the temperature of 298 K. The mechanical properties of the samples were measured according to ASTM D638 with an XLD-A universal testing machine (Second Experimental Machine Factory).
Synthesis of α-amine ketoximesilanes
Synthesis of chloromethyltris(methylethylketoxime)silane. Diacetylmonoxime (105 g) and n-hexane (120 mL) were added in a four-port flask under nitrogen atmosphere. The chloromethyltrichlorosilane (37.06 g) was added drop wise to the mixture, which was mechanically stirred for 3 hours at room temperature under nitrogen atmosphere. After then, the mixture was neutralized with triethylamine and separated through a glass filter. The un-reacted volatile oligomers (n-hexane and diacetylmonoxime) were removed by distillation under reduced pressure (<10 mmHg) at 70 °C for no less than 3 hours. The chloromethyltris(methylethylketoxime)silane (Scheme 1, 95% yield) was thus obtained.
Synthesis of DEMOS. A mixture of chloromethyltris(methylethylketoxime)silane samples (67.12 g) and acetonitrile (60.0 mL) was first added in a four-port flask with a condensing tube, a stirring system, a nitrogen inlet and a bottom valve. After 5 minutes of nitrogen purge, diethylamine (36.57 g) was added into the mixture, and followed by stirring the system for 4 hours at room temperature. After the reaction, the resulting product, DEMOS (Scheme 1, 82% yield), purified by distillation under reduced pressure (<8 mmHg) to remove the remaining acetonitrile and diethylamine.
Synthesis of DBMOS. A mixture of chloromethyltris(methylethylketoxime)silane (69.45 g) and acetonitrile (105 mL) was added in a thermostated reactor fitted with a condensing tube, a stirring and a bottom valve. The di-n-butylamine (38.81 g) was then added when temperature reached 50 °C to initiate the reaction. The reaction was stopped after 5 hours of reaction at 50 °C. The crude products were separated through a glass filter and purified by distillation under reduced pressure (<6 mmHg) to remove the remaining acetonitrile and di-n-butylamine to obtain a purified product of DBMOS (Scheme 1, 60% yield).
Synthesis of n-BMOS. A mixture of chloromethyltris(methylethylketoxime)silane (63.47 g), acetonitrile (100 mL) and n-butylamine (40.88 g) was added in a four-port flask with a condensing tube, a stirring system, a nitrogen inlet and a bottom valve. The reaction was kept at 35 °C for 6 hours and purified by distillation under reduced pressure (<10 mmHg) to remove the remaining acetonitrile and n-butylamine to obtain a purified product of n-BMOS (Scheme 1, 67% yield).
Preparation of room temperature vulcanizing (RTV) silicones
Different types of cross-linkers (i.e., MOS, DEMOS, DBMOS, n-BMOS, 1–6% by weight) were added into the PDMS (Mw = 23
700) separately on a double roller mixer. The reaction was maintained at the temperature of 27 °C and humidity of 33%. After mixing for certain time, and then different types of RTV silicone were prepared. In order to compare the different types of cross-linkers in the preparation of silicon, a series of RTV silicone in the presence of dibutyltin dilaurate (0.2% by weight) were also prepared with the same procedures.
Computational modeling
Density functional theory (DFT) is a quantum mechanical modeling approach that expresses the total energy of a system as a function of electron density. From utilization of Becke's 1988 gradient-corrected exchange functional, DFT calculations can give transition state geometries and barrier heights of reactions to determine whether the reaction proceeds. In order to examine the reaction mechanisms of α-amine ketoximosilanes as auto-catalyzed cross-linker in the preparation of vulcanized silicone rubber, geometrical parameters of the reactants, transition states, and products were fully optimized at the B3LYP level with a standard basis set at 6-311G (d, p). The position of stationary points and the zero-point energy (ZPE) were determined by the vibrational frequency calculation at the same level. For each transition state, the intrinsic reaction coordinate (IRC) calculation was performed using the same electronic structure theory to confirm that it was connected to the right minima along the reaction pathway. The changes in thermodynamic functions, including entropy, enthalpy, free energies for reactants, transition states, and products, and the equilibrium constants of the reaction in the Eyring transition state theory were calculated at the B3LYP/6-311G (d, p) level. All the calculations were performed with the Gaussian 03 software package (Gaussian, Inc., Wallingford, CT, USA).26
Acknowledgements
This research was supported by Special Plan of Independent Innovation in Shandong Province (No. 2013CXC10205).
References
- B. Kumar and Y. S. Negi, Polymer, 2015, 80, 159–179 CrossRef CAS.
- H. Liu, Q. Zhu, L. Feng, B. Yao and S. Feng, J. Mol. Struct., 2013, 1032, 29–34 CrossRef CAS.
- L. Wang, D. Wang, H. Wang and S. Feng, J. Organomet. Chem., 2014, 767, 40–45 CrossRef CAS.
- L. Xue, D. Wang, Z. Yang, Y. Liang, J. Zhang and S. Feng, Eur. Polym. J., 2013, 49, 1050–1056 CrossRef CAS.
- L. Xue, Z. Yang, D. Wang, Y. Wang, J. Zhang and S. Feng, J. Organomet. Chem., 2013, 735, 58–64 CrossRef.
- F. Dong, S. Diao, D. Ma, S. Zhang and S. Feng, React. Funct. Polym., 2015, 96, 14–20 CrossRef CAS.
- Q. Liu, Z. Ji and Y. Bei, J. Colloid Interface Sci., 2013, 394, 646–651 CrossRef CAS PubMed.
- S. Diao, F. Dong, J. Meng, P. Ma, Y. Zhao and S. Feng, Mater. Chem. Phys., 2015, 153, 161–167 CrossRef CAS.
- W. Feng, S. Lv, J. Cui, X. Han, J. Du, J. Sun, K. Wang, Z. Wang, X. Lu, J. Guo, K. Oda, N. Amizuka, X. Xu and M. Li, Mater. Sci. Eng., C., 2015, 54, 133–141 CrossRef CAS PubMed.
- L. Li, R. Liang, Y. Li, H. Liu and S. Feng, J. Colloid Interface Sci., 2013, 406, 30–36 CrossRef CAS PubMed.
- L. Li, L. Xue, S. Feng and H. Liu, Inorg. Chim. Acta, 2013, 407, 269–273 CrossRef CAS.
- X. Li, P. Han, G. Song, Z. Peng, Y. Yu, S. Feng, C. Zhou and M. Li, Mater. Lett., 2015, 141, 157–160 CrossRef CAS.
- X. Li, P. Li, G. Song, Z. Peng, S. Feng and C. Zhou, Mater. Lett., 2014, 122, 58–61 CrossRef CAS.
- J. Tan, D. Ma, S. Feng and C. Zhang, Colloids Surf., A, 2013, 417, 146–153 CrossRef CAS.
- Q. H. Tan, G. H. Wang, L. Nie, A. Dinse, C. Buda, J. Shabaker and D. E. Resasco, ACS Catal., 2015, 5, 6271–6283 CrossRef CAS.
- N. Nakatani, J. Hasegawa, Y. Sunada and H. Nagashima, Dalton Trans., 2015, 44, 19344–19356 RSC.
- T. Zheng, Q. Zhou, Q. Li, H. Y. Li, L. Y. Zhang and Y. L. Hu, RSC Adv., 2015, 5, 9328–9336 RSC.
- A. Amgoune, M. Krumova and S. Mecking, Macromolecules, 2008, 41, 8388–8396 CrossRef CAS.
- D. Wang, L. Wang, L. Xue, D. Zhou, S. Feng and X. Zhao, J. Organomet. Chem., 2013, 735, 58–64 CrossRef CAS.
- L. Xue, Y. Zhang, Y. Zuo, S. Diao, J. Zhang and S. Feng, Mater. Lett., 2013, 106, 425–427 CrossRef CAS.
- J. B. Grande, A. S. Fawcett, A. J. McLaughlin, F. Gonzaga, T. P. Bender and M. A. Brook, Polymer, 2012, 53, 3135–3142 CrossRef CAS.
- D. B. Thompson and M. A. Brook, J. Am. Chem. Soc., 2008, 130, 32–33 CrossRef CAS PubMed.
- J. Chojnowski, J. Kurijata, W. Fortuniak, S. Rubinsztajn and B. Trzebicja, Macromolecules, 2012, 45, 2657–2661 CrossRef.
- S. P. Vasilakos, M. I. Triantou and P. A. Tarantili, Polym. Eng. Sci., 2015, 55, 957–965 CAS.
- Q. Liu, Y. Zou, Y. Bei, G. Qi and Y. Meng, Mater. Lett., 2008, 62, 3294–3296 CrossRef CAS.
- Y. Yu and S. Feng, J. Phys. Chem. A, 2006, 110, 12463–12469 CrossRef CAS PubMed.
Footnote |
† Electronic supplementary information (ESI) available. See DOI: 10.1039/c6ra04445j |
|
This journal is © The Royal Society of Chemistry 2016 |
Click here to see how this site uses Cookies. View our privacy policy here.