DOI:
10.1039/C6RA03866B
(Paper)
RSC Adv., 2016,
6, 30205-30216
New indole based co-sensitizers for dye sensitized solar cells exceeding 10% efficiency†
Received
11th February 2016
, Accepted 16th March 2016
First published on 17th March 2016
Abstract
In this work, we report the molecular engineering and synthesis of three novel indole co-sensitizers DBA-3, DBA-4 and DBA-5 with D–D–A (donor–donor–acceptor) architecture. In the quest to comprehend the role of auxiliary donors on co-sensitization, we have incorporated auxiliary electron donating moieties with varying geometries and electron donating capabilities to the indole moiety in order to obtain the aforementioned co-sensitizers. Their electrochemical and photo-physical properties along with molecular geometries, obtained from Density Functional Theory (DFT) are studied to vindicate the effect of the co-sensitizer structures on the photovoltaic properties of DSSCs. Furthermore, for the first time we demonstrate the profound effect of auxiliary donor groups on the co-sensitization performance of the organic molecules. Devices co-sensitized using DBA-3, DBA-4 and DBA-5 along with ruthenium sensitizer NCSU-10, displayed significantly different photovoltaic conversion efficiencies (PCEs) when compared to that of the device sensitized using only NCSU-10. The photovoltaic and EIS studies revealed that, the co-sensitizer DBA-4 succeeded in enhancing the light harvesting capability as well as efficiently suppressing undesirable charge recombinations in the cell. Due to the aforementioned reasons, a cell co-sensitized using DBA-4 has shown promising photovoltaic results and exhibited an enhanced overall efficiency of 10.12%. Furthermore, vertical electronic excitations, calculated using TD-DFT, are in good agreement with the experimental λmax results, which clearly indicates that, the energy functional and basis set utilized in this study can be effectively employed for predicting the absorption spectra of novel photosensitizers, with high confidence prior to their synthesis. All these results provide a better understanding and deeper insight into the intricacies involved in the design of superior co-sensitizers to further improve the performance of DSSCs.
1. Introduction
Solar energy has emerged as a bright spot amidst the efforts to find a clean and abundant source of renewable energy in order to meet the requirements and developments of contemporary society. In the field of solar-energy conversion into electricity, dye-sensitized solar cells (DSSCs) have gained remarkable attention due to their low cost to efficiency ratio and relative ease of fabrication.1 Although, there are many components which constitute a typical DSSC, the improvement in conversion efficiency of the cell (η) hinges on the development of new photosensitizers. In recent years, many strategies have been employed by researchers to develop efficient sensitizers having enhanced conversion efficiency and improved photo-stability. Further, the devices sensitized with ruthenium-based dyes such as N3, N719 and Black dye have shown high efficiencies.2–4
The high efficiency displayed by ruthenium-based DSSCs can be accredited to their broad absorption spectra.5 However, the aforesaid class of sensitizers are plagued by many issues, such as high cost, metal scarcity, tedious and laborious purification steps etc.6 Further, many researchers have been successful in achieving high efficiencies using porphyrin based dyes.7 However, the major drawback of porphyrin based sensitizers is the very weak absorption characteristics in the spectral region between the Soret and Q bands.8 On the other hand, the metal-free organic sensitizers are of great interest owing to their facile synthesis, low cost and high molecular extinction coefficients.9–11 However, the performance of the DSSCs based on pure organic sensitizers is hampered due to the lack of metal–ligand charge transfer (MLCT) bands and their narrow absorption profiles. Taking all these points into consideration, co-sensitization strategy is employed, wherein a DSSC based on ruthenium sensitizer is co-sensitized using an organic dye with complementary absorption spectrum and thereby enabling the cell to harvest maximum number of photons.12,13
In recent times, a wide range of strategies have been employed to extend the π-electron delocalization and upturn the molar absorptivity coefficient of the materials. In this regard, the incorporation of triphenylamine, carbazole and thiophene moieties in some of the structures have proved to be effective strategies for extending the π-conjugation of the chromophore and enhancing the molar absorptivity of the compound.14–17 Further, the aforesaid configuration makes the molecule to occupy a larger surface area than a molecule having linear shape and hence leading to the decline of dye loading capacity.18,19 Furthermore, the auxiliary donor group improves the electron donating proficiency of the principal donor and thereby leading to a bathochromic shift in the absorption spectrum that contributes to the enhancement of photocurrent.20 The typical moieties employed as auxiliary donors are nonplanar bulky substituted triarylamines with excellent electron-donating capabilities. However, in the present study the linear planar groups such as methoxy or 4-methoxyphenyl donor group together with the electron-rich indole moiety have been used to provide a seamless electron migration from donor to acceptor. The foremost objective of the present study is to comprehend the repercussions of varying the structure and strength of the auxiliary donor group on the co-sensitization properties of small organic co-sensitizers. Further, indole moiety is incorporated in the current design predominantly due to its excellent electron donating capability and exceptional light harvesting proficiency.21–23 These newly compounds are used as co-sensitizers along with ruthenium-based sensitizer NCSU-1024 to enhance its photovoltaic efficiency by counteracting the competitive light absorption due to triiodide species in 400–500 nm range of the visible spectrum and also reducing the various undesired recombination processes happening inside the cell. Moreover, in order to avoid dye aggregation and to fine-tune the distance between the ruthenium-based sensitizer NCSU-10 molecules, we introduced hexyl chains in the two co-sensitizers. The presence of these hexyl chains avoids dye aggregation and thereby resulting in the enhancement of overall efficiency of the cell. Besides, due to their higher molar extinction coefficients, these organic dyes can minimize the amount of the dye required to yield appropriate optical density, which can provide enough space on the TiO2 for the attachment of other sensitizer molecules. Further, because of the size differences between the organic co-sensitizers and the ruthenium-based NCSU-10 dye, the metal-oxide surface can be covered more effectively and efficiently as the small size of the organic dye can get adsorbed onto the gaps between the large NCSU-10 sensitizer molecules.
Typically, in DSSCs anchoring groups are of paramount importance, as they are quintessential in embedding the sensitizer onto the semiconductor surface, and thereby determining the efficiency of electron injection from the LUMO level of the sensitizer into the conduction band of the semiconductor.25 Taking this into consideration, in the present study barbituric acid has been selected as an anchoring/acceptor group in order to study its effect on the co-sensitizing characteristics as well as on the overall efficiency of the cell. Keeping all the above mentioned points in view, in the present work, we have the designed and synthesized of three new co-sensitizers (DBA-3, DBA-4 and DBA-5) having an auxiliary donor moiety attached to an indole ring linked to barbituric acid acceptor unit, leading to the formation of the corresponding co-sensitizers and their photovoltaic performance has been investigated. Fig. 1 depicts the chemical structures of the co-sensitizers and ruthenium-based sensitizer NCSU-10.
 |
| Fig. 1 Chemical structures of co-sensitizers and NCSU-10. | |
2. Experimental
2.1 Materials and methods
The starting materials 5-bromo-1H-indole, 5-methoxy-1H-indole-3-carbaldehyde, indole-3-carbaldehyde, 1-bromohexane, sodium hydride, 4-methoxyphenylboronic acid, tetrakis(triphenylphosphine)palladium(0) [Pd(PPh3)4] and barbituric acid, were obtained from Sigma-Aldrich. All the solvents were dried prior to use by following standard protocols. All the reactions were conducted under argon atmosphere and the reaction progression was monitored by TLC technique. Chromatographic separations were performed using silica gel (230–400 mesh). 1H NMR and 13C NMR spectra were recorded using Bruker Avance 500 MHz and 400 MHz spectrometers, in CDCl3 or DMSO-d6 and tetramethylsilane as internal standard. Mass spectra were acquired from thermo scientific-EXACTIVE (ESI-MS), while the elemental analysis were attained from a Flash EA1112CHNS analyzer (Thermo Electron Corporation). UV-visible spectra of all the sensitizers were obtained at room temperature using SPECORD S 600 spectrophotometer and FTIR spectra were recorded using Nicolet Avatar 5700. Further, the fluorescence spectra were acquired on a Perkin Elmer LS55 fluorescence spectrophotometer. The cyclic voltammetry (CV) and impedance studies were conducted on Bio-Logic SP-150 electrochemical workstation. Cyclic voltammograms of the dyes were executed in freshly dried acetonitrile with 0.1 M (n-Bu)4N+(ClO4)− as a supporting electrolyte. The CV experiments were performed by the conventional three electrode configuration (glassy carbon as the working electrode, platinum as counter and Ag/AgCl as a reference electrode). The data were obtained at a scan rate of 100 mV s−1. Turbomole software package was employed to perform the DFT calculations. Further, the DSSC fabrication procedure is incorporated in the ESI.†
2.2 Synthetic methods
2.2.1 Synthesis of 5-bromo-1-hexyl-1H-indole (1). A solution of 5-bromo-1H-indole (1 g, 5.1 mmol), NaH (0.244 g, 0.01 mmol) in DMF (10 mL) was stirred at room temperature for 0.5 h under argon. Then 1-bromohexane (0.925 g, 5.6 mmol) was added into the mixture and stirred at room temperature for 12 h. After the completion of reaction, water was added and neutralization was done using 2 N HCl. The product was extracted with DCM. Further, organic phase was dried over sodium sulfate and the solvent was evaporated under reduced pressure. The residue was purified by column chromatography on silica gel using hexane as an eluent to give compound 1 as a colorless liquid. Yield: 83%. 1H NMR (500 MHz, CDCl3, ppm): δ 8.46 (d, J = 1.5 Hz, 1H), 7.69 (s, 1H), 7.42–7.40 (m, 1H), 7.24 (t, J = 9 Hz, 1H), 4.14 (t, J = 7 Hz, 2H), 1.90–1.84 (m, 2H), 1.31–1.29 (m, 6H), 0.87 (t, J = 7 Hz, 3H). Calcd for C14H18BrN: C, 60.01; H, 6.47; N, 5.00 found: C, 60.21; H, 6.41; N, 5.05. ESI-MS (+ve mode) m/z calcd for C14H18BrN: 279.06 found: 280.06 [M + H]+.
2.2.2 Synthesis of 5-bromo-1-hexyl-1H-indole-3-carbaldehyde (2). A two neck round bottom flask was charged with freshly distilled DMF (1.56 mL, 5 eq.) and POCl3 (1.96 mL, 5 eq.) was added to it drop-wise, with constant stirring at 0 °C under argon to obtain a glassy white solid and to this 5-bromo-1-hexyl-1H-indole (1, 1.2 g, 4.28 mmol) dissolved in dichloroethane (10 mL) was added while stirring. The stirring was continued at ambient temperature for 15 h. After completion of reaction, the reaction mass was poured into crushed ice and subsequently basified using 5 M NaOH solution. The precipitated solid was filtered and the crude product was purified by column chromatography on silica gel using ethyl acetate/hexane (1
:
10) as mobile phase to give compound 2. Light brown solid, yield 77%. 1H NMR (500 MHz, CDCl3, ppm): δ 9.95 (s, 1H), 8.46 (d, J = 1.5 Hz, 1H), 7.69 (s, 1H), 7.42–7.40 (m, 1H), 7.24 (t, J = 8.5 Hz, 1H), 4.14 (t, J = 7 Hz, 2H), 1.90–1.84 (m, 2H), 1.31–1.29 (m, 6H), 0.87 (t, J = 7 Hz, 3H). 13C (125 MHz, CDCl3, ppm): δ 184.19, 138.59, 135.87, 126.92, 124.80, 117.42, 116.51, 111.49, 47.50, 31.25, 29.69, 26.47, 22.44, 13.94. Anal. calcd for C15H18BrNO: C, 58.45; H, 5.89; N, 4.54 found: C, 58.23; H, 5.81; N, 4.55. ESI-MS (+ve mode) m/z calcd for C15H18BrNO: 307.06 found: 308.06 [M + H]+.
2.2.3 Synthesis of 1-hexyl-5-(4-methoxyphenyl)-1H-indole-3-carbaldehyde (3). A 15 mL THF solution of compound 2 (0.5 g, 1.6 mmol), 4-methoxyphenylboronic acid (0.371 g, 2.44 mmol), Pd(PPh3)4 (0.187 g, 0.16 mmol), and 2 M K2CO3 (5 mL) was refluxed under argon atmosphere for 15 h. After cooling to room temperature, water was added to quench the reaction and extraction was done with dichloromethane (25 mL × 3). The organic layer was dried over anhydrous sodium sulphate, and the solvent was removed under reduced pressure. The crude product was purified by column chromatography on silica with hexane/ethyl acetate mixture (15
:
1). Product 3 was obtained as off-white solid. Yield 79%. 1H NMR (500 MHz, CDCl3, ppm): δ 10.02 (s, 1H), 8.49 (d, J = 1 Hz, 1H), 7.73 (s, 1H), 7.61 (d, J = 8.5 Hz, 2H), 7.55–7.53 (m, 1H), 7.41 (d, J = 8.5 Hz, 1H), 6.99 (d, J = 8.5 Hz, 1H), 4.19 (t, J = 7.5 Hz, 2H), 3.86 (s, 1H), 1.94–1.89 (m, 2H), 1.35–1.25 (m, 6H), 0.88 (t, J = 7 Hz, 3H). Anal. calcd for C22H25NO2: C, 78.77; H, 7.51; N, 4.18 found: C, 78.61; H, 7.49; N, 4.17. ESI-MS (+ve mode) m/z calcd for C20H21NO2S: 335.19 found: 336.19 [M + H]+.
2.2.4 Synthesis of 1-hexyl-5-methoxy-1H-indole-3-carbaldehyde (5a). A mixture of 1-bromohexane (0.565 g, 3.42 mmol), 5-methoxy-1H-indole-3-carbaldehyde (0.5 g, 2.85 mmol) and potassium carbonate (0.591 g, 4.28 mmol), in DMF (15 mL) was stirred at 30 °C for 10 h. After completion of the reaction, the contents were neutralized with 2 N HCl and the product was extracted using ethylacetate (50 mL × 3). Organic phase was dried using sodium sulfate. Then, ethyl acetate was removed under reduced pressure. The crude oily product obtained was purified by column chromatography on silica gel using hexane as an eluent, to get a white solid. Yield: 83%. 1H NMR (400 MHz DMSO-d6, ppm): d 9.93 (s, 1H), 7.79 (s, 1H), 7.64 (s, 1H), 7.24 (d, J = 9 Hz, 1H), 6.95 (s, J = 8.5 Hz, 1H), 4.10 (t, J = 7 Hz, 2H), 3.89 (s, 3H), 1.85 (d, J = 6 Hz, 2H), 1.30 (s, 6H), 0.87 (t, 3H). ESI-MS (+ve mode) m/z calcd for C16H21N2O2: 259.16 found: 260.16 [M + H]+.
2.2.5 Synthesis of 1-hexyl-1H-indole-3-carbaldehyde (5b). A mixture of 1-bromohexane (0.682 g, 4.13 mmol), indole-3-carbaldehyde (0.5 g, 3.44 mol), potassium carbonate (0.571 g, 4.13 mmol), in DMF (15 mL) was stirred at room temperature for 10 h. The reaction was then neutralized with 2 N HCl and the product was extracted using ethyl acetate/water (50 mL × 3). Organic phase was dried using sodium sulfate. The solvent was removed under reduced pressure and the crude oily product obtained was further purified by column chromatography on silica gel using hexane as an eluent. The product was a light brown colored liquid. Yield: 85%. 1H NMR (400 MHz, DMSO-d6): δ (ppm) 9.92 (s, 1H), 8.32 (s, 1H), 8.13 (d, J = 7.6 Hz, 1H), 7.61 (d, J = 8.4 Hz, 1H), 7.33–7.23 (m, 2H), 4.26 (t, J = 6.8 Hz, 2H), 1.78 (t, J = 6.5 Hz, 2H), 1.24 (s, 6H), 0.83 (t, J = 6.8 Hz, 3H). Anal. calcd for C15H19NO: C, 78.56; H, 8.35; N, 6.11 found: C 78.66; H, 8.43; N, 6.19.
2.2.6 Synthesis of 5-((1-hexyl-5-(4-methoxyphenyl)-1H-indol-3-yl)methylene)pyrimidine-2,4,6(1H,3H,5H)-trione (DBA-3). A mixture of 1-hexyl-5-(4-methoxyphenyl)-1H-indole-3-carbaldehyde (3, 0.5 g, 1.49 mmol) and barbituric acid (0.210 mg, 1.63 mmol) was refluxed in absolute methanol for 5 h. The obtained precipitate was filtered and recrystallized from methanol to obtain bright yellow color solid. Yield 83%. 1H NMR (400 MHz DMSO-d6, ppm): δ 11.14 (s, 1H), 11.06 (s, 1H), 9.55 (s, 1H), 8.75 (s, 1H), 8.03 (s, 1H), 7.78–7.72 (m, 3H), 7.06 (s, 2H), 4.42 (s, 2H), 3.82 (s, 3H), 1.85 (s, 2H), 1.29 (s, 6H), 0.84 (s, 3H). 13C (100 MHz, DMSO-d6) δ (ppm): 165.02, 163.71, 150.89, 142.78, 135.85, 133.62, 130.84, 128.68, 123.26, 115.93, 114.86, 112.74, 111.28, 108.96, 55.65, 47.57, 31.15, 29.70, 26.18, 22.42, 14.32. Anal. calcd for C26H27N3O4: C, 70.09; H, 6.11; N, 9.43 found: C, 70.09; H, 6.11; N, 9.41 ESI-MS (+ve mode) m/z calcd for C26H27N3O4: 445.20 found: 446.20 [M + H]+.
2.2.7 Synthesis of 5-((1-hexyl-5-methoxy-1H-indol-3-yl)methylene)pyrimidine-2,4,6(1H,3H,5H)-trione (DBA-4). The compound DBA-4 was synthesized by refluxing 5a (0.5 g, 1.92 mmol) with barbituric acid (0.271 mg, 2.12 mmol) in absolute methanol for 6 h. The obtained product was filtered and recrystallized from methanol to obtain yellow solid. Yield: 85%. 1H NMR (400 MHz DMSO-d6, ppm): δ 11.11 (s, 1H), 11.01 (s, 1H), 9.48 (s, 1H), 8.62 (s, 1H), 7.61 (d, J = 6.5 Hz, 1H), 7.34 (s, 1H), 6.98 (d, J = 7 Hz, 1H), 4.34 (s, 2H), 3.88 (s, 3H), 1.81 (s, 2H), 1.27 (s, 6H), 0.82 (d, J = 5 Hz, 3H). 13C (100 MHz, DMSO-d6) δ (ppm): 168.24, 165.10, 163.72, 156.95, 152.12, 150.90, 143.58, 142.46, 131.72, 131.42, 113.78, 113.30, 111.10, 108.07, 100.72, 56.05, 47.62, 31.11, 29.60, 26.14, 22.40, 14.29. Anal. calcd for C20H23N3O4: C, 65.03; H, 6.28; N, 11.37 found: C, 65.17; H, 6.31; N, 11.43 ESI-MS (+ve mode) m/z calcd for C20H23N3O4: 369.17 found: 370.17 [M + H]+.
2.2.8 Synthesis of 5-((1-hexyl-1H-indol-3-yl)methylene)pyrimidine-2,4,6(1H,3H,5H)-trione (DBA-5). The compound DBA-5 was synthesized by refluxing 5b (0.5 g, 2.18 mmol) with barbituric acid (0.307 mg, 2.40 mmol) in absolute methanol for 5 h. The obtained precipitate was filtered and recrystallized from methanol to obtain yellow solid. Yield: 87%. 1H NMR (400 MHz DMSO-d6, ppm): δ 11.05 (s, 2H), 9.53 (s, 1H), 8.67 (s, 1H), 7.91 (s, 1H), 7.72 (s, 1H), 7.38 (s, 1H), 4.39 (s, 2H), 1.83 (s, 2H), 1.28 (s, 6H), 0.83 (d, J = 5 Hz, 3H). 13C (100 MHz, DMSO-d6) δ (ppm): 168.23, 164.99, 163.69, 152.12, 150.90, 143.40, 142.33, 136.97, 130.24, 124.27, 123.53, 118.41, 112.32, 110.98, 108.96, 47.45, 31.13, 29.64, 26.17, 22.41, 14.31. Anal. calcd for C19H21N3O3: C, 67.24; H, 6.24; N, 12.38 found: C, 67.44; H, 6.29; N, 12.31 ESI-MS (+ve mode) m/z calcd for C19H21N3O3: 339.16 found: 340.16 [M + H]+.
3. Results and discussions
3.1 Synthesis and structure characterization
The synthetic pathways of the three new co-sensitizers DBA-3, DBA-4 and DBA-5 are depicted in Scheme 1 and 2. N-Alkylation of 5-bromo-1H-indole was done using 1-bromohexane to yield compound 1. Compound 1 was then subjected to Vilsmeier–Haack formylation to give compound 2. The product was coupled with 4-methoxyphenylboronic acid via Suzuki coupling to yield compound 3. Further, Knoevenagel condensation of this aldehyde with barbituric acid in methanol was carried out to obtain DBA-3. Compounds 5a and 5b are obtained by N-alkylation of the corresponding indole derivatives 4a and 4b, respectively. Finally, Knoevenagel condensation of the corresponding aldehydes with barbituric acid in methanol was carried out to obtain DBA-4 and DBA-5.
 |
| Scheme 1 Synthetic route of the sensitizers (i) 1-bromohexane, NaH, DMF, RT (ii) POCl3, DMF, RT (iii) Pd(PPh3)4, K2CO3, THF, ethanol, water (iv) barbituric acid, CH3OH, 80 °C. | |
 |
| Scheme 2 Synthetic route of the co-sensitizers (i) 1-bromohexane, K2CO3, DMF, RT (ii) barbituric acid, CH3OH, 80 °C. | |
3.2 Photophysical properties
The absorption and emission spectra of the co-sensitizers were recorded in CHCl3 and are depicted in Fig. 2(a) and (b), respectively; whereas the corresponding data are depicted in Table 1. From the absorption spectrum, it is evident that the dyes exhibit two distinct bands. The band, in the lower wavelength region is ascribed to the π–π* electronic excitations localized within the auxiliary donor and indole π-conjugated segment, while the band centered around 430 nm can be attributed to the charge transfer (CT) from indole donor to the barbituric acid acceptor moiety.26 Further, the fluorescence emission spectra of the co-sensitizers were recorded upon their excitation wavelengths and the relating results are tabulated in Table 1, whereas the corresponding spectra are shown in Fig. 2(b). Furthermore, the large values of Stokes shift obtained in these co-sensitizers can be ascribed to the efficient charge transfer from the indole donor moiety to the two barbituric acid acceptor groups. Fig. 2(c) depicts the UV-visible spectrum of NCSU-10. It is important to note that, the molar extinction coefficients for the charge transfer transition in these dyes are considerably higher than the ruthenium-based sensitizers.
 |
| Fig. 2 (a) UV-visible spectra of co-sensitizers in CHCl3 (10−5 M) (b) fluorescence spectra of co-sensitizers in CHCl3 (10−5 M) (c) UV-visible spectrum of NCSU-10. | |
Table 1 Absorption and emission properties for DBA-3, DBA-4, DBA-5 and NCSU-10 sensitizers
Sensitizer |
Absorption λmax (nm) |
Emission λmax (nm) |
Stokes shift (cm−1) |
ε (M−1 cm−1) |
E0–0 |
DBA-3 |
435 |
514 |
3530 |
29 990 |
2.65 |
DBA-4 |
438 |
546 |
4520 |
42 540 |
2.69 |
DBA-5 |
434 |
486 |
2520 |
32 310 |
2.75 |
NCSU-10 |
545 |
758 |
4900 |
20 650 |
1.88 |
These high values of molar extinction coefficients exhibited by the organic dyes can minimize the amount of the dye required to yield appropriate optical density, this can provide enough space on the TiO2 for the attachment of other sensitizer molecules. Further, the nature of charge transfer in these co-sensitizers is established by investigating the absorption spectrum of the precursors (Fig. 3). The absorption spectrum of compound 2 shows λmax at 301 nm, which can be ascribed to weak charge transfer from indole donor to aldehyde acceptor group. Further, on extending the π-conjugation by incorporating 4-methoxyphenylboronic acid (3) lead to a red shift in the absorption profile, which is due to more effective delocalization of the electron density. The aforesaid bathochromic shift in the absorption maxima is quite desirable. Additionally, similar observations were made for the precursor 5a of Scheme 2.
 |
| Fig. 3 UV-visible spectra of precursors in chloroform (10−5 M). | |
3.3 Molecular modeling
To gain a deeper understanding into the electronic and optical properties of the newly synthesized co-sensitizers, DFT calculations were performed using Turbomole software package. Initially all the geometry were optimized by means of semi empirical AM1 basis with MOPAC in TmoleX. Further optimization of the aforementioned geometries were carried out using C1 point group symmetry at DFT level via the Becke's three-parameter hybrid functional and Lee–Yang–Parr's gradient-corrected correlation functional (B3LYP).27,28 Further, in all the calculations, default convergence criteria were maintained for the optimizations. Furthermore, all the calculations were performed using def-TZVPP basis set. Fig. 4 portrays the optimized geometries and electron density distributions in the HOMOs and LUMOs of the co-sensitizers. From the Fig. 4, it is apparent that in the HOMO levels of all the sensitizers, the electron density is mainly located on the corresponding donor segment, whereas in the LUMO levels, a distinct shift of the electron density towards π-bridge and acceptor group can be observed. Additionally, the close proximity of the LUMO levels to the anchoring group would facilitate better orbital overlap between the 3d orbitals of titanium and hence, excited electrons can be efficiently injected into conduction band of TiO2.
 |
| Fig. 4 Optimized structure and frontier molecular orbitals of DBA-3, DBA-4 and DBA-5. | |
Moreover, time-dependent density functional theory (TD-DFT) studies were conducted to examine the electronic excitations of co-sensitizers in presence of time-dependent perturbations. Typically, in TD-DFT, a time-dependent Schrödinger equation is solved to obtain the excited-state properties of the material. In TD-DFT an adiabatic approximation is made and following which temporal nonlocality is neglected. According to the aforementioned approximation, at any point of time, the exchange–correlation (xc) functional depends only on the instantaneous density.29
Due to the aforesaid approximation, traditional approximations (time-independent) can be applied to the xc-functional derived for ground state DFT, i.e. BP (Beck–Perdew) and hybrid functionals (B3LYP).30 Typically, the precision of the results acquired using TD-DFT profoundly depends on the functional and basis set employed for the calculations. The simulated absorption spectrum of DBA-4 obtained at the B3LYP functional and def-TZVPP basis set is portrayed in Fig. 5. Although, TD-DFT is known for its inabilities in precisely predicting energies related with long-range charge transfer states due to the self-interaction error in TD-DFT, which is owing to the electron transfer in the extended charge-transfer state.
The above-mentioned variance is not observed in the present study, as the molecules under investigation are not large enough to display a long-range intramolecular charge transfer.31 From Fig. 5 it is evident that the simulated spectrum of DBA-4 exhibits two distinct bands, corresponding to π–π* and charge transfer process. Further, the simulated spectrum is well in accordance with the experimentally obtained one. The accuracy of TD-DFT studies demonstrate the validity of the basis set and functional used for the calculation.
 |
| Fig. 5 Simulated absorption spectrum of DBA-4. | |
Further, the IR-absorption spectrum of the co-sensitizer has been simulated using Turbomole software. Fig. 6 portrays the simulated FT-IR spectrum of DBA-4. As anticipated, the theoretically obtained spectrum is well in agreement with the experimentally acquired one.
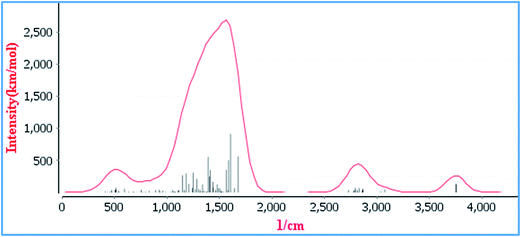 |
| Fig. 6 Theoretically obtained FT-IR spectrum of DBA-4. | |
3.4 Electrochemical characterization
Cyclic voltammetry (CV) was employed to investigate the electrochemical behavior and to evaluate the position of the lowest unoccupied molecular orbital (LUMO) and the highest occupied molecular orbital (HOMO) energy levels of the co-sensitizers. It is well established that, the efficient functioning of DSSCs necessitates that the LUMO level of the dye should be more negative than the conduction band edge of TiO2 to ensure an effective electron injection from the dye molecules to the TiO2 film and also, the HOMO level of the sensitizer is more positive than the redox potential of I3−/I− to ensure the effective regeneration of dye molecules. Fig. 7 presents the energy band diagram of the indole based co-sensitizers. From the Fig. 7, it is apparent that all the three co-sensitizers have a more negative GSOP (ground state oxidation potentials) (eV) than the Nernst potential of the I−/I3− redox couple (−5.2 eV),32 indicating that the I− species in the electrolyte can reduce and regenerate the oxidized dyes molecules and thereby, preventing the charge recombinations between oxidized dye molecules and photo-injected electrons in the conduction band of the TiO2. All the values in volts (V) against NHE were converted to electron volt (eV) by following eqn (1).20 |
ESOP = [(GSOP (V) + 4.7) − E0–0] eV
| (1) |
 |
| Fig. 7 Energy level diagram of DBA-3, DBA-4 and DBA-5 co-sensitizers. | |
The aforementioned equation was employed to calculate the ESOP (excited state oxidation potentials) levels of all the co-sensitizers by using GSOP values and zeroth–zeroth energy (E0–0) of the corresponding co-sensitizer. It is evident that, the LUMO levels of all the co-sensitizers are sufficiently more negative than the conduction band edge of TiO2 and hence, providing ample thermodynamic driving force for efficient electron injection. The negative free energy for electron injection is in the order: DBA-4 > DBA-5 > DBA-3. From the aforementioned data, it can be envisaged that, co-sensitizer DBA-4 will be more efficient in injecting electrons into the conduction band of TiO2 than the other two co-sensitizers.
Thus, although all the three co-sensitizers fulfill the necessary criteria required for a molecule to act as a sensitizer, there is a stark contrast between the thermodynamic feasibility of electron injection. This difference might prove to be crucial in determining overall efficiency of the co-sensitized devices.
3.5 Photovoltaic device characterization
The photovoltaic performance of devices sensitized using NCSU-10 alone and co-sensitized using DBA-3, DBA-4 and DBA-5 on a nanocrystalline TiO2 electrode have been investigated under standard AM 1.5 irradiation (100 mW cm−2). The photovoltaic properties of the co-sensitized devices were studied in order to comprehend the ramifications of auxiliary donor's architecture in the co-sensitizers and to establish a relationship between the structure of the co-sensitizers and their performance in the cell. The introduction of varying electron donating substituent on the principal donor group in all co-sensitizers have had profound influence on the incident photon-to-current conversion efficiency and photovoltaic properties of the co-sensitized devices. The device co-sensitized using DBA-4 with methoxy group as auxiliary donor showed superior performance than the device co-sensitized using DBA-5 and the one co-sensitized using DBA-3, which showed the lowest photovoltaic properties. Although, the ruthenium sensitizer NCSU-10 absorbs light in a wide range of wavelengths, the co-sensitizers are employed to perceive the potential of novel co-sensitizers for improving the light harvesting efficiency in the range from 400 to 500 nm and to try and suppress the dark current due to back electron transfer between injected electrons in the conduction band of TiO2 and triiodide species present in the electrolyte.
The molar extinction coefficients of the co-sensitizers are in the range from 2.9 × 104 to 4.2 × 104 M−1 cm−1, which is much higher than that of I3− (2.5 × 104 M−1 cm−1) at this wavelength. Therefore, the aforementioned co-sensitizers should be effective in reducing the competitive absorption of light by I3− and thereby, resulting in an enhancement in IPCE efficiency. Moreover, owing to their small size the organic co-sensitizers can cover the surface of the TiO2 nanoparticles more efficiently, where the large sized three-dimensional ruthenium based dye (NCSU-10) molecules cannot get adsorbed, and hence providing a better surface coverage.33,34 Further, the presence of co-sensitizers also helps in suppressing the charge recombinations caused by dye aggregation or close π–π stacking and thereby, considerably improving the Voc values.35 Current–voltage (J–V) characteristics of the cell based on NCSU-10 and the corresponding co-sensitized solar cells are presented in Fig. 8, while the corresponding photovoltaic parameters are summarized in Table 2. The photovoltaic performances (η%) of the DSSCs co-sensitized using 0.2 mM of DBA-3, 0.3 mM of DBA-3, DBA-4 and DBA-5 are 8.61, 8.60, 10.12, and 9.20%, respectively. In the quest to understand the reasons for this prevalent difference in the photovoltaic performances, IPCE studies were performed (Fig. 9). The pivotal cause for the enhanced photovoltaic performance of the cell co-sensitized by DBA-4 and DBA-5 with respect to the cell sensitized using only NCSU-10 is the enhancement in photocurrent values. It was also proposed that, acidic co-adsorbents, such as CDCA, may act as a proton buffer for the dye, thus ‘regulating’ the dye proton content and assisting dye adsorption onto the semiconductor surface.
 |
| Fig. 8 J–V characteristics of DSSCs based on NCSU-10 and co-sensitized with DBA-3, DBA-4 and DBA-5. | |
Table 2 Photovoltaic parameters of DSSCs based on NCSU-10 with/without co-sensitization
Sensitizer |
CDCA (mM) |
Jsc (mA cm−2) |
Voc (V) |
ff (%) |
η (%) |
NCSU-10 (mM) |
Co-sensitizers |
0.2 |
— |
20 |
18.23 ± 0.04 |
0.706 ± 0.003 |
68.7 ± 0.2 |
8.84 ± 0.02 |
0.2 |
DBA-3 0.2 mM |
20 |
18.47 ± 0.03 |
0.693 ± 0.002 |
67.3 ± 0.1 |
8.61 ± 0.01 |
0.2 |
DBA-3 0.3 mM |
20 |
18.97 ± 0.04 |
0.690 ± 0.003 |
65.7 ± 0.2 |
8.60 ± 0.01 |
0.2 |
DBA-4 0.2 mM |
20 |
22.69 ± 0.04 |
0.715 ± 0.003 |
62.4 ± 0.3 |
10.12 ± 0.03 |
0.2 |
DBA-6 0.2 mM |
20 |
20.49 ± 0.04 |
0.687 ± 0.004 |
65.4 ± 0.3 |
9.20 ± 0.01 |
N719 |
— |
20 |
15.9 ± 0.04 |
0.73 ± 0.003 |
66 ± 0.4 |
7.64 ± 0.03 |
LI-3 |
— |
— |
9.46 ± 0.00 |
0.71 ± 0.00 |
64 ± 0.00 |
4.31 ± 0.00 |
LI-4 |
— |
— |
10.25 ± 0.00 |
0.69 ± 0.00 |
61 ± 0.00 |
4.31 ± 0.00 |
 |
| Fig. 9 IPCE spectra of the DSSCs based on NCSU-10 and co-sensitized with DBA-3, DBA-4 and DBA-5. | |
The aforementioned enhancement in photocurrent can be attributed to the improved light harvesting efficiency of the co-sensitized solar cell. Although, the devices co-sensitized using DBA-3 also displayed improved light harvesting capabilities with a corresponding enhancement in Jsc values, but were dammed by lowered Voc values as compared to the cell sensitized by NCSU-10 sensitizer alone. The drop in Voc values suggests that the electron recombination rate has amplified as a result of co-sensitization, thereby, leading to the inferior performance of the co-sensitized devices. All these observations were further established by performing electrochemical impedance spectroscopy analysis. Further, it is noteworthy that, the first indole based sensitizers for DSSC's were designed and synthesized by Li et al. in the year 2009.36 In the aforesaid study, Li et al. attached triphenylamine and carbazole moieties to the pyrrole ring through a carbon–nitrogen single bond, in order to suppress the dye-aggregation on the surface of TiO2. However, the maximum efficiency obtained was only 4.12% (Table 2). Clearly, in the present study a significant improvement in the overall efficiency (10.12%) has been achieved as a result of co-sensitization strategy.
3.6 Electrochemical impedance spectroscopy characterization
Electrochemical impedance spectroscopy (EIS) provides rational insights into the charge dynamics of an operating solar cell and is powerful technique for evaluating the interfacial charge recombination dynamics and redox reaction process taking place at photoanode/electrolyte interface.26 Fig. 10 depicts the Nyquist plots of DSSC sensitized with only ruthenium based sensitizer NCSU-10 as well as the devices co-sensitized using various co-sensitizers.
 |
| Fig. 10 EIS Nyquist plots for DSSCs based on NCSU-10 and cosensitized with DBA-3, DBA-4 and DBA-5. | |
As evidenced from the figure, all the devices depicted two distinct semicircles, the peak in high frequency region can be attributed to resistance at the counter electrode/electrolyte interface, whereas the peak in the middle frequency range is accredited to charge recombination resistance (Rct) at TiO2/dye/electrolyte interface. From the Nyquist plots it is evident that, radius of semicircle in the middle-frequency range decreased in the order: 0.2 mM DBA-4 > NCSU-10 > 0.2 mM DBA-3 > 0.3 mM DBA-3 > 0.2 mM DBA-5. The higher electron recombination resistance observed for the device co-sensitized using DBA-4 relative to other devices indicates more effective suppression of the back reaction of the injected electrons with triiodide species in the electrolyte. The aforementioned is reflected in the improvements observed in the Jsc or Voc value, yielding substantially enhanced device efficiency for the device co-sensitized using DBA-4.
Further, the Bode plots (Fig. 11) of all the devices also exhibit two distinct peaks: one at lower frequency and another one at higher frequency. From the literature, it is well established that, the characteristic frequency at the lower frequency peak in the Bode plot is related to the charge recombination rate, and is associated with the electron lifetime according to the relation: τCB = 1/(2πf),37 where τ is the lifetime of electrons in TiO2 and f is the intermediate frequency peak from Bode plot. The electron lifetimes for the DSSCs co-sensitized using 0.2 mM DBA-3, 0.3 mM DBA-3, 0.2 mM DBA-4 and 0.2 mM DBA-5 were found to be 9.3, 8.7, 10.5 and 8.5 ms, respectively. Whereas, the electron lifetime in the device sensitized using only NCSU-10 was found to be 9.8 ms. These results suggest that the charge recombination is suppressed in the device co-sensitized using DBA-4, when compared to the other two co-sensitizers (DBA-3 and DBA-5). The calculated electron lifetime values are well in agreement with the Voc values of these devices (Table 2).
 |
| Fig. 11 Bode plots for DSSCs based on NCSU-10 and co-sensitized with DBA-3, DBA-4 and DBA-5. | |
4. Conclusions
In conclusion, three new indole based organic molecules (DBA-3, DBA-4 and DBA-5) have been successfully designed and synthesized as effective co-sensitizers for application in DSSCs. A systematic exploration between the structure and photovoltaic performance of the co-sensitizers has been carried out. Further, based on the IPCE and photovoltaic studies, it is evident that, all the three co-sensitizers were successful in enhancing light harvesting efficiency and thereby, increasing the photocurrent in the co-sensitized devices. The EIS studies revealed that, only the device co-sensitized using DBA-4 was capable in effectively suppressing the undesirable charge recombinations occurring in the cell and thereby resulting in longer electron lifetime. The aforesaid observations highlight the importance of molecular matching between the sensitizer and co-sensitizer. Consequently, the aforesaid device has achieved a remarkable PCE of 10.12%, rendering the newly synthesized co-sensitizer (DBA-4) highly attractive. In addition, the profound role of auxiliary donor groups on the co-sensitization properties has been demonstrated. Finally, we strongly believe that, the aforementioned structure–performance relationship will facilitate in further enhancing the overall efficiency of DSSCs.
Acknowledgements
The authors are thankful to NITK, Surathkal, India, for providing necessary laboratory facilities. The authors are also thankful to the department of Textile Engineering, Chemistry and Science at North Carolina State University for the financial support.
Notes and references
- M. Grätzel, Nature, 2001, 414, 338–344 CrossRef PubMed.
- M. K. Nazeeruddin, P. Péchy and M. Grätzel, Chem. Commun., 1997, 1705–1706 RSC.
- M. K. Nazeeruddin, S. M. Zakeeruddin, R. Humphry-Baker, M. Jirousek, P. Liska, N. Vlachopoulos, V. Shklover, C.-H. Fischer and M. Grätzel, Inorg. Chem., 1999, 38, 6298–6305 CrossRef CAS PubMed.
- M. K. Nazeeruddin, F. De Angelis, S. Fantacci, A. Selloni, G. Viscardi, P. Liska, S. Ito, B. Takeru and M. Grätzel, J. Am. Chem. Soc., 2005, 127, 16835–16847 CrossRef CAS PubMed.
- L. Wei, Y. Na, Y. Yang, R. Fan, P. Wang and L. Li, Phys. Chem. Chem. Phys., 2014, 17, 1273–1280 RSC.
- Y. Numata, A. Islam, H. Chen and L. Han, Energy Environ. Sci., 2012, 5, 8548–8552 CAS.
- Y. Wang, B. Chen, W. Wu, X. Li, W. Zhu, H. Tian and Y. Xie, Angew. Chem., 2014, 126, 10955–10959 CrossRef.
- S. Fan, X. Lu, H. Sun, G. Zhou, Y. J. Chang and Z.-S. Wang, Phys. Chem. Chem. Phys., 2015, 18, 932–938 RSC.
- S. Higashijima, Y. Inoue, H. Miura, Y. Kubota, K. Funabiki, T. Yoshida and M. Matsui, RSC Adv., 2012, 2, 2721–2724 RSC.
- G. D. Sharma, M. A. Reddy, K. Ganesh, S. P. Singh and M. Chandrasekharam, RSC Adv., 2013, 4, 732–742 RSC.
- A. Abbotto, V. Leandri, N. Manfredi, F. De Angelis, M. Pastore, J.-H. Yum, M. K. Nazeeruddin and M. Grätzel, Eur. J. Org. Chem., 2011, 2011, 6195–6205 CrossRef CAS.
- S. Chang, H. Wang, Y. Hua, Q. Li, X. Xiao, W.-K. Wong, W. Y. Wong, X. Zhu and T. Chen, J. Mater. Chem. A, 2013, 1, 11553–11558 CAS.
- C. Magne, M. Urien and T. Pauporté, RSC Adv., 2013, 3, 6315–6318 RSC.
- M. Liang, W. Xu, F. Cai, P. Chen, B. Peng, J. Chen and Z. Li, J. Phys. Chem. C, 2007, 111, 4465–4472 CAS.
- T. Marinado, K. Nonomura, J. Nissfolk, M. K. Karlsson, D. P. Hagberg, L. Sun, S. Mori and A. Hagfeldt, Langmuir, 2010, 26, 2592–2598 CrossRef CAS PubMed.
- J. Tang, J. Hua, W. Wu, J. Li, Z. Jin, Y. Long and H. Tian, Energy Environ. Sci., 2010, 3, 1736–1745 CAS.
- J. He, J. Hua, G. Hu, X. J. Yin, H. Gong and C. Li, Dyes Pigm., 2014, 104, 75–82 CrossRef CAS.
- W. Wu, J. Yang, J. Hua, J. Tang, L. Zhang, Y. Long and H. Tian, J. Mater. Chem., 2010, 20, 1772–1779 RSC.
- Y. Hua, S. Chang, D. Huang, X. Zhou, X. Zhu, J. Zhao, T. Chen, W.-Y. Wong and W.-K. Wong, Chem. Mater., 2013, 25, 2146–2153 CrossRef CAS.
- D. D. Babu, H. Cheema, D. Elsherbiny, A. El-Shafei and A. V. Adhikari, Electrochim. Acta, 2015, 176, 868–879 CrossRef CAS.
- G. D. Sharma, M. A. Reddy, K. Ganesh, S. P. Singh and M. Chandrasekharam, RSC Adv., 2013, 4, 732–742 RSC.
- Q. Li, L. Lu, C. Zhong, J. Shi, Q. Huang, X. Jin, T. Peng, J. Qin and Z. Li, J. Phys. Chem. B, 2009, 113, 14588–14595 CrossRef CAS PubMed.
- S. Higashijima, Y. Inoue, H. Miura, Y. Kubota, K. Funabiki, T. Yoshida and M. Matsui, RSC Adv., 2012, 2, 2721–2724 RSC.
- A. El-Shafei, M. Hussain, A. Atiq, A. Islam and L. Han, J. Mater. Chem., 2012, 22, 24048–24056 RSC.
- W. Lee, S. B. Yuk, J. Choi, H. J. Kim, H. W. Kim, S. H. Kim, B. Kim, M. J. Ko and J. P. Kim, Dyes Pigm., 2014, 102, 13–21 CrossRef CAS.
- D. D. Babu, S. R. Gachumale, S. Anandan and A. V. Adhikari, Dyes Pigm., 2015, 112, 183–191 CrossRef CAS.
- A. D. Becke, Phys. Rev. A, 1988, 38, 3098–3100 CrossRef CAS.
- C. Lee, W. Yang and R. G. Parr, Phys. Rev. B: Condens. Matter Mater. Phys., 1988, 37, 785–789 CrossRef CAS.
- M. J. G. Peach, P. Benfield, T. Helgaker and D. J. Tozer, J. Chem. Phys., 2008, 128, 044118 CrossRef PubMed.
- A. D. Becke, J. Chem. Phys., 1993, 98, 1372–1377 CrossRef CAS.
- R. Sánchez-de-Armas, M. Á. S. Miguel, J. Oviedo and J. F. Sanz, Phys. Chem. Chem. Phys., 2011, 14, 225–233 RSC.
- P. Qu and G. J. Meyer, Langmuir, 2001, 17, 6720–6728 CrossRef CAS.
- L. H. Nguyen, H. K. Mulmudi, D. Sabba, S. A. Kulkarni, S. K. Batabyal, K. Nonomura, M. Grätzel and S. G. Mhaisalkar, Phys. Chem. Chem. Phys., 2012, 14, 16182–16186 RSC.
- Y.-J. Chen, Y.-C. Chang, L.-Y. Lin, W.-C. Chang and S.-M. Chang, Electrochim. Acta, 2015, 178, 414–419 CrossRef CAS.
- L. Han, A. Islam, H. Chen, C. Malapaka, B. Chiranjeevi, S. Zhang, X. Yang and M. Yanagida, Energy Environ. Sci., 2012, 5, 6057–6060 CAS.
- Q. Li, L. Lu, C. Zhong, J. Shi, Q. Huang, X. Jin, T. Peng, J. Qin and Z. Li, J. Phys. Chem. B, 2009, 113, 14588–14595 CrossRef CAS PubMed.
- D. D. Babu, R. Su, A. El-Shafei and A. V. Adhikari, Electrochim. Acta DOI:10.1016/j.electacta.2016.03.061.
Footnote |
† Electronic supplementary information (ESI) available: DSSC fabrication procedures, optimized ground-state geometries and 1H NMR, 13C NMR, mass spectra, FTIR spectra of sensitizers. See DOI: 10.1039/c6ra03866b |
|
This journal is © The Royal Society of Chemistry 2016 |