DOI:
10.1039/C6RA02996E
(Paper)
RSC Adv., 2016,
6, 33501-33509
Bionanocomposite from self-assembled building blocks of nacre-like crystalline polymorph of chitosan with clay nanoplatelets†
Received
1st February 2016
, Accepted 21st March 2016
First published on 22nd March 2016
Abstract
Biopolymers are biocompatible and nontoxic but their other properties rank much below synthetic polymers. Their mechanical performance is improved significantly in bionanocomposites with a layered brick-and-mortar structure biomimicking the nacre of a crustaceous shell after integration with nanoparticles. Biopolymers are found in a disordered state, whereas crustaceans hold chitin in a crystalline form. Herein, it is biomimicked in a bionanocomposite of chitosan with clay nanoplatelets. Films were prepared using a new one-pot technique combining an initial formation in situ of building blocks of clay nanoplatelets and chitosan macromolecules charged progressively in their presence with evaporation-induced self-assembly, which results in highly ordered nanocrystalline narrow rectangle microparticles of thickness is ca. 15 nm that are made from interstratified layers of a new crystalline polymorph of chitosan with nanoplatelets. Its presence and structure is characterized by small/wide-angle X-ray scattering, scanning and transmission electron microscopy, microRaman spectroscopy. Crystalline polymorph can be separated easily. It is insoluble in water and organics that provide scope for its use in nanocomposites.
1 Introduction
Bionanocomposites consisting of biopolymers and nanoparticles attract currently widespread attention. Among the advantages are biocompatibility and biodegradability, abundant renewable raw sources, improved performance, durability, mechanical and other properties approaching to that of synthetic polymers manufactured mainly from fossil resources.1–8 As believed, their application will give mankind the chance to decrease the human impact on the environment. Chitosan is considered one of the most promising building components of bionanocomposites.1,3,7,9,10 It is produced from chitin (Fig. 1), of which the renewable amount is rather close to the cellulose value, ranging annually up to 1011 tons.11,12 Chitosan demonstrates good film- and fiber-forming properties, used for coatings, absorbing metal ions and organic pollutants in wastewater.11,13–19 Furthermore, it is also biologically active, possessing well-documented antiseptic, wound-healing and immune-stimulating activities.11,12,16,20–26
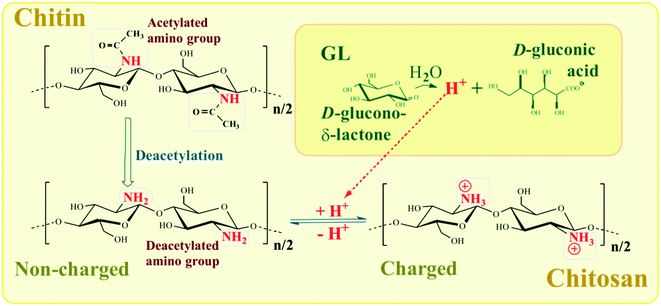 |
| Fig. 1 Structural formula of chitin and chitosan shown in charged and non-charged forms. Its charging was performed in a progressive manner by adding D-glucono-δ-lactone (see inset) to a solution that hydrolyzes slowly with the formation of D-gluconic acid after its contact with water.76 | |
The application of this cationic polysaccharide in material chemistry is frequently based on its positive charging.9,14,17,26,27 The vast majority of polyelectrolytes, organics, nano/microparticles, living cells and surfaces bring negative charges. Chitosan interacts with them electrostatically, forming polyelectrolyte complexes or attaching to the surfaces.7,19,28–31 The electrostatic association with nanoparticles bearing negative charges also forms the basis for bionanocomposite formation.3,7,19,32–34
The common preparation technique consists of mixing of solutions of chitosan and dispersion of nanosized particles. It seems a simple matter but in actual practice it is not so easy to regulate the processes and prepare bionanocomposites with homogeneously distributed constituents. Chitosan feature is its high sensitivity to the presence of anionic substances or particles in its solutions.7,27,28,35 Even trace amounts cause the precipitation of a heterogeneous mixture, in which the proper distribution of a nanoscale component in bulk bionanocomposites is physically impracticable, even though vigorous stirring or ultrasonic treatment is applied.
Mineralized tissues or biominerals of living organisms serve as examples of composite materials with a highly hierarchically organized structure from the nano- to macro-scale.36–45 A well-known example is provided by the most studied nacre of the abalone shell. Its extraordinary mechanical properties from the constituents originate from a brick-and-mortar layered composite structure formed by calcium carbonate platelets and sheets from fibrous chitin networks covered by acidic proteins.37,42,46–52 When biomimicking the nacreous construction at the nano/microscopic levels, material scientists have found remarkable mechanical performance.8,43,44,50,53–64 To the best of our knowledge, developed numerous materials with nacre-like structure constitutes of (bio)polymers in a disordered state, whereas the crustaceous shells contain highly structurally ordered crystalline chitin.14,65,66
Herein, it has been biomimicked for the first time. A layered film was made from chitosan and clay nanoplatelets, which self-assembled together in the form of a crystalline polymorph. It has come about through previous study reported by Shchipunov et al.67 The primary goal of this study is to provide evidence of a new crystalline polymorph formation.
2 Experimental
2.1 Materials
Chitosan poly-(β-(1,4)-D-glucosamine-co-N-acetyl-D-glucosamine) shown in Fig. 1, ca. 400 kDa, deacetylation degree about 85% was obtained from Fluka (Germany). Saponite SKS-20 was supplied by Clariant (Germany). D-Glucono-δ-lakton (GL) and glycerol were purchased from Merck (USA) and Mosreactiv (Russia), respectively. They were used as received.
2.2 Preparation of bionanocomposite films
The bionanocomposite films were prepared using the method suggested in our previous publication.68 In brief, chitosan as a fine powder was dispersed in a solution with a 1.5 wt% saponite and 2 wt% glycerol serving as a plasticizer. 1 wt% GL was added under continuous stirring. The mentioned concentrations were kept constant in this study. Only the polysaccharide content was varied from 0.02 to 2.4 wt%, which gave a change of its ratio to clay nanoparticles over a broad range of 0.013–1.6. More details about the dependence of bionanocomposite formation and properties on the component concentrations can be found in previous publications.67–70 The prepared solution was poured into a plastic vessel after its viscosity was increased to the point of ceasing the precipitation of chitosan microparticles. It was dried in a thermostat at 40 °C.
2.3 Separation of crystalline polymorph
A bionanocomposite film was placed in water to swell. It was useful to take samples with a composition a little further from the stoichiometric ratio of chitosan and saponite (see ESI file for details, Fig. S1 and S2†). In this case, the films absorbed a certain amount of water that facilitated their disintegration, whereas the soluble components were solubilized in water. To disintegrate a film and separate the crystalline polymorph, swollen samples were treated in an ultrasonic bath for an hour followed by centrifugation at 2.500 rpm for 30 min. The supernatant solution containing the crystalline polymorph was separated from the precipitate for further characterization.
2.4 Characterization
The film morphology was studied by a scanning electron microscopy (SEM), a model EVO 40 (Carl Zeiss, Germany). Before the observation, a small piece was cut to have a freshly prepared surface. It was coated with sprayed platinum with a small amount of gold. Transmission electron microscopy (TEM) was performed using a high resolution JEM-2010 (JEOL, Japan) or Libra 200 (Carl Zeiss, Germany). The samples for observations were prepared by dispersing a tiny film piece in ethanol using an ultrasonic dispenser. Its droplet was placed onto a copper grid coated with an amorphous polymer or carbon film and dried under a warm air stream. Small angle X-ray scattering (SAXS) was performed using the S3-MicroPIX SAXS spectrometer (Hecus X-Ray Systems GmbH, Austria). It was equipped with an X-ray generator (XenoX GeniX, France) operating at 50 kV and 1 mA with a sealed tube Cu anode (CuKα radiation, λ = 0.154 nm). A Stoe Stadi P diffractometer was used for wide angle X-ray scattering (WAXS) measurements to have powder diffraction data from examined films. The 2θ angular regions between 5° and 80° were explored with an angular resolution of 0.02°. The microRaman spectra were taken with a confocal Raman microscope (WITec alpha 500/300R+, WITec, Germany) equipped with a back-illuminated CCD camera at a wavelength of 532 nm from 100 to 3700 cm−1.
3 Results and discussion
3.1 Film preparation
The preparation of the bionanocomposite films proceeds through a sequence of two processes: the formation of building blocks of chitosan with nanoparticles in situ through its regulated charging in their solution and evaporation-induced self-assembly. The method was suggested initially in ref. 67 for the preparation of hydrogels and then adapted for films.7,68 It is based on the property of chitosan to be in a charged or non-charged state depending on the media pH (Fig. 1).11,13,14,17,71–74 In the beginning, polysaccharide was added to a solution of dispersed individual nanoparticles at pH around 6.5. Synthetic saponite, which along with montmorillonite belongs to the same clay smectite family,75 was used because of its complete delamination in water into less than 1 nm thick sheets having a lateral size of 20–40 nm. Its dispersion proceeds with ease after the contact with water and simple stirring with a magnetic stirrer. Saponite nanoparticles can be observed in Fig. 2A and B. After mixing, most amino groups of chitosan at pH 6.5 are in the uncharged state because of their pKa ≅ 6.3 (Fig. 1). Charging is regulated via the acidification of a solution that is made by a gradual pH shift by means of GL.67 This nontoxic acidifying agent used in Food Chemistry is hydrolyzing slowly after the contact with water, producing D-gluconic acid (Fig. 1, ref. 76). The macromolecules charged progressively in situ coat nanoplatelets bearing opposite charges. Their association through the electrostatic interactions was demonstrated previously and confirmed by Raman spectroscopy. Details are given in the ESI as Fig. S3.† The associates thus formed serve as building blocks of free-standing films formed through self-assembly in the course of water evaporation. Their formation and evaporation-induced self-assembly is illustrated by the schematic drawing in Fig. 3A–D.
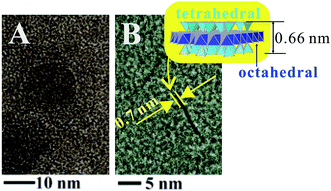 |
| Fig. 2 TEM images of saponite nanoplatelets. Two nanoplatelets are observed in horizontal (A) and upright (B) positions. Synthetic saponite is easily dispersed into individual nanoplatelets 20–40 nm in diameter (A). Their thickness is ca. 0.7 nm (B). This corresponds to an unhydrated clay nanoparticle only visible under a microscope in a vacuum. The inset shows a schematic of its crystalline structure consisting of an octahedral layer between two tetrahedral layers of 0.22 nm thick each and 0.66 nm as the total. | |
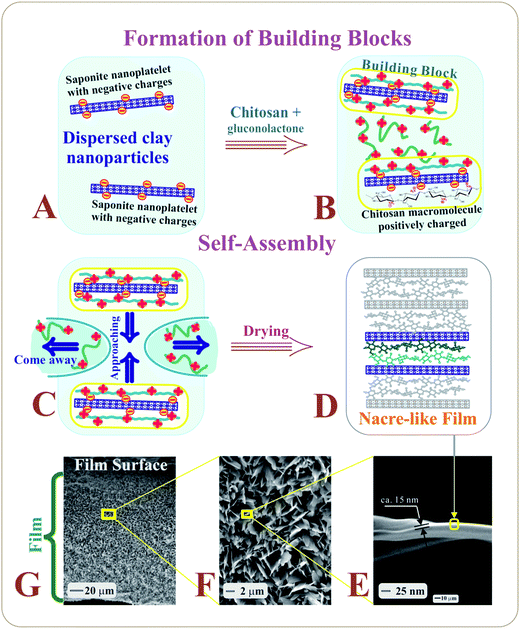 |
| Fig. 3 Schematic of the method used to prepare the films, including the formation of building blocks (A and B) and their self-assembly into a film with a nacre-like structure (C and D). Structural unit of chitosan-saponite polymorph in (D) is shown in color. The method is detailed in the text. (E) SEM image of a microparticle edge of thickness about 15 nm at large magnification. (F) House-of-cards-type stacking of microparticles in the interior of the film. (G) SEM image of a bionanocomposite film cross-section. | |
There is a vague similarity between our approach and the method used in ref. 55 and 77. The authors in the cited studies used montmorillonite to prepare bionanocomposites with chitosan. It was made in two stages: (i) preparation of building blocks by mixing solutions of chitosan and clay and then their separation. (ii) Film formation using a solution prepared initially by dispersing the building blocks. Its major disadvantage is a heterogeneous mixing of polysaccharide with nanoparticles. When both solutions are poured together, fast cooperative electrostatic interactions between oppositely charged chitosan and montmorillonite cause the instant appearance of an inhomogeneous precipitate.7,78 We could exclude the precipitation, preparing homogeneous building blocks only in the course of the progressive charging of chitosan in the solutions of nanoparticles.7,67
3.2 Structure and morphology
Morphological features of an inner volume of films are obvious from a series of images obtained by SEM at three different magnifications in Fig. 3E–G. It provides insight into its structural organization at various hierarchical levels. One may observe that the film inner volume (Fig. 3G) is filled with microparticles (Fig. 3F) of nanosized thickness (Fig. 3E). They are shaped mainly like a narrow rectangle, attaining a length of 100 μm or even longer (Fig. 3F) at the same time being as thick as about 15 nm (Fig. 3E). The difference in the aspect ratio is three orders of magnitude. Of significant importance is that the microparticles are very homogeneous over their thickness (Fig. 3E and F). The mentioned features point to the fact of their high structural ordering. Saponite nanoplatelets, of which thickness is slightly smaller than 1 nm and the diameter is between 20 and 40 nm (Fig. 2A and B), are most likely oriented in a plane of the microparticles, thereby determining the arrangement of less rigid chitosan macromolecules.
An insight into structural organization of microparticles is gained by combining SAXS, WAXS, TEM, SEM and microRaman techniques. Results are summarized in Fig. 4 and given in Fig. S3.† Normalized intensity (I) measured by the SAXS versus scattering vector (q) for five samples (curves 2–7) and a film made from saponite alone (curve 1) is shown in Fig. 4A. The bionanocomposites were prepared from solutions with the same saponite content of 1.5 wt%, while the chitosan concentration was varied over the broad range from 0.02 to 2.4 wt%. As soon as the clay nanoparticles were combined with the polysaccharide, it resulted in an appearance of a sharp Bragg reflex at q = 0.35 Å−1 and two broad peaks at smaller angles in the SAXS pattern. Before chitosan addition, there is a smooth curve 1 without certain reflections in the range of scattering vectors examined for saponite alone.
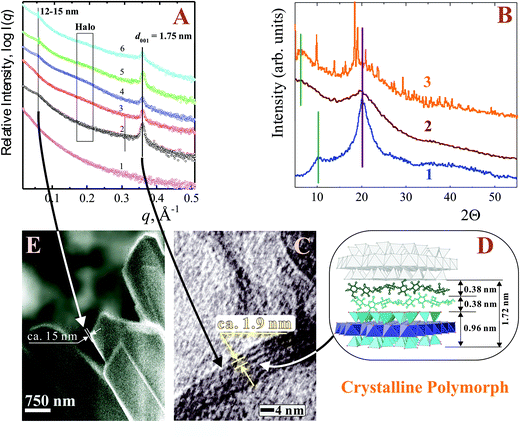 |
| Fig. 4 (A) SAXS pattern of chitosan-saponite films prepared from solutions with 1.5 wt% saponite, 2 wt% glycerol and 0 (1), 0.02 (2), 1.1 (3), 1.4 (4), 1.7 (5) and 2.4 wt% (6) chitosan. (B) TEM image of the chitosan-saponite stack. Additional ones are given in Fig. S4.† (C) SEM image of the microparticles. (D) Schematic (in color) of a structural unit of stack composed of clay nanoplatelet and bimolecular layer of chitosan. | |
Saponite is a nanocrystallite (Fig. 2, inset) that is characterized by reflections at larger angles, which are absent in curve 1 (Fig. 4A). They may be observed in the WAXS pattern in Fig. 5A (curve 1). The first (001) peak corresponds to an interlayer space (basal spacing or d-spacing) of hydrated platelet with a thickness of 1.34 nm. The positions of another five reflections and their assignments are presented in Table 1 and are in a good agreement with the data of ref. 79.
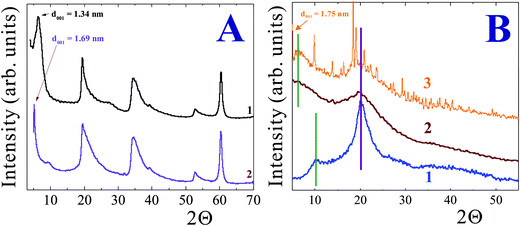 |
| Fig. 5 Powder diffractograms. (A) 1 – film of saponite alone, 2 – saponite with glycerol, (B) 1 – neat chitosan, 2 – film made from polysaccharide alone, 3 – bionanocomposite prepared from a solution with 1.5 wt% saponite, 1 wt% chitosan and 2 wt% glycerol. | |
Table 1 WAXS data for saponite
d0a nm |
d0refb nm |
hklc |
d0 spacing for saponite used in our study. d0ref spacing for saponite obtained in ref. 79. hkl Miller indexes. |
1.340 |
1.529 |
001 |
0.458 |
0.461 |
020, 110 |
0.263 |
0.264 |
200 |
0.256 |
0.256 |
201 |
0.229 |
0.23 |
204 |
0.173 |
0.174 |
311 |
0.153 |
0.153 |
060, 332 |
Curve 2 in Fig. 5 shows a WAXS pattern of a film of saponite with glycerol. There is a shift of the peak characterizing the d-spacing that increases to 1.69 nm. This means that glycerol molecules localize between saponite platelets, attaching to their surface. A similar effect was observed with glycerol in place of water in the case of montmorillonite owing to the solvent intercalation into the interlayer galleries.80
Fig. 5B present WAXS patterns for chitosan, which is a semicrystalline polymer of a natural origin.11,66 This character is obvious from the strong prominent signature peaks at approximately 10° and 20° (curve 1). They characterize the presence of hydrated crystalline form in the neat polysaccharide sample.66,81 When a film was prepared from chitosan in the same manner as the bionanocomposites by adding GL but in the absence of saponite in the solution, one may find a change in the diffractogram presented by curve 2 in Fig. 5B. The peak sharpness at 2θ = 20° was reduced notably and a new broad one at 2θ = 6° appeared instead of the reflex at 2θ = 10°. The mentioned changes indicate that the method used for the film preparation result in some changes in the crystallinity of chitosan. This state stayed put after its combining with the clay nanoparticles. There is a curve 3 in Fig. 5B, in which there are better expressed reflections at 6° and 19°. In addition, the formation of a bionanocomposite film by the method of regulated charging and evaporation-induced self-assembly resulted in the appearance of new numerous sharp reflections in the diffraction pattern. They are representative of the presence of a crystalline polymorph formed by chitosan with saponite. Additional confirmation of the crystallization follows from restricted movement of polysaccharide backbone revealed from observations by microRaman spectroscopy in the ESI file (Fig. S3†).
3.3 Chitosan-clay crystalline polymorph
Comparison of the obtained WAXS pattern (curve 3, Fig. 5B) with those published in the literature for known polymorphs of chitosan reveals its close similarity to the diffractogram of chitosan with hydriodic acid in ref. 82 and 83. At the same time, there was no total coincidence with their data. Few new reflections were found in our measured patterns that were absent in the cited studies and vice versa. Analysis of the details is presented in ESI as Table S1.† One may find 69 independent diffraction reflections in curve 3 (Fig. 5B) that can be indexed to a tetragonal unit cell with dimension lattice parameters of a = b = 10.68 Å and c (fiber axis) = 35.96 Å. This polymorph differs in the main form characterized in ref. 82 and 83 by the fiber period which was found to be 40.77 Å. The difference points to a new crystalline form of polysaccharide formed with nanoplatelets in the self-assembling regime.
Structural organization of the polymorph is gained from an examination of SAXS and electron microscopy. The Bragg peak in the SAXS patterns (curves 2–6, Fig. 4A) correspond to a d-spacing of 1.75 nm (001). It should be emphasized that its position is independent of the chitosan to saponite concentration ratios. The reflex is observed at the same place in the curves. It points to a reproducible, inherent structural organization of chitosan with nanoplatelets.
The periodicity following from the SAXS patterns was confirmed by TEM observations. An image is shown in Fig. 4B, as well as additional ones, in Fig. S4 (ESI†). There is a stack of coplanar aligned saponite nanoplatelets, which can be only visible under the TEM. The periodic dimension is about 1.9 nm being in rather good agreement with that followed from the position of the Bragg peak (Fig. 4A). If one takes into account 0.96 nm for the thickness of single (exfoliated) saponite nanoplatelets,80 and 0.38 nm for the monolayer thickness of chitosan according to ref. 84, it adds up to close to 1.75 nm under the assumption of a bimolecular filling of the gap between clay nanoparticles. In other words, a structural unit of chitosan-saponite polymorph is built up from an inorganic nanoplatelet layer and attached bimolecular sheet of chitosan macromolecules from one side or their monolayer from the each side. This is illustrated by the schematic drawing in Fig. 4D. Rather close similarity of crystalline chitosan-saponite polymorph with that of this polysaccharide with hydriodic acid (see Table S1, ESI†) means that chitosan macromolecules might possess an analogous conformation, aligning symmetrically in an antiparallel manner so that they form a zigzag bimolecular sheet between the saponite nanoplatelets. The polysaccharide structure is stabilized by intermolecular hydrogen bonds.66,83,85 Clay nanoparticles could provide an additional stabilizing effect, such as water and iodide ions, in the chitosan polymorphs through hydrogen bonds and electrostatic interactions between oppositely charged groups.
Another signature reflection appeared at q = 0.055 Å−1 (12–15 nm) in the SAXS patterns after mixing chitosan with saponite (Fig. 4A). It may be assigned to the thickness of nanocrystalline microparticles observed well in Fig. 3E and 4C. As found from these SEM images, it is also equal to about 15 nm. Because the thickness of the structural unit of chitosan-saponite polymorph is estimated to be 1.75 nm, there are ca. 8–9 layers in a nacre-like structure from an alternating sequence of polysaccharide and clay in the stack (Fig. 3D).
The presence of a halo at q = 0.2 Å−1 (Fig. 2A) corresponds to a periodicity of about 4 nm that correlates well with the helical period of 35.96 Å determined for a polymorph of chitosan with saponite. It may be considered as an additional confirmation of the validity of this molecular model for chitosan-saponite polymorph.
Highly ordered nacre-like crystalline structure of chitosan with clay nanoparticles found here can be explained by the method used. Common formation of bionanocomposites includes the mixing of solutions of oppositely charged chitosan and nanoparticles. Fast cooperative electrostatic interactions leads to a heterogeneous precipitate.7 Furthermore, natural clays are only partially exfoliated. There are short stacks of nanoplatelets.80 Bionanocomposites are formed through intercalation of chitosan macromolecules into the spacing between them (see, for example, ref. 7, 33, 84, 86 and 87).
The opposite case is realized in our method combining the polysaccharide charging in situ with evaporation-induced self-assembly (see Fig. 3A–D).7,67,68 There are completely exfoliated nanoplatelets in the initial solution. The chitosan macromolecules are coated onto them with the appearance of charges to yield the building blocks. The latter are brought progressively face-to-face together during drying. The macromolecules not bound electrostatically with nanoplatelets move away from the gap because of the electrostatic repulsion from the adsorbed ones (Fig. 1C). When the process comes to an end, there are only electrostatically attached macromolecules. Their perfect alignment can do much toward self-assembling into the crystalline state.
The unique property of chitosan is its rich polymorphic behavior.66,85 To date, six polymorphs were identified. They are called “tendon-chitosan” because of its preparation from a lobster tendon chitin,65 “annealed” or anhydrous form obtained by annealing at ca. 200 °C,88 whereas “form I”, “form II”, “I-2”, “L-2” were separated from solutions of polysaccharide with transition metals, mineral and organic acids.66,85 An important point is that these polymorphs are found in small amounts if the experiments were conducted under ambient conditions. To prepare them, rigorous conditions of hydrothermal treatment, under high temperature (>200 °C) and pressure, and/or sometimes mechanical stretching are needed. In our experiments, chitosan forms a polymorph with clay nanoparticles, as obvious from the WAXS diffractogram (curve 3, Fig. 5B) at room temperature.
Note that there is no unit microparticle. The polymorph is present as plentiful microparticles with nanosize thickness (Fig. 3E–G). They can be separated under mild conditions, including swelling in water and the treatment of swollen film, in an ultrasound bath as detailed in the Methods section and ESI† file. Thus, the separated nanocrystalline microparticles may be observed in Fig. 6 and S2.† They are highly stable, insoluble in water and organic solvents, which can be explained by the numerous electrostatic interactions and hydrogen bonds between the chitosan macromolecules and clay nanoplatelets.
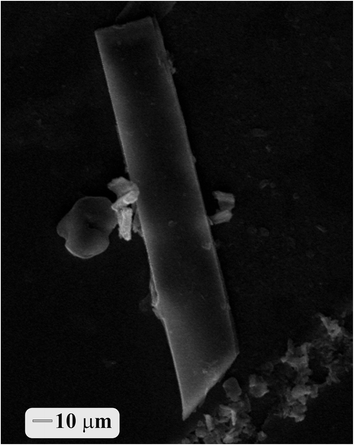 |
| Fig. 6 SEM image of a chitosan-saponite polymorph separated from a bionanocomposite film in which it is found as a nanocrystalline microparticle (see Fig. 3F and G and 4C). Its bottom part was cut when it was placed on a mica surface for observation. One may see a scratch on the underside. Nanocrystalline microparticles have a rectangle shape of which the length can exceed 0.1 mm. | |
4 Conclusions
Bionanocomposite films were prepared by a new one-pot method that includes the initial formation of building blocks through the association of saponite nanoplatelets with chitosan macromolecules charged in situ and their evaporation-induced self-assembly into highly ordered nanocrystalline narrow rectangle microparticles with a uniform thickness and high aspect ratio. Their characterization by the SAXS, WAXS, SEM and TEM revealed the presence of crystalline polymorph unknown for chitosan with nanosized particles before. It possessed a nacre-like structure from alternating layers of two chitosan macromolecules and saponite nanoplatelets. The nanocrystalline narrow rectangle microparticles are stable, insoluble in water and organic solvents owing to the strong polysaccharide – -nanoparticles association through numerous electrostatic interactions and hydrogen bonds that enabled one to separate them by a simple procedure. They might go to work as alternatives to nanofibrils of chitin and cellulose, of which separation meets with severe difficulties in applications such as reinforcing nanofillers in (bio)nanocomposites, packaging, biomedical materials, and biomimetic optical nanomaterials.
Acknowledgements
This study was partially supported by grants 14-03-91700 and 14-03-31350 from the Russian Foundation for Basic Research, program “Dalnii Vostok” and NRF-RFBR Joint Research Program (No. 2013K2A1A7076267) from the Ministry of Science, ICT and Future Planning of Republic of Korea.
Notes and references
- E. Ruiz-Hitzky, in Functional hybrid materials, ed. P. Gomez-Romero and C. Sanchez, Wiley-VCH, Weinheim, 2004, ch. 2, pp. 15–49 Search PubMed.
- S. S. Ray and M. Bousmina, Prog. Mater. Sci., 2005, 50, 962 CrossRef CAS.
- E. Ruiz-Hitzky, M. Darder and P. Aranda, in Bio-inorganic hybrid nanomaterials, ed. E. Ruiz-Hitzky, K. Ariga and Y. M. Lvov, Wiley-VCH, Weinheim, 2008, ch. 1, pp. 1–40 Search PubMed.
- I. Armentano, M. Dottori, E. Fortunati, S. Mattioli and J. M. Kenny, Polym. Degrad. Stab., 2010, 95, 2126 CrossRef CAS.
- S. Pilla, in Handbook of bioplastics and biocomposites engineering applications, ed. S. Pilla, Scrivener, Salem, Massachusetts, 2011, ch. 1, pp. 1–15 Search PubMed.
- C. Aimi and T. Coradin, J. Polym. Sci., Part B: Polym. Phys., 2012, 50, 669 CrossRef.
- Y. Shchipunov, Pure Appl. Chem., 2012, 84, 2579 CrossRef CAS.
- J. Wang, Q. Cheng and Z. Tang, Chem. Soc. Rev., 2012, 41, 1111 RSC.
- S. Hein, K. Wang, W. F. Stevens and J. Kjems, Mater. Sci. Technol., 2008, 24, 1053 CrossRef CAS.
- M. Prabaharan and R. Jayakumar, Adv. Polym. Sci., 2011, 244, 1 CrossRef CAS.
- S. Hirano, in Ullmann's encyclopedia of industrial chemistry, Wiley-VCH, Weinheim, 2000, pp. 471–481 Search PubMed.
- K. Kurita, Mar. Biotechnol., 2006, 8, 203 CrossRef CAS PubMed.
- P. A. Sandford, in Chitin and chitosan. Sources, chemistry, biochemistry, physical properties and applications, ed. G. Skjak-Braek, T. Anthonsen and P. A. Sandford, Elsevier Applied Science, London, 1989, ch. 3, pp. 51–69 Search PubMed.
- G. A. F. Roberts, Chitin chemistry, MacMillan Education, Basingstoke, Hampshire, UK, 1992 Search PubMed.
- T. D. Rathke and S. M. Hudson, J. Macromol. Sci., Rev. Macromol. Chem. Phys., 1994, C34, 375 CrossRef CAS.
- E. Khor, Chitin: fulfilling a biomaterials promise, Elsevier Science, Amsterdam, 2001 Search PubMed.
- M. Rinaudo, Prog. Polym. Sci., 2006, 31, 603 CrossRef CAS.
- C. K. S. Pillai, W. Paul and C. P. Sharma, Prog. Polym. Sci., 2009, 34, 641 CrossRef CAS.
- M. Z. Elsabee and E. S. Abdou, Mater. Sci. Eng., C, 2013, 33, 1819 CrossRef CAS PubMed.
- R. N. Tharanathan and F. S. Kittur, Crit. Rev. Food Sci. Nutr., 2003, 43, 61 CrossRef CAS PubMed.
- H. Ueno, T. Mori and T. Fujinaga, Adv. Drug Delivery Rev., 2001, 52, 105 CrossRef CAS PubMed.
- F. Shahidi and R. Abuzaytoun, Adv. Food Nutr. Res., 2005, 49, 93 CrossRef CAS PubMed.
- M. N. V. R. Kumar, R. A. A. Muzzarelli, C. Muzzarelli, H. Sashiwa and A. J. Domb, Chem. Rev., 2004, 104, 6017 CrossRef PubMed.
- T. G. S. Denis, T. Dai, Y. Y. Huang and M. R. Hamblin, in Chitosan-based systems for biopharmaceuticals: delivery, targeting and polymer therapeutics, ed. B. Sarmento and J. das Neves, Wiley, Chichester, 2012, ch. 22, pp. 429–450 Search PubMed.
- T. Cunha, B. Teixeira, B. Santos, M. Almeida, G. Dias and J. das Neves, in Chitosan-based systems for biopharmaceuticals: delivery, targeting and polymer therapeutics, ed. B. Sarmento and J. das Neves, Wiley, Chichester, 2012, ch. 5, pp. 76–92 Search PubMed.
- F. Croisier and C. Jerome, Eur. Polym. J., 2013, 49, 780 CrossRef CAS.
- Y. A. Shchipunov and I. V. Postnova, Compos. Interfaces, 2009, 16, 251 CrossRef CAS.
- E. V. Shumilina and Y. A. Shchipunov, Colloid J., 2002, 64, 372 CrossRef CAS.
- C. Schatz, J. M. Lucas, C. Viton, A. Domard, C. Pichot and T. Delair, Langmuir, 2004, 20, 7766 CrossRef CAS PubMed.
- A. Drogoz, L. David, C. Rochas, A. Domard and T. Delair, Langmuir, 2007, 23, 10950 CrossRef CAS PubMed.
- C. Peniche, W. Arguelles-Monal and F. M. Goycoolea, in Monomers, polymers and composites from renewable resources, ed. N. B. Mohamed and G. Alessandro, Elsevier, Amsterdam, 2008, ch. 25, pp. 517–542 Search PubMed.
- M. Darder, M. Colilla and E. Ruiz-Hitzky, Appl. Clay Sci., 2005, 28, 199 CrossRef CAS.
- J. W. Rhim and P. K. W. Ng, Crit. Rev. Food Sci. Nutr., 2007, 47, 411 CrossRef CAS PubMed.
- M. Liu, Y. Zhang, C. Wu, S. Xiong and C. Zhou, Int. J. Biol. Macromol., 2012, 51, 566 CrossRef CAS PubMed.
- J. Berger, M. Reist, J. M. Mayer, O. Felt and R. Gurny, Eur. J. Pharm. Biopharm., 2004, 57, 35 CrossRef CAS PubMed.
- J. Vincent, Structural biomaterials, Princeton University Press, Princeton, 1990 Search PubMed.
- L. Addadi and S. Weiner, Angew. Chem., Int. Ed., 1992, 31, 153 CrossRef.
- R. Lakes, Nature, 1993, 361, 511 CrossRef.
- S. Mann, Nature, 1993, 365, 499 CrossRef CAS.
- Handbook of biomineralization. Biological aspects and structure formation, Wiley-VCH, Weinheim, 2007 Search PubMed.
- P. Fratzl and R. Weinkamer, Prog. Mater. Sci., 2007, 52, 1263 CrossRef CAS.
- M. A. Meyers, P. Y. Chen, A. Y. M. Lin and Y. Seki, Prog. Mater. Sci., 2008, 53, 1 CrossRef CAS.
- G. M. Luz and J. F. Mano, Philos. Trans. R. Soc., A, 2009, 367, 1587 CrossRef CAS PubMed.
- B. Bhushan, Philos. Trans. R. Soc., A, 2009, 367, 1445 CrossRef CAS PubMed.
- Materials design inspired by nature: function through inner architecture, ed. P. Fratzl, J. W. C. Dunlop and R. Weinkamer, RCS Publishing, Cambridge, 2013 Search PubMed.
- C. M. D. Gregoire, J. Biophys. Biochem. Cytol., 1957, 3, 797 CrossRef CAS PubMed.
- N. Watabe, J. Ultrastruct. Res., 1965, 12, 351 CrossRef CAS PubMed.
- S. Weiner and W. Traub, Phil. Trans. R. Soc. Lond. B, 1984, 304, 425 CrossRef CAS.
- Y. Levi-Kalisman, G. Falini, L. Addadi and S. Weiner, J. Struct. Biol., 2001, 135, 8 CrossRef CAS PubMed.
- H. Imae and Y. Oaki, in Handbook of biomineralization, ed. P. Behrens and E. Bauerlein, Wiley-VCH, Weinheim, 2007, ch. 5, pp. 89–197 Search PubMed.
- F. Barthelat, H. Tang, P. Zavattieri, C. Li and H. Espinosa, J. Mech. Phys. Solids, 2007, 55, 306 CrossRef CAS.
- M. A. Meyers, J. McKittrick and P. Y. Chen, Science, 2013, 339, 773 CrossRef CAS PubMed.
- P. Podsiadlo, Z. Tang, B. S. Shim and N. A. Kotov, Nano Lett., 2007, 7, 1224 CrossRef CAS PubMed.
- L. J. Bonderer, A. R. Studart and L. J. Gauckler, Science, 2008, 319, 1069 CrossRef CAS PubMed.
- H. B. Yao, Z. H. Tan, H. Y. Fang and S. H. Yu, Angew. Chem., Int. Ed., 2010, 49, 10127 CrossRef CAS PubMed.
- K. W. Putz, O. C. Compton, M. J. Palmeri, S. B. T. Nguyen and L. Brinson, Adv. Funct. Mater., 2010, 20, 3322 CrossRef CAS.
- X. Wang, H. Bai, Z. Yao, A. Liu and G. Shi, J. Mater. Chem., 2010, 20, 9032 RSC.
- Q. Cheng, L. Jiang and Z. Tang, Acc. Chem. Res., 2014, 47, 1256 CrossRef CAS PubMed.
- J. Wang, Q. Cheng, L. Lin and L. Jiang, ACS Nano, 2014, 8, 2739 CrossRef CAS PubMed.
- S. S. Wang, Y. Q. Shu, B. L. Liang, L. C. Gao, M. Gao, P. G. Yin and L. Guo, Chin. J. Polym. Sci., 2014, 32, 675 CrossRef CAS.
- Q. Cheng, J. Duan, Q. Zhang and L. Jiang, ACS Nano, 2015, 9, 2231 CrossRef CAS PubMed.
- P. Das, J. M. Malho, K. Rahimi, F. H. Schacher, B. Wang, D. E. Demco and A. Walther, Nat. Commun., 2015, 6, 5967, DOI:10.1038/ncomms6967.
- B. Zhu, N. Jasinski, A. Benitez, M. Noack, D. Park, A. S. Goldmann, C. Barner-Kowollik and A. Walther, Angew. Chem., Int. Ed., 2015, 54, 8653 CrossRef CAS PubMed.
- K. Okumura, MRS Bull., 2015, 40, 333 CrossRef.
- G. L. Clark and A. F. Smith, J. Phys. Chem., 1935, 40, 863 CrossRef.
- W. Maniukiewicz, in Chitin, chitosan, oligosaccharides and their derivatives: biological activities and applications, ed. S. K. Kim, CRC Press, Boca Raton, 2011, ch. 8, pp. 83–94 Search PubMed.
- Y. A. Shchipunov, N. Ivanova and V. Silant'ev, Green Chem., 2009, 11, 1758 RSC.
- Y. A. Shchipunov, V. E. Silant'ev and I. V. Postnova, Colloid J., 2012, 74, 627 CrossRef CAS.
- Y. A. Shchipunov, S. A. Sarin, V. E. Silant'ev and I. V. Postnova, Colloid J., 2012, 74, 636 CrossRef CAS.
- I. Postnova, S. Sarin, V. Silant'ev, C. S. Ha and Y. Shchipunov, Pure Appl. Chem., 2015, 87, 793 CrossRef CAS.
- E. Khor, Curr. Opin. Solid State Mater. Sci., 2002, 6, 313 CrossRef CAS.
- A. Domard and M. Domard, in Polymeric biomaterials, ed. S. Dumitriu, Marcel Dekker, New York, 2002, ch. 9, pp. 196–218 Search PubMed.
- M. Dash, F. Chiellini, R. M. Ottenbrite and E. Chiellini, Prog. Polym. Sci., 2011, 36, 981 CrossRef CAS.
- W. M. Bruck, J. W. Slater and B. F. Carney, in Chitin, chitosan, oligosaccharides and their derivatives : biological activities and applications, ed. S. K. Kim, CRC Press, Boca Raton, 2011, ch. 2, pp. 11–23 Search PubMed.
- M. F. Brigatti, E. Galan and B. K. G. Theng, in Handbook of clay science. Developments in clay science, ed. F. Bergaya, B. K. G. Theng and G. Lagaly, Elsevier, Amsterdam, 2006, ch. 2, pp. 19–86 Search PubMed.
- J. D. Dziezak, in Encyclopedia of food science and nutrition, ed. B. Caballero, Academic Press, New York, 2004, pp. 12–17 Search PubMed.
- J. Zhu, M. Tian, Y. Zhang, H. Zhang and J. Liu, Chem. Eng. J., 2015, 265, 184 CrossRef CAS.
- D. F. Xie, V. Martino, P. Sangwan, C. Way, G. A. Cash, E. Pollet, K. M. Dean, P. J. Halley and L. Averous, Polymer, 2013, 54, 3654 CrossRef CAS.
- H. Suquet, C. de la Calle and H. Pezerat, Clays Clay Miner., 1975, 23, 1 CAS.
- L. A. Utracki, Clay-containing polymeric nanocomposites, Rapra Technology, Shawbury, UK, 2004 Search PubMed.
- J. Kumirska, M. Czerwicka, Z. Kaczynski, A. Bychowska, K. Brzozowski, J. Thoeming and P. Stepnowski, Mar. Drugs, 2010, 8, 1567 CrossRef CAS PubMed.
- K. Ogawa and S. Inukai, Carbohydr. Res., 1987, 160, 425 CrossRef CAS.
- A. Lertworasirikul, K. Noguchi, K. Ogawa and K. Okuyama, Carbohydr. Res., 2004, 339, 835 CrossRef CAS PubMed.
- M. Darder, M. Colilla and E. Ruiz-Hitzky, Chem. Mater., 2003, 15, 3774 CrossRef CAS.
- K. Okuyama, K. Noguchi, M. Kanenari, T. Egawa, K. Osawa and K. Ogawa, Carbohydr. Polym., 2000, 41, 237 CrossRef CAS.
- E. Ruiz-Hitzky, P. Aranda and M. Darder, in Kirk-Othmer Encyclopedia of Chemical Technology, Wiley, 2008, pp. 1–28 Search PubMed.
- F. Chivrac, E. Pollet and L. Averous, Mater. Sci. Eng., R, 2009, 67, 1 CrossRef.
- K. Ogawa, S. Hirano, T. Miyanishi, T. Yui and T. Watanabe, Macromolecules, 1984, 17, 973 CrossRef CAS.
Footnote |
† Electronic supplementary information (ESI) available. See DOI: 10.1039/c6ra02996e |
|
This journal is © The Royal Society of Chemistry 2016 |
Click here to see how this site uses Cookies. View our privacy policy here.