DOI:
10.1039/C6RA02965E
(Paper)
RSC Adv., 2016,
6, 31840-31849
Preparation of ORMOSIL nanoparticles conjugated with vitamin D3 analogues and their biological evaluation†
Received
1st February 2016
, Accepted 21st March 2016
First published on 24th March 2016
Abstract
Dye-doped, 90 nm diameter, multifunctional organically modified silica (ORMOSIL) nanoparticles were prepared by condensation of vinyltriethoxysilane within surfactant-stabilized microemulsions and conjugated with several vitamin D3 derivatives. For this, vitamin D analogues possessing at least one hydroxyl group derivatized as vinylcarbonate have been synthesized. These analogues were conjugated to ORMOSIL nanoparticles through the carbonate function, forming carbamate derivatives of vitamin D nanoparticles. The interaction between these nanoparticles and human serum albumin, which is one of the serum transporters of the insoluble vitamin D3, was investigated by surface plasmon resonance. The results obtained revealed that this approach allows obtaining water-soluble vitamin D3 derivatives, which maintain their full protein binding ability.
Introduction
Over the last decade, silica nanoparticles, highly cross-linked inorganic polymers formed by base-catalysed polymerization of silicates in water, have emerged as one of the most intensively studied nanomaterials for biomedical applications.1 Such success is not due to any peculiar nanosize-related property,2 but to the possibility of obtaining topologically and functionally complex structures. This polymeric structure allows to accommodate organic molecules either in their interior, on their surface or within pores.3 Moreover, it is possible to process them into very complex structures, including mesoporous particles,1c,4 nanoshells5 and multi-shell particles, and to selectively place the organic payload in each of such subcompartments.3,6 Such versatility has opened the way to prepare several sophisticated systems, such as gated porous particles for controlled release,1a,f multimodal imaging and delivery agents,1 or chemical sensors.7
Recently, we developed8 a new synthetic protocol for the one-pot preparation of multifunctional PEGylated organically modified silica (ORMOSIL9) nanoparticles. The idea was based on the synthesis of ORMOSIL nanoparticles by base-catalysed condensation of vinyltriethoxysilane (VTES, Scheme 1) in aqueous solution containing a surfactant, which was first proposed by Prasad and co-workers in 2003 for the development of photosensitizer delivery agents in photodynamic therapy,10 and subsequently employed to prepare nanoparticles for several other relevant applications.11
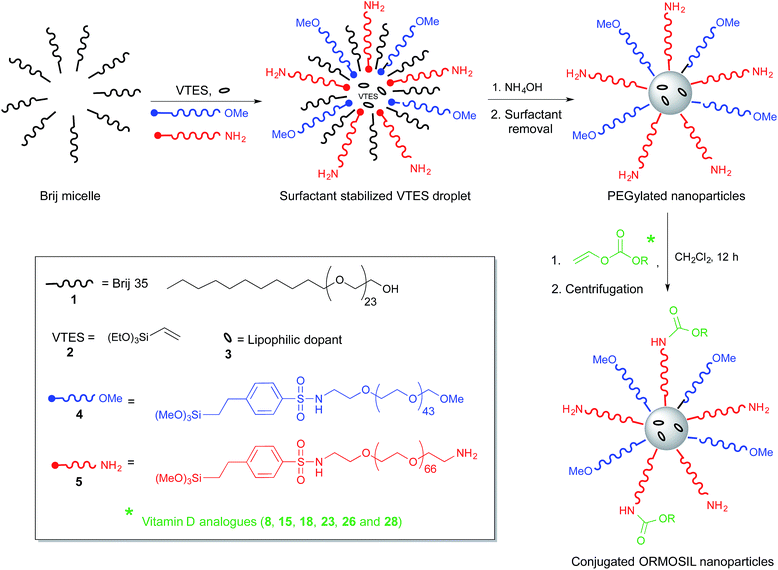 |
| Scheme 1 One-pot synthesis of doped, PEGylated and functional ORMOSIL nanoparticles. Any of the components indicated in the caption, with the exception of the surfactant (1), may be omitted to prepare nanoparticles with different features. | |
In the Prasad synthesis, the nanoparticles formation occurs in the hydrophobic core of surfactant-stabilized microemulsions. We realized that such microemulsions could act not only as nanocontainers where the organosilane polymerization is confined, but also as a template that pre-organizes the nanoparticle components, placing them precisely in the sites where they are driven by their hydrophobic/hydrophilic balance. Water insoluble molecules (dyes, photosensitizers or drugs) will locate themselves in the emulsion oil core, resulting entrapped in the polymerized ORMOSIL matrix. Amphiphilic species will by the contrary locate at the oil/water interface and will form, if capable to copolymerize with the ORMOSIL precursors, a surface functionalization layer.11a Taking full advantage of such approach, we recently showed8,12 that densely PEGylated nanoparticles can be prepared. Functional groups for the subsequent conjugation with targeting agents can be introduced in the PEG coating during the nanoparticle synthesis. The dense PEG coating obtained strongly improve the nanoparticles biocompatibility by reducing their toxic13 and pro-coagulant14 properties and avoiding capture by immune system cells,8 but also minimizes uptake by cells.12
However, in the preparation of such multifunctional entity all the possible interactions between the components should be taken into account. Indeed, dense PEGylation of the nanoparticles minimizes their toxicity and RES capture, but also uptake by cells.12 Such unfavorable feature could enable higher selectivity when active targeting strategies, i.e. nanoparticle conjugation with specific directing agents, minimizes unwanted uptake by healthy cells. However, there is also the possibility of adverse interactions between the targeting molecules and the stealthing layer, which forms a low-polarity pseudophase around the nanoparticle core. Likely, steric hindrance or even masking of the conjugated ligands by the dense PEG coating could prevent their efficient interaction with the corresponding cellular receptors. A deeper understanding of the nanoparticle structure and the possible mutual interactions/interferences between the particles and the targeting agents would be very important in designing simple but effective delivery agents.
Vitamin D3 (6, Chart 1) plays a significant role in the endocrine system through its hormonally active form 1α,25-dihydroxyvitamin D3 [1α,25-(OH)2-D3, 7], exhibiting a much broader spectrum of biological activities than the ones related to calcium and phosphorus homeostasis.15
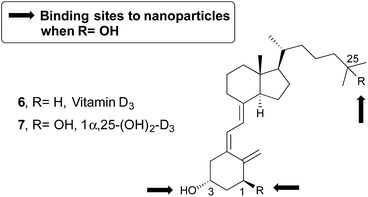 |
| Chart 1 Structure of vitamin D3 (6) and its hormonally active form 7. Marked with arrows are the connection points to the nanoparticles. | |
Studies revealed that this hormone is also involved in cell proliferation and differentiation, and immune regulation.16 The required physiological doses of 1α,25-(OH)2-D3 lead to calcemic side effects such as hypercalcemia, hypercalciuria and bone decalcification. These findings are a major obstacle in the clinical development of this compound.
In order to avoid these limitations, we have envisaged that the selective delivery of the vitamin D analogues in their targets can overcome these disadvantages. Thus, the analogues can be transported to their specific receptor linked to a nanoparticle and this could prevent or reduce their toxicity. Most of the vitamin D derivatives shown three potential binding sites, that is, the hydroxyl groups present in positions C1, C3, and C25 (Chart 1). In this paper, we report a detailed investigation of the ability of hydrophobic molecules, namely vitamin D or analogues, grafted to the PEG coating layer of ORMOSIL nanoparticles, to interact with one of their transport proteins, the Human Serum Albumin (HSA).
Results and discussion
Synthesis of vitamin D derivatives
Vitamin D3 is an ideal candidate to study the effect of dense PEG coating on the ability of small targeting molecules to interact with their biological receptor. Indeed, although essential for different biological process, this molecule is essentially insoluble in water. As a consequence, it is transported in the bloodstream by suitable proteins, including HSA and the structurally related vitamin D3 Binding Protein (DBP), and recognized on the cell membrane by the specific Vitamin D3 Receptor (VDR). Among these proteins, albumin is well known, robust and readily available and can provide a good model to evaluate how the conjugation of vitamin D with PEG-ORMOSIL nanoparticles may affect its interaction with the transport proteins. Remarkably, interaction of albumin for different vitamin D3 derivatives, albeit weaker than that of the specific DBP, follows the same affinity trend (Table 1), confirming that albumin can be used as model of more specific receptors.
Table 1 Vitamin D3 and metabolites affinity by DBP and HSA17
Entry |
K
DBP (μM−1) |
K
HSA (μM−1) |
1/KDBP (μM) |
1/KHSA (μM) |
Vitamin D3 |
11 |
0.011 |
0.085 |
91 |
25-OH-D3 |
700 |
0.600 |
0.001 |
1.7 |
1α,25-(OH)2-D3 |
37 |
0.054 |
0.027 |
19 |
Five nanoparticle-conjugable vitamin D3 derivatives were prepared in order to explore the effect of changing the nanoparticle conjugation site on the interaction between vitamin D3 and albumin. All five derivatives featured a vinylcarbonate group respectively in positions C1, C3, or C25 of the polycyclic skeleton. The vinyl carbonate moieties allow the conjugation with the nanoparticles via condensation with primary amino groups inserted in the nanoparticle coating layer, resulting in the formation of a stable carbamate derivative.
The synthesis of vitamin D3 derivative 8 involves alkoxycarbonylation of vitamin D3 with vinyl chloroformate in the presence of Et3N and CH2Cl2 as solvent (Scheme 2). The approach to prepare the C1 or C3 derivatives of 1α-hydroxyvitamin D3 started with the vitamin D3-sulfur dioxide adduct 9, which were prepared in almost quantitative yield by the reaction of vitamin D3 with liquid sulfur dioxide.18 The resulting mixture of adducts was treated with tert-butyldimethylsilyl chloride-imidazole to give the silyl protected ether 10. Thermolytic desulfonylation in the presence of NaHCO3 gives the 5E isomer 11 in 98% yield. Hydroxylation of the later with SeO2 and N-methylmorpholine N-oxide (NMO) in methanol at reflux afforded with high regio- and steroselectivity the 1α-hydroxylated products in approximately a 90
:
10 ratio of the 1α/1β isomers.19 This stereoselectivity is dictated by a strong predisposition for an equatorial conformation of the 3-O-TBDMS group in the selenoxide intermediate. The 5E vitamin D derivatives 12 and 13 were transformed to the 5Z configuration by photosensitized isomerization. After column chromatography, 14 was isolated in 55% yield. The subsequent treatment with vinyl chloroformate gave the desired C1-substituted derivative 15.
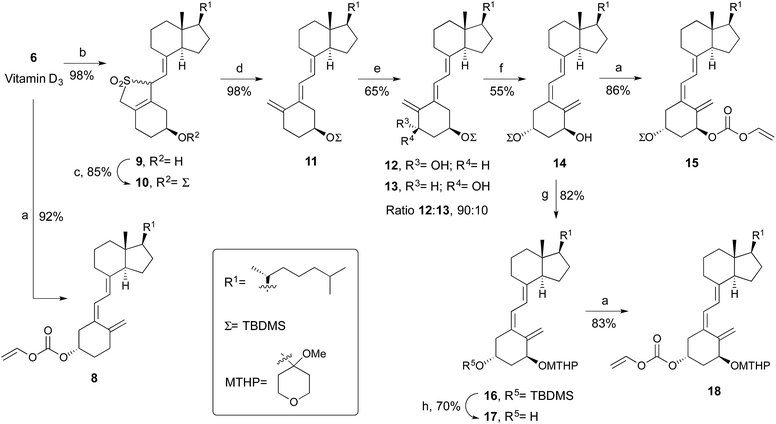 |
| Scheme 2 Synthesis of vitamin D3 and 1α-OH-D3 carbonate derivatives 8, 15, and 18. Reagents and conditions: (a) vinyl chloroformate, Et3N, CH2Cl2, rt, 12 h; (b) SO2 (g), −50 °C to −20 °C, 20 min; (c) TBDMSCl, imidazole, CH2Cl2, rt, 12 h; (d) NaHCO3, EtOH, reflux, 3 h; (e) SeO2, NMO, MeOH, reflux, 2 h; (f) hν, anthracene, Et3N, toluene, rt, 3 h; (g) MDHP, PPTS, CH2Cl2, rt, 4 h; (h) TBAF, THF, 0 °C to rt, 4 h. | |
To access the C3-modified analogue of 1α-hydroxyvitamin D3, orthogonal protection of the hydroxyl groups of 14 is needed. Thus, 4-methoxytetrahydropyran-4-yl acetal protecting group is used for the 1α-hydroxyl. Selective TBDMS deprotection and alkoxycarbonylation of the C3 position provided derivative 18 (Scheme 2).
The triene skeleton of the corresponding 25-hydroxyvitamin D3 analogues was constructed by a Horner coupling developed by Lythgoe20 between the phosphine oxide 19 (ref. 21) and the 25-ethoxymethyl ether Grundmann's ketone derivative 20 (ref. 22) (Scheme 3). The coupling reaction proceeded in excellent yield, and deprotection with TBAF of 21 and alkoxycarbonylation of the C3-hydroxyl group afforded analogue 23.23 On the other hand, treatment of 21 with (−)-CSA yields 24, then selective protection of the C3-hydroxyl group of the latter, and introduction of the vinyloxycarbonyl function at C25 generated compound 26.
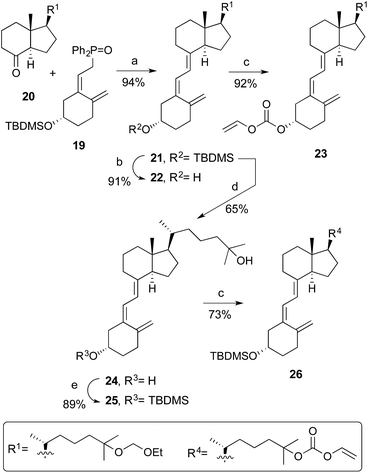 |
| Scheme 3 Synthesis of 25-OH-D3 carbonate derivatives 23 and 26. Reagents and conditions: (a) nBuLi, THF, −78 °C, 6 h; (b) TBAF, THF, 0 °C to rt, 7 h; (c) vinyl chloroformate, Et3N, CH2Cl2, rt, 12 h; (d) (−)-CSA, MeOH, rt, 4 h; (e) TBDMSCl, imidazole, CH2Cl2, rt, 12 h. | |
Finally, derivative 28 (Scheme 4), resulting from the coupling of 8 with 2-[2-(2-methoxyethoxy)ethoxy]ethan-1-amine, was prepared as conjugation model in order to assess the effect of the introduction of the PEG spacer on the intrinsic affinity of the nanoparticle for albumin.
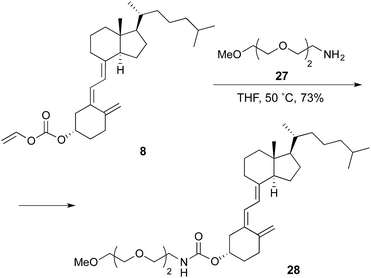 |
| Scheme 4 Synthesis of vitamin D3 carbamate derivative 28. | |
Nanoparticule conjugation
The synthesis of the PEG-ORMOSIL nanoparticles simply requires the polymerization of vinyltriethoxysilane (2, VTES, Scheme 1), promoted by a base as ammonia, in a water solution containing the surfactant Brij 35 (1). Nanoparticles size is controlled by the concentration of VTES in the reaction mixture. In our case, 90 nm-diameter nanoparticles were prepared by setting the VTES concentration to 100 mM. Coating with PEG2000 was obtained by adding to the reaction mixture the derivative 4 (3 mM), which locates at the microemulsion oil/water interphase and copolymerizes with the ORMOSIL matrix. The addition of the PEG-trialkoxysilane derivative 5 (0.3 mM), bearing a terminal amino group at the end of a PEG3000 spacer, introduced the reactive groups necessary for conjugation of the vitamin D3 derivatives.
In our previous work, conjugation of nanoparticles with targeting agents was performed directly in the reaction mixture, or in buffered aqueous solutions after nanoparticles purification. In both cases, the competition of water and other nucleophiles present in the solvent forced us to use large excesses of conjugation derivatives. Such protocol was not compatible with the low water solubility of vitamin D3 and its derivatives, and also with the little amount available. For this reason, we decided to investigate a different strategy. After purification, PEG-ORMOSIL nanoparticles coated with 4 and 5 were dried under vacuum to remove all the water and then dissolved in anhydrous CH2Cl2. Conjugation with vitamin D3 derivatives was performed by addition of one equivalent (with respect to the total amount of primary amino group present, see vide infra) of derivatives 8, 15, 18, 23, and 26, and incubation for 12 h (last process, Scheme 1). After reaction, solvent was evaporated, nanoparticles dissolved in water and filtered to remove unconjugated vitamin D3 residues, and finally purified by centrifugation dialysis with 10
000 Da cutoff membranes.
Nanoparticule characterization
The vitamin D3 conjugated ORMOSIL nanoparticles, namely ORMOSIL-8, -15, -18, -23, and -26, were characterized by Dynamic Light Scattering (DLS), zeta potential measurements, UV-Vis absorption, thermogravimetric analysis (TGA), Nuclear Magnetic Resonance (NMR), and Transmission Electron Microscopy (TEM).
DLS (Table 2) and TEM (Fig. 1) analyses confirm that the average diameter of the nanoparticles is around 90 nm. Remarkably, no aggregation or degradation occurs due to solvent evaporation and dispersion in organic solvent. Moreover, TEM images show a homogeneous distribution.
Table 2 Hydrodynamic diameter and Z potential values for the conjugated nanoparticles
Entry |
Nanoparticles |
Sizea (nm) |
Z potentialb (mV) |
Data obtained by DLS.
In parentheses are Z potential of nanoparticles before conjugation.
|
1 |
No conjugation |
80 ± 10 |
|
2 |
ORMOSIL-8 |
97 ± 10 |
−3.40 (−3.68) |
3 |
ORMOSIL-15 |
85 ± 10 |
−2.57 (−2.92) |
4 |
ORMOSIL-18 |
93 ± 10 |
−2.68 (−2.92) |
5 |
ORMOSIL-23 |
75 ± 10 |
+2.61 (+2.95) |
6 |
ORMOSIL-26 |
75 ± 10 |
+2.75 (+3.10) |
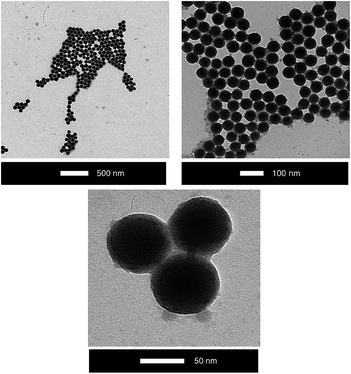 |
| Fig. 1 TEM images of conjugated nanoparticles to different scales. | |
The Z-potential (in PBS buffer, pH 7.4) is in the range between −4 and +3 mV (Table 2). Z-Potential measures the electric potential of the double layer at the location of the slipping plane and provides information about the surface charge of a colloid. As we already reported, ORMOSIL-PEG nanoparticles coated only with 4 commonly have Z-potential values around −6 mV. Such figures are quite close to neutrality and likely indicate that most of the nanoparticle surface is coated by PEG or vinyl groups, with only a few deprotonated silanol groups responsible for the small negative charge.8 The values measured here are slightly less negative than those of nanoparticles coated only with 4, and in several cases they turn positive. This effect, although very small, could be due to the presence of the protonated amino groups in the PEG coating.
Successful purification is confirmed by 1H NMR analysis. Two different pulse sequences were used: first is a solvent suppression sequence, which allows to remove only the very intense signal of water from the spectrum while all the remaining signals arising from molecules present in the sample remain visible; the second is a translational diffusion filter that isolates the signal of large, slow-diffusing species from those of fast-diffusing molecular species.24 Remarkably, both the water suppression and the diffusion filtered spectra are identical and show only the presence of the signals relative to the PEG moiety: the methylene protons at 3.7 ppm and the terminal methyl at 3.3 ppm. This indicates that all the organic contaminants have been successfully removed from the sample, since all the detected signals arise from slow diffusing species, and that the PEG chains are really grafted to the nanoparticles and share their reduced diffusion coefficient. The proton signals of the hydrophobic portion of 4 are not detected since they are so close to the nanoparticles surface, or even embedded inside it, and the signals are too broadened. Also the signals relative to vitamin D3 derivatives are not visible. This fact could be ascribed to two different, and probably concurrent, reasons: at first, the PEG3000 spacer is likely folded to locate the hydrophobic vitamin D3 moieties inside the PEG monolayer, causing a relevant signal broadening due to reduced mobility; second, the intensity of the signals relative to vitamin D3 moieties, which decorate only a small fraction of the total coating molecules, is too low for the NMR sensitivity.
Vitamin D3 conjugation was hence further investigated via UV-Vis spectroscopy and reactivity tests. Amino groups present on the surface of the nanoparticles were quantified using the fluorescamine test. This reagent reacts with primary amines to give a fluorescent product. Integration of the emission intensity of the samples after fluorescamine addition and comparison with a calibration curve allowed to determine the concentration of amine groups in the nanoparticles batches. In all the samples, the concentration of amine groups decreased by about 40% after conjugation with the vitamin D3 derivatives as consequence of their conversion into carbamate groups. This result demonstrates the efficiency of the conjugation protocol used with respect to the previous ones, where similar amount of conjugation could be reached only by consuming 20-fold larger amounts of targeting agents.
It is noteworthy that when the carbamate is formed through one of the A-ring positions, about 30–40% of the free amino groups are combined (entries 1–4 and 7–10, Table 3). If the reaction is carried out at 50 °C, conjugation increases, but the difference is not very large (entries 5–6, Table 3).
Table 3 Conjugation of nanoparticles according to fluorescamine test
Entry |
Nanoparticles |
[RNH2]a (μM) |
Conjugation (%) |
Values obtained for nanoparticles water solutions of 4.3 mg mL−1.
No conjugated nanoparticles.
Conjugation at 50 °C in a sealed tube.
|
1 |
ORMOSILb |
85 |
38% |
2 |
ORMOSIL-8 |
53 |
3 |
ORMOSILb |
95 |
43% |
4 |
ORMOSIL-15 |
54 |
5 |
ORMOSILb |
102 |
48% |
6 |
ORMOSIL-15c |
53 |
7 |
ORMOSILb |
91 |
45% |
8 |
ORMOSIL-18 |
50 |
9 |
ORMOSILb |
84 |
30% |
10 |
ORMOSIL-23 |
59 |
11 |
ORMOSILb |
84 |
61% |
12 |
ORMOSIL-26 |
33 |
Also, it is of note that when the conjugation takes place through C25 carbonate position, the binding percentage increases considerably (entries 11–12, Table 3). This fact can be attributed to smaller steric constraints when the carbamate group is formed through the side chain.
Further evidence for the successful conjugation was provided by UV-Vis analyses. Vitamin D3 derivatives present a characteristic absorption band at 268–272 nm arising from the conjugated π system. On the other hand, PEG-ORMOSIL nanoparticles feature only an absorption band at 230 nm due to the benzensulfonamide moiety of 4 and 5. In the spectra of nanoparticles ORMOSIL-8, -15, -18, -23, and -26 a shoulder around 270 nm is clearly visible, confirming the presence of the vitamin D3 derivatives in the nanoparticle samples.
Data in Table 3 allow estimating that 4.3 mg mL−1 samples of conjugated nanoparticle contain vitamin D3 at a concentration ranging in the interval 30–50 μM. Nanoparticle concentration can be increased up to at least 20 mg mL−1, resulting in 100–300 μM vitamin D3 concentration. Such figures are quite greater than the water solubility of vitamin D3 and its derivatives, which is around 1–20 μM, and confirm that PEG-ORMOSIL nanoparticles conjugation is an effective method to increase the water solubility of lipophilic molecules.
Biological evaluation
The availability of the conjugated vitamin D3 to interact with HSA was assessed by Surface Plasmon Resonance (SPR) analysis, which allows for the direct measurement of the binding events. HSA was immobilized on a dextrane-coated gold chip via amide coupling (about 10 ng mm−2) and exposed to increasing concentrations of vitamin D3, derivative 28, and nanoparticles (5% of DMSO was present in the buffers to ensure the full solubility of vitamin D3 and 28).
In case of molecular ligands, as vitamin D3 and 28, the sensorgrams collected (multi cycle kinetics) indicated a fast binding and release of the molecule from the chip (squared shape of the binding-release profiles). Signal intensity was quite low [0–20 reflectance units (RU)] as expected for ligands with small molecular weight. Fitting of the RU values versus ligand concentration data using a 1
:
1 binding model provided the Kd values of 7 × 10−5 M and 1.8 × 10−4 M, respectively for vitamin D3 and 28. The value obtained for vitamin D3 is very close to that reported in the literature (see Table 1), confirming that the immobilization of HSA on the dextran chip did not alter its binding ability. HSA affinity for derivative 28 is only slightly (2.6 fold) lower than that for vitamin D3, indicating that the introduction of the carbamate group and the PEG spacer has a minor effect on the recognition by the protein.
Experiments performed with nanoparticles produced quite different binding profiles (single cycle kinetics). At first, unconjugated nanoparticles did not reveal any interaction with HSA, as expected for densely PEGylated nanoparticles. On the other hand, vitamin D-ORMOSIL nanoparticles revealed a relatively strong binding to HSA. In this case, association and dissociation processes resulted slower than those observed with vitamin D3 and 28, likely because of the lower diffusion coefficient and the bulkiness of the nanoparticles.
Kinetic profiles could be nicely fitted with a 1
:
1 binding kinetic model. Fitting results indicated that nanoparticles binding is characterized by a relatively fast association (kon ∼ 100 M−1 s−1) and slow dissociation rates (koff ∼ 5 × 10−4 s−1), with very small differences between the different conjugated vitamin D derivatives (Table 4).
Table 4 Interaction of conjugated nanoparticles with HSA
Entry |
Nanoparticles |
k
on
a (M−1 s−1) |
k
off
b (s−1) |
k
off/konc (μM) |
K
d
d (μM) |
Association rate constant.
Dissociation rate constant.
Dissociation rate constant calculated from kinetic data.
Thermodynamic dissociation constant.
|
1 |
Vitamin D3 (6) |
1783 |
0.0346 |
19 |
70 |
2 |
28
|
6516 |
0.4547 |
70 |
180 |
3 |
ORMOSIL-8 |
127 |
7.5 × 10−4 |
6 |
40 |
4 |
ORMOSIL-15 |
174 |
3.9 × 10−4 |
2 |
32 |
5 |
ORMOSIL-18 |
90 |
4.8 × 10−4 |
5 |
85 |
6 |
ORMOSIL-23 |
44 |
6.2 × 10−4 |
14 |
n.d. |
7 |
ORMOSIL-26 |
77 |
4.4 × 10−4 |
6 |
96 |
Interestingly, overall affinity of the nanoparticles for HSA results quite high since the Kd values obtained by the fitting are around 50 μM. Such result is remarkable since nanoparticles affinity for HSA is in the order of magnitude of vitamin D3. Nanoparticles conjugation hence not only compensates the small negative effect of the PEG spacer on albumin binding but also produced an affinity increase for the transport protein.
Conclusions
In summary, the results here reported shed an interesting light on the effect of PEG coating on the interaction of nanoparticles targeted with small molecules with biological receptors. Using HSA as model protein, we could demonstrate that not only the presence of the dense PEG layer does not prevent the recognition of the target, even a hydrophobic molecule as vitamin D3, from a suitable biological receptor. This suggests that previous low cellular uptake results obtained with nanoparticles targeted with different molecules, as folate or the RGD peptide, are not simply the result of targeting molecules masking produced by the PEG layer acting as an hydrophobic pseudophase. In these case, more subtle effects must be involved, which may include specific interactions of the targeting molecules with the coating layer or enhanced interaction with the protein present in the culture medium resulting into the sequestration of the ligands.
Indeed, another interesting featured revealed by this investigation is the possibility to enhance the affinity of albumin for vitamin D3 by grafting the latter to the nanoparticles. Albumin is highly concentrated in human serum (50 mg mL−1, 0.8 mM), consequently, nanoparticles injected in circulation may be expected to be completely bound to albumin. On the other hand, albumin is well known as potential tumour targeting agent since it binds to the CD60 receptor overexpressed by epithelial cells of many tumours. Vitamin D3 nanoparticles could hence be exploited as potential drug delivery agents that self-target after injection. Further studies are undergoing in our laboratories.
Experimental protocols
General
Detailed description of the starting material sources as well as the equipment and methods used is contained in the ESI section.†
Synthesis of vitamin D derivatives
3-O-(Vinyloxycarbonyl)vitamin D3 (8).
To a solution of vitamin D3 (500 mg, 1.30 mmol) in anhydrous CH2Cl2 (13 mL) at 0 °C, triethylamine (450 μL, 3.25 mmol) and vinyl chloroformate (dropwise, 300 μL, 3.25 mmol) were successively added. The reaction was stirred overnight. The crude was diluted with CH2Cl2 and filtered over Celite®. Solvents were evaporated under vacuum to afford 8 (92% yield), which was sufficiently pure for direct use in the next step. Rf: 0.9 (15% EtOAc/hexane); IR (NaCl): ν 2951, 2873, 1758, 1650 cm−1; 1H NMR (300.13 MHz, CDCl3): δ 0.55 (s, 3H, Me18), 0.87 (d, 6H, Me26 + Me27, J 6.6 Hz), 0.94 (d, 3H, Me21, J 6.0 Hz), 1.01–2.08 (several m, 21H), 2.22 (ddd, 1H, J 13.8, 9.4, 4.6 Hz), 2.45 (ddd, 2H, J 21.0, 9.7, 6.5 Hz), 2.66 (dd, 1H, J 13.4, 3.9 Hz), 2.81 (dd, 1H, J 11.8, 3.4 Hz), 4.57 (dd, 1H, H3′cis, J 6.2, 2.0 Hz), 4.87 (s, 1H, H19), 4.88 (m, 1H, H3), 4.91 (dd, 1H, H3′trans, J 13.9 + 2.0 Hz), 5.07 (s, 1H, H19), 6.03 (d, 1H, H6, J 11.3 Hz), 6.25 (d, 1H, H7, J 11.2 Hz), 7.10 (dd, 1H, H2′, J 13.8, 6.3 Hz) ppm; 13C NMR (75.5 MHz, CDCl3): δ 12.1 (Me18), 19.0 (Me21), 22.4 (CH2), 22.7 and 22.9 (Me26 + Me27), 23.7 (CH2), 24.0 (CH2), 27.8 (CH2), 28.1 (CH, C25), 29.2 (CH2), 31.9 (CH2), 32.0 (CH2), 36.21 (CH, C20), 36.22 (CH2), 39.6 (CH2), 40.7 (CH2), 42.0 (CH2), 46.0 (C13), 56.5 and 56.7 (2CH, C14 + C17), 76.5 (CH, C3), 97.6 (CH2, C3′), 113.2 (CH2, C19), 117.6 (CH, C7), 123.1 (CH, C6), 133.5 (C10), 142.7 (CH, C2′), 143.0 (C8), 144.2 (C5), 152.3 (C
O) ppm; MS (ESI+, m/z): 477 [(M + Na)+, 100%]; HRMS (ESI+, m/z): calcd for C30H47O3 [(M + H)+]: 455.3520, found: 455.3497.
3-O-(tert-Butyldimethylsilyl)-1-(vinyloxycarbonyloxy)vitamin D3 (15).
Same procedure as 8. The residue was purified by column chromatography (2% EtOAc/hexane as eluent) to give the derivative 15 in 86% yield. Rf: 0.5 (5% EtOAc/hexane); IR (KBr): ν 2951, 1762, 1642, 1469, 1379, 1259 cm−1; 1H NMR (300.13 MHz, CDCl3): δ 0.08 (s, 6H, SiMe), 0.50 (s, 3H, Me18), 0.85 (d, 6H, Me26 + Me27, J 6.6 Hz), 0.87 (s, 9H, SiCMe3), 0.91 (d, 3H, Me21, J 5.8 Hz), 1.00–2.83 (several m, 24H, 2H2 + 2H4 + 2H9 + 2H11 + 2H12 + H14 + 2H15 + 2H16 + H17 + H20 + 2H22 + 2H23 + 2H24 + H25), 4.15 (m, 1H, H3), 4.55 (dd, 1H, H3′, J 6.2 + 1.9 Hz), 4.89 (dd, 1H, H3′, J 13.9, 1.9 Hz), 5.08 (d, 1H, H19, J 1.8 Hz), 5.34 (dd, 1H, H1, J 5.2, 3.7 Hz), 5.38 (d, 1H, H19, J 1.2 Hz), 5.91 (d, 1H, H7, J 11.0 Hz), 6.33 (d, 1H, H6, J 11.3 Hz), 7.05 (dd, 1H, H2′, J 13.9, 6.2 Hz) ppm; 13C NMR (75.5 MHz, CDCl3): δ −4.6 (SiMe), −4.5 (SiMe), 12.1 (Me18), 18.2 (SiC), 19.0 (Me21), 22.3 (CH2), 22.7 and 23.0 (Me26 + Me27), 23.7 (CH2), 24.0 (CH2), 26.0 (SiCMe3), 27.8 (CH2), 28.2 (CH, C25), 29.2 (CH2), 29.9 (C), 36.3 (CH + CH2), 39.7 (CH2), 40.7 (CH2), 40.9 (CH2), 46.0 (C, C13), 46.1 (CH2), 56.5 (CH, C17), 56.7 (CH, C14), 67.2 (CH, C3), 78.9 (CH, C1), 97.7 (CH2, C3′), 116.4 (CH2, C19), 117.2 (CH, C7), 125.1 (CH, C6), 132.5 (C, C10), 141.9 (C, C8), 142.8 (CH, C2′), 143.2 (C, C5), 152.0 (C
O) ppm; MS (ESI+, m/z): 608 [(M + Na)+, 100%]; HRMS (ESI+, m/z): calcd for C36H61O4Si [(M + H)+]: 585.4339, found: 585.4344.
3-O-(tert-Butyldimethylsilyl)-1-[(4-methoxytetrahydro-2H-pyran-4-yl)oxy]vitamin D3 (16).
To a solution of 14 (100 mg, 0.19 mmol) in anhydrous CH2Cl2 (1.3 mL), pyridinium p-toluensulfonate (44 mg, 0.17 mmol) and 4-methoxy-5,6-dihydro-2H-pyran (dropwise, 65 μL, 0.58 mmol) were successively added and the reaction was stirred at room temperature for 4 h. Next, the mixture was extracted with CH2Cl2. The combined organic fractions were dried (Na2SO4) and concentrated under vacuum. The resulting crude was purified by flash chromatography (5% EtOAc/hexane) to afford 16 in 82% yield. Rf: 0.4 (5% EtOAc/hexane); IR (NaCl): ν 2954, 2865, 1470, 1376, 1359 cm−1; 1H NMR (300.13 MHz, CDCl3): 0.07 (s, 3H, SiMe), 0.08 (s, 3H, SiMe), 0.53 (s, 3H, Me18), 0.86 (2d, 6H, Me26 + Me27, J 6.6 Hz) 0.87 (s, 9H, SiCMe3), 0.91 (d, 3H, Me21, J 6.3 Hz), 1.00–2.85 (several m, 2H2 + 2H4 + 2H9 + 2H11 + 2H12 + H14 + 2H15 + 2H16 + H17 + H20 + 2H22 + 2H23 + 2H24 + H25 + 2H5′ + 2H2′), 3.21 (s, 3H, H6′), 3.65 and 3.71 (2m, 4H, H3′ + H4′), 4.21 (m, 1H, H3), 4.48 (m, 1H, H1), 4.93 (d, 1H, H19, J 2.3 Hz), 5.19 (d, 1H, H19, J 2.3 Hz), 6.02 (d, 1H, H7, J 11.4 Hz), 6.29 (d, 1H, H6, J 11.4 Hz) ppm; 13C NMR (75.5 MHz, CDCl3): δ −4.5 (SiMe), −4.4 (SiMe), 12.1 (Me18), 18.3 (SiC), 19.0 (Me21), 22.3 (CH2), 22.7 and 23.0 (Me26 + Me27), 23.7 (CH2), 24.1 (CH2), 26.0 (SiCMe3), 27.8 (CH2), 28.2 (CH, C25), 29.1 (CH2), 35.0 (CH2), 35.1 (CH2), 36.2 (CH2), 36.3 (CH), 39.6 (CH2), 40.7 (CH2), 43.9 (CH2), 46.0 (C, C13), 46.5 (CH2), 48.6 (CH3), 56.5 (CH, C17), 56.8 (CH, C14), 65.3 (CH2, C3′ + C4′), 67.5 (CH, C3), 70.5 (CH, C1), 99.0 (C, C1′), 113.1 (CH2, C19), 117.5 (CH, C7), 123.2 (CH, C6), 135.0 (C, C10), 142.1 (C, C8), 147.1 (C, C5) ppm; MS (ESI+, m/z): 630 [(M + H)+, 100%].
1α-[(4-Methoxytetrahydro-2H-pyran-4-yl)oxy]vitamin D3 (17).
TBAF (120 μL, 0.12 mmol, 1.0 M in THF) was added dropwise to a solution of silyl ether 16 (30 mg, 0.05 mmol) in anhydrous THF (480 μL) at 0 °C in darkness. The reaction mixture was followed by TLC (5% EtOAc/hexane) until complete consumption of starting material (4 h). Then, solvent was evaporated and the mixture was extracted with EtOAc. The combined organic fractions were dried (Na2SO4) and concentrated under vacuum. The crude was purified by column chromatography (20% EtOAc/hexane) to give 17 in 70% yield. Rf: 0.3 (30% EtOAc/hexane); IR (NaCl): ν 3269, 2951, 2867, 1467, 1351, 1301 cm−1; 1H NMR (300.13 MHz, CDCl3): δ 0.52 (s, 3H, Me18), 0.86 (2d, 6H, Me26 + Me27, J 6.1 Hz), 0.91 (d, 3H, Me21, J 6.2 Hz), 1.00–2.35 (several m, 27H, 2H2 + H4ax + H9ax + 2H11 + 2H12 + H14 + 2H15 + 2H16 + H17 + H20 + 2H22 + 2H23 + 2H24 + H25 + 2H5′ + 2H2′ + OH), 2.62 (dd, 1H, H4eq, J 11.5, 3.1 Hz), 2.82 (dd, 1H, H9eq, J 13.1, 3.8 Hz), 3.23 (s, 3H, H6′), 3.63 (m, 2H, H3′), 3.72 (m, 2H, H4′), 4.23 (m, 1H, H3), 4.51 (m, 1H, H1), 4.76 (s, 1H, OH), 4.97 (d, 1H, H19, J 2.3 Hz), 5.27 (d, 1H, H19, J 1.6 Hz), 6.02 (d, 1H, H7, J 11.4 Hz), 6.33 (d, 1H, H6, J 11.4 Hz) ppm; 13C NMR (75.5 MHz, CDCl3): δ −12.0 (Me18), 19.0 (Me21), 22.3 (CH2), 22.7 and 23.0 (Me26 + Me27), 23.7 (CH2), 24.0 (CH2), 27.8 (CH2), 28.2 (CH, C25), 29.1 (CH2), 35.0 (2CH2, C2′ + C5′), 36.2 (CH2), 36.3 (CH, C20), 39.6 (CH2), 40.7 (CH2), 43.1 (CH2), 45.8 (CH2), 46.0 (C, C13), 48.7 (CH3), 56.5 (CH, C17), 56.8 (CH, C14), 65.20 and 65.23 (CH2, C3′ and C4′), 67.2 (CH, C3), 69.6 (CH, C1), 99.1 (C, C1′), 112.8 (CH2, C19), 117.3 (CH, C7), 123.8 (CH, C6), 134.2 (C, C10), 142.9 (C, C8), 147.2 (C, C5) ppm; HRMS (ESI+, m/z): calcd for C33H54O4Na [(M + Na)+]: 537.3914, found: 537.3903.
1α-(4-Methoxytetrahydro-2H-pyran-4-yl)oxy-3-O-(vinyloxycarbonyl)vitamin D3 (18).
Same procedure as 8. The residue was purified by column chromatography (8% EtOAc/hexane as eluent) to give the derivative 18 in 83% yield. Rf: 0.3 (10% EtOAc/hexane); IR (NaCl): ν 2952, 2867, 2359, 23411657, 1467, 1351, 1260 cm−1; 1H NMR (300.13 MHz, CDCl3): δ 0.52 (s, 3H, Me18), 0.85 (d, 3H, Me26, J 6.6 Hz), 0.87 (d, 3H, Me27, J 6.1 Hz), 0.92 (d, 3H, Me21, J 6.2 Hz), 1.00–2.49 (several m, 27H), 2.73 (m, 2H, H4eq + H9eq), 3.23 (s, 3H, H6′′), 3.66 (m, 4H, H3′′ + H4′′), 4.52 (m, 1H, H1), 4.57 (dd, 1H, H3′, J 6.2, 2.0 Hz), 4.91 (dd, 1H, H3′, J 13.9, 2.0 Hz), 4.99 (d, 1H, H19, J 2.1 Hz), 5.18 (m, 1H, H3), 5.25 (d, 1H, H19, J 2.1 Hz), 6.02 (d, 1H, H7, J 11.4 Hz), 6.32 (d, 1H, H6, J 11.4 Hz), 7.09 (dd, 1H, H2′, J 13.9, 6.2 Hz) ppm; 13C NMR (75.5 MHz, CDCl3): δ 11.9 (Me18), 19.0 (Me21), 22.2 (CH2), 22.7, 23.0 (Me26 + Me27), 23.7 (CH2), 24.0 (CH2), 27.8 (CH2), 28.2 (CH, C25), 29.2 (CH2), 34.9 (CH2), 35.0 (CH2), 36.2 (CH, C20), 36.3 (CH2), 39.5 (CH2), 39.6 (CH2), 40.7 (CH2), 41.8 (CH2), 43.1 (CH2), 46.1 (C13), 48.7 (Me6′′), 56.5 and 56.8 (2CH, C14 + C17), 65.2 (2CH2, C3′′ + C4′′), 69.7 (CH, C1), 74.7 (CH, C3), 97.8 (CH2, C3′), 99.3 (C, C1′′), 113.8 (CH2, C19), 117.1 (CH, C7), 124.6 (CH, C6), 132.2 (C, C10), 142.7 (CH, C2′), 143.6 (C, C8), 146.0 (C, C5), 152.2 (C
O) ppm; HRMS (ESI+, m/z): calcd for C36H56O6Na [(M + Na)+]: 607.3969, found: 607.3956.
3-O-(Vinyloxy)carbonyl-25-ethoxymethyloxyvitamin D3 (23).
Same procedure as 8. The residue was purified by column chromatography (10% Et2O/hexane as eluent) to give the derivative 23 in 92% yield. Rf: 0.5 (10% EtOAc/hexane); IR (NaCl): ν 2946, 2873, 1758, 1650, 1469 cm−1; 1H NMR (300.13 MHz, CDCl3): δ 0.54 (s, 3H, Me18), 0.93 (d, 3H, Me21, J 6.2 Hz), 1.19 (t, 3H, Me3′′, J 6.9 Hz), 1.21 (s, 6H, Me26 + Me27), 0.98–2.50 (several m, 2H2 + H4ax + H1ax + 2H9 + 2H11 + 2H12 + H14 + 2H15 + 2H16 + H17 + H20 + 2H22 + 2H23 + 2H24), 2.66 (1H, dd, H4eq, J 13.4, 3.9 Hz), 2.80 (dd, 1H, H1eq, J 11.6, 3.2 Hz), 3.61 (q, 2H, H2′′, J 7.1 Hz), 4.56 (dd, 1H, H3′′cis, J 6.2, 2.0 Hz), 4.75 (s, 2H, H1′′), 4.86 (d, 1H, H19, J 2.0 Hz), 4.88 (m, 1H, H3), 4.90 (dd, 1H, H3′trans,J 13.9, 2.0 Hz), 5.07 (d, 1H, H19, J 2.0 Hz), 6.02 (d, 1H, H7, J 11.3 Hz), 6.24 (d, 1H, H6, J 11.4 Hz), 7.10 (dd, 1H, H2′, J 13.8, 6.3 Hz) ppm; 13C NMR (75.5 MHz, CDCl3): δ 12.1 (Me18), 15.3 (Me3′′), 19.0 (Me21), 20.7 (CH2), 22.4 (CH2), 23.7 (CH2), 26.5 and 26.6 (Me26 + Me27), 27.8 (CH2), 29.2 (CH2), 31.9 (CH2), 32.0 (CH2), 36.3 (CH, C20), 36.6 (CH2), 40.7 (CH2, C12), 42.0 (CH2), 42.4 (CH2), 46.1 (C, C13), 56.5 and 56.8 (2CH, C17 + C14), 63.1 (CH2, C2′′), 76.3 (C, C25), 76.7 (CH, C3), 89.7 (CH2, C1′′), 97.7 (CH2, C3′), 113.2 (CH2, C19), 117.6 (CH, C7), 123.1 (CH, C6), 133.5 (C, C10), 142.8 (CH, C2′), 143.0 (C, C8), 144.2 (C5), 152.3 (C
O) ppm; MS (ESI+, m/z): 551 [(M + Na)+, 100%]; HRMS (ESI+, m/z): calcd for C33H52NaO5 (M + Na)+: 551.3707, found: 551.3710.
3-O-(tert-Butyldimethylsilyl)-25-(vinyloxycarbonyloxy)vitamin D3 (26).
Same procedure as 8. The residue was purified by column chromatography (4% EtOAc/hexane as eluent) to give the derivative 26 in 73% yield; Rf: 0.5 (5% EtOAc/hexane); 1H NMR (300.13 MHz, CDCl3): δ 0.07 (s, 6H, SiMe), 0.54 (s, 3H, Me18), 0.89 (s, 9H, SiCMe3), 0.92 (d, 3H, Me21, J 6.4 Hz), 1.49 (s, 6H, Me26 + Me27), 0.98–2.50 (several m, 2H2 + 2H4 + H1ax + 2H9 + 2H11 + 2H12 + H14 + 2H15 + 2H1 + H17 + H20 + 2H22 + 2H23 + 2H24), 2.83 (d, 1H, H1eq, J 11.7 Hz), 3.82 (m, 1H, H3), 4.51 (d, H3′, 1H, J 6.0 Hz) 4.78 (s, 1H, H19), 4.87 (d, 1H, H3′, J 13.9 Hz), 5.00 (s, 1H, H19), 6.01 (d, 2H, H7, J 10.5 Hz) 6.16 (d, 2H, H6, J 10.9 Hz), 7.06 (dd, 1H, H2′, J 13.6, 5.9 Hz) ppm; 13C NMR (75.5 MHz, CDCl3): δ −4.5 (SiMe), −4.4 (SiMe), 12.3 (Me18), 18.3 (SiC), 18.9 (Me21), 20.6 (CH2), 22.4 (CH2), 23.6 (CH2), 25.8 (Me26 + Me27), 26.1 (SiCMe3), 27.9 (CH2), 29.1 (CH2), 29.9 (CH2), 32.9 (CH2, C1), 36.2 (CH, C20), 36.3 (CH2), 36.6 (CH2), 40.7 (CH2, C12), 41.0 (CH2), 45.9 (C, C13), 47.1 (CH2), 56.5 and 56.7 (2CH, C14 + C17), 70.7 (CH, C3), 86.0 (C, C25), 97.1 (CH2, C3′), 112.3 (CH2, C19), 118.0 (CH, C7), 121.5 (CH, C6), 136.5 (C, C10), 141.6 (C, C8), 142.7 (CH, C2′), 145.6 (C, C5), 150.9 (C
O) ppm; MS (APCI+, m/z): 585 [(M + H)+, 50%], 497 [(M − vinyl carbonate)+, 100%], 365 [(M − vinyl carbonate − OTBDMS)+, 55%]; HRMS (ESI+, m/z): calcd for C30H60O4Si [(M + H)+]: 585.4260, found: 585.4246.
3-O-[N-(8-Methoxy-3,6-dioxaoctyl)carbamoyl]vitamin D3 (28).
A solution of vinyl carbonate 8 (57 mg, 0.12 mmol) in anhydrous THF (1.7 mL) was treated with 51 mg (0.31 mmol) of 2-[2-(2-methoxyethoxy)ethoxy]ethylamine and the mixture was stirred at 50 °C until TLC showed complete consumption of starting material (48 h). Then, solvent was evaporated and the residue purified by column chromatography (eluent 40% EtOAc/hexane) to afford 28 in 73% yield. Rf: 0.4 (60% EtOAc/hexane); 1H NMR (250.13 MHz, CDCl3): δ 0.53 (s, 3H, Me18), 0.85 (d, 6H, Me26 + Me27, J 6.8 Hz), 0.90 (d, 3H, Me21, J 6.7 Hz), 0.80–3.00 (several m), 3.37–3.62 (several m, 15H, H2′–8′), 4.82 (s, 1H, H19), 4.88 (m, 1H, H3), 5.03 (s, 1H, H19), 5.42 (br s, 1H, NH), 6.02 (d, 1H, H7, J 12.4 Hz), 6.21 (d, 2H, H6, J 12.0 Hz) ppm; 13C NMR (75.5 MHz, CDCl3): δ 12.1 (Me18), 19.0 (Me21), 22.4 (CH2), 22.7 and 22.9 (Me26 + Me27), 23.7 (CH2), 24.0 (CH2), 27.8 (CH2), 28.1 (CH, C25), 29.2 (CH2), 32.3 (CH2), 32.4 (CH2), 36.3 (CH2 + CH, C20), 39.7 (CH2), 40.7 (CH2), 40.9 (CH2), 42.7 (CH2), 46.0 (C, C13), 56.5 and 56.7 (2CH, C17 + C14), 59.2 (CH3, C8′), 70.2 (CH2), 70.4 (CH2), 70.6 (CH2), 72.0 (CH, C3), 72.7 (CH2), 112.6 (CH2, C19), 117.6 (CH, C7), 122.4 (CH, C6), 134.8 (C10), 142.3 (C8), 145.0 (C5), 156.2 (C
O) ppm.
Acknowledgements
Financial support by the Spanish Ministerio de Ciencia e Innovación (MICINN) (Projects CTQ2011-24237 and CTQ2014-55015), Principado de Asturias (Project FC-15-GRUPIN14-002), Italian Ministry of Research (Project FIRB 2011 RBAP114AMK-RINAME) and University of Padova (Progetto Strategico NAMECA) are gratefully acknowledged. T. G.-G. thanks FICYT (Asturias) for a pre-doctoral fellowship.
References
-
(a) M. W. Ambrogio, C. R. Thomas, Y.-L. Zhao, J. I. Zink and J. F. Stoddartt, Acc. Chem. Res., 2011, 44, 903–913 CrossRef CAS PubMed
;
(b) S.-H. Wu, Y. Hung and C.-Y. Mou, Chem. Commun., 2011, 9972–9985 RSC
;
(c) P. Couleaud, V. Morosini, C. Frochot, S. Richeter, L. Raehm and J.-O. Durand, Nanoscale, 2010, 2, 1083–1095 RSC
;
(d) J. L. Vivero-Escoto, I. I. Slowing, B. G. Trewyn and V. S.-Y. Lin, Small, 2010, 6, 1952–1967 CrossRef CAS PubMed
;
(e) W. J. M. Mulder, G. J. Strijkers, G. A. F. Van Tilborg, D. P. Cormode, Z. A. Fayad and K. Nicolay, Acc. Chem. Res., 2009, 42, 904–914 CrossRef CAS PubMed
;
(f) Y. Piao, A. Burns, J. Kim, U. Wiesner and T. Hyeon, Adv. Funct. Mater., 2008, 18, 3745–3758 CrossRef CAS
.
-
Colloidal Silica: Fundamentals and Applications, ed. H. E. Bergna and W. O. Roberts, CRC Press, Boca Raton, 2006 Search PubMed
.
-
(a) A. Burns, P. Sengupta, T. Zedayko, B. Baird and U. Wiesner, Small, 2006, 2, 723–726 CrossRef CAS PubMed
;
(b) A. Vanblaaderen and A. Vrij, Langmuir, 1992, 8, 2921–2931 CrossRef CAS
.
- B. G. Trewyn, I. I. Slowing, S. Giri, H.-T. Chen and V. S.-Y. Lin, Acc. Chem. Res., 2007, 40, 846–853 CrossRef CAS PubMed
.
-
(a) J. Hu, M. Chen, X. Fang and L. Wu, Chem. Soc. Rev., 2011, 40, 5472–5491 RSC
;
(b) X. Du and J. He, Nanoscale, 2011, 3, 3984–4002 RSC
;
(c) L. Bau, B. Bartova, M. Arduini and F. Mancin, Chem. Commun., 2009, 7584–7586 RSC
.
- M. Arduini, F. Mancin, P. Tecilla and U. Tonellato, Langmuir, 2007, 23, 8632–8636 CrossRef CAS PubMed
.
- L. Bau, P. Tecilla and F. Mancin, Nanoscale, 2011, 3, 121–133 RSC
.
- I. M. Rio-Echevarria, F. Selvestrel, D. Segat, G. Guarino, R. Tavano, V. Causin, E. Reddi, E. Papini and F. Mancin, J. Mater. Chem., 2010, 20, 2780–2787 RSC
.
- R. Ciriminna, M. Sciortino, G. Alonzo, A. de Schrijver and M. Pagliaro, Chem. Rev., 2011, 111, 765–789 CrossRef CAS PubMed
.
-
(a) M. Gary-Bobo, Y. Mir, C. Rouxel, D. Brevet, I. Basile, M. Maynadier, O. Vaillant, O. Mongin, M. Blanchard-Desce, A. Morere, M. Garcia, J. O. Durand and L. Raehm, Angew. Chem., Int. Ed., 2011, 50, 11425–11429 CrossRef CAS PubMed
;
(b) S. Kim, T. Y. Ohulchanskyy, D. Bharali, Y. Chen, R. K. Pandey and P. N. Prasad, J. Phys. Chem. C, 2009, 113, 12641–12644 CrossRef CAS PubMed
;
(c) S. Kim, T. Y. Ohulchanskyy, H. E. Pudavar, R. K. Pandey and P. N. Prasad, J. Am. Chem. Soc., 2007, 129, 2669–2675 CrossRef CAS PubMed
;
(d) T. Y. Hulchanskyy, I. Roy, L. N. Goswami, Y. Chen, E. J. Bergey, R. K. Pandey, A. R. Oseroff and P. N. Prasad, Nano Lett., 2007, 7, 2835–2842 CrossRef PubMed
;
(e) I. Roy, T. Y. Ohulchanskyy, H. E. Pudavar, E. J. Bergey, A. R. Oseroff, J. Morgan, T. J. Dougherty and P. N. Prasad, J. Am. Chem. Soc., 2003, 125, 7860–7865 CrossRef CAS PubMed
.
-
(a) R. Kumar, I. Roy, T. Y. Hulchanskyy, L. N. Goswami, A. C. Bonoiu, E. J. Bergey, K. M. Tramposch, A. Maitra and P. N. Prasad, ACS Nano, 2008, 2, 449–456 CrossRef CAS PubMed
;
(b) W.-C. Law, K.-T. Yong, I. Roy, G. Xu, H. Ding, E. J. Bergey, H. Zeng and P. N. Prasad, J. Phys. Chem. C, 2008, 112, 7972–7977 CrossRef CAS
;
(c) S. Kim, H. E. Pudavar and P. N. Prasad, Chem. Commun., 2006, 2071–2073 RSC
;
(d) D. J. Bharali, I. Klejbor, E. K. Stachowiak, P. Dutta, I. Roy, N. Kaur, E. J. Bergey, P. N. Prasad and M. K. Stachowiak, Proc. Natl. Acad. Sci. U. S. A., 2005, 102, 11539–11544 CrossRef CAS PubMed
;
(e) I. Roy, T. Y. Ohulchanskyy, D. J. Bharali, H. E. Pudavar, R. A. Mistretta, N. Kaur and P. N. Prasad, Proc. Natl. Acad. Sci. U. S. A., 2005, 102, 279–284 CrossRef CAS PubMed
.
- F. Selvestrel, F. Moret, C. Fedeli, J. H. Woodhams, S. Zanini, I. Rio Echevarria, L. Baù, F. Rastrelli, C. Compagnin, E. Reddi, R. Tavano, D. Segat, E. Papini, A. Mackenzie, M. Bovis, E. Yaghini, A. MacRobert, G. Fracasso, A. Boscaini, M. Colombatti and F. Mancin, Nanoscale, 2013, 5, 6106–6116 RSC
.
- D. Segat, R. Tavano, M. Donini, F. Selvestrel, I. Rio-Echevarria, M. Rojnik, P. Kocbek, J. Kos, S. Iratni, D. Scheglmann, F. Mancin, S. Dusi and E. Papini, Nanomedicine, 2011, 6, 1027–1046 CrossRef CAS PubMed
.
- R. Tavano, D. Segat, E. Reddi, J. Kos, M. Rojnik, P. Kocbek, S. Iratni, D. Scheglmann, M. Colucci, I. M. Rio Echevarria, F. Selvestrel, F. Mancin and E. Papini, Nanomedicine, 2010, 5, 881–896 CrossRef CAS PubMed
.
-
(a)
Vitamin D, ed. D. Feldman, J. W. Pike and J. S. Adams, Elsevier Academic Press, San Diego, CA, 3rd edn, 2011 Search PubMed
;
(b)
Vitamin D, ed. D. Feldman, J. W. Pike and F. H. Glorieux, Elsevier Academic Press, Burlington, MA, 2nd edn, 2005 Search PubMed
.
-
(a) M. T. Cantorna, Proc. Nutr. Soc., 2010, 69, 286–289 CrossRef CAS PubMed
;
(b) G. Jones, S. A. Strugnell and H. F. DeLuca, Physiol. Rev., 1998, 78, 1193–1231 CAS
;
(c)
H. F. DeLuca, J. Burmester, H. Darwish and J. Krisinger, Molecular mechanism of the action of 1,25-dihydroxyvitamin D3, in Comprehensive Medicinal Chemistry, ed. C. Hansch, P. G. Sammes and J. B. Taylor, Pergamon Press, Oxford, 1991, vol. 3, pp. 1129–1143 Search PubMed
.
- D. D. Bikle, P. K. Siiteri, E. Ryzen and J. G. Haddad, J. Clin. Endocrinol. Metab., 1985, 61, 969–975 CrossRef CAS PubMed
.
-
(a) S. Yamada, T. Suzuki and H. Takayama, J. Org. Chem., 1983, 48, 3483–3488 CrossRef CAS
;
(b) S. Yamada and H. Takayama, Chem. Lett., 1979, 8, 583–586 CrossRef
;
(c) W. Reischl and E. Zbiral, Helv. Chim. Acta, 1979, 62, 1763–1769 CrossRef CAS
.
- J. A. Marshall, J. Grote and B. Shearer, J. Org. Chem., 1986, 51, 1635–1637 CrossRef
.
- B. Lythgoe, T. A. Moran, M. E. N. Nambudhy, S. Ruston, J. Tideswell and P. W. Wright, Tetrahedron Lett., 1975, 16, 3863–3866 CrossRef
.
- H. T. Toh and W. H. Okamura, J. Org. Chem., 1983, 48, 1414–1417 CrossRef CAS
.
- L. Sánchez-Abella, S. Fernández, A. Verstuyf, L. Verlinden, V. Gotor and M. Ferrero, J. Med. Chem., 2009, 52, 6158–6162 CrossRef PubMed
.
- A. Hernández-Martín, T. González-García, M. Lawlor, L. Preston, V. Gotor, S. Fernández and M. Ferrero, Bioorg. Med. Chem., 2013, 21, 7779–7789 CrossRef PubMed
.
-
(a) R. Marega, V. Aroulmoji, F. Dinon, L. Vaccari, S. Giordani, A. Bianco, E. Murano and M. Prato, J. Am. Chem. Soc., 2009, 131, 9086–9093 CrossRef CAS PubMed
;
(b) A. D. Chen and M. J. Shapiro, Anal. Chem., 1999, 71, 669A–675A CrossRef CAS PubMed
.
Footnote |
† Electronic supplementary information (ESI) available: Experimental procedures; analytical data; figures giving 1H, 13C, and DEPT NMR spectral data of vitamin D3 analogues and intermediates; 1H NMR spectra of nanoparticles; UV spectra of vitamin D3 analogues, nanoparticles, and conjugates; fluorescence spectra of nanoparticles; SPR sensorgrams; affinity curves of vitamin D3-HSA and 28-HSA. See DOI: 10.1039/c6ra02965e |
|
This journal is © The Royal Society of Chemistry 2016 |
Click here to see how this site uses Cookies. View our privacy policy here.