DOI:
10.1039/C6RA02397E
(Paper)
RSC Adv., 2016,
6, 38117-38123
Immobilization of horseradish peroxidase enzymes on hydrous-titanium and application for phenol removal
Received
26th January 2016
, Accepted 28th March 2016
First published on 1st April 2016
Abstract
In this work, hydrous titanium was utilized to immobilize horseradish peroxidase (HRP) in order to improve its stability and adaptability under different water qualities using a biomimetic titanification process. The catalytic performance of immobilized HRP for phenol removal from aqueous solution was evaluated. The physicochemical properties of immobilized HRP (IM-HRP) were characterized by means of scanning electron microscopy (SEM), transmission electron microscopy (TEM), and Fourier transform infrared spectroscopy (FT-IR). The effects of the reaction conditions (H2O2 and IM-HRP dosages and initial phenol concentration) on phenol removal were investigated. Enzymatic activity analysis indicated that the immobilization process has no adverse effects on the catalytic performance of HRP, and a slight increase in the enzymatic catalytic kinetic constant (km) of IM-HRP was observed compared to its free enzymes. It was found that at a synthetic temperature of 37 °C ± 3 °C, IM-HRP exhibited excellent phenol removal of over 90% after reaction for 15 min. In addition, the enzyme activity of IM-HRP was very stable over a wide range of reaction conditions (e.g., temperatures from 20 °C to 90 °C and pH from 3.0 to 11.0). Therefore, HRP enzymes loaded on hydrous titanium are very effective at improving the stability of enzymes and can alleviate the adverse effects of various environmental and water conditions on contaminant removal.
1. Introduction
Phenolic compounds are considered as environment threats and are usually discharged from industrial processes such as coal conversion, dyes production, plastic processing and so on.1 Most phenolic compounds in water are toxic and may pose a great threat to the environment and human health. Many conventional methods have been developed and applied to remove/degrade phenolic compounds in the environment, such as adsorption by carbon or membranes, chemical oxidation, biological degradation, solvent extraction and coagulation.2,3 With the advent of green technology, the use of enzymes as biocatalysts has attracted considerable attention and become increasingly popular. Enzyme-catalytic degradation techniques provide a novel solution for the abatement of pollutants in the environment.4–6 As effective biocatalysts, enzymes have many advantages, including substrate specificity, high catalytic efficiency and mild reaction conditions; furthermore, they can be utilized and degraded by bacteria. Horseradish peroxidase (HRP), which is the peroxidase extracted from plants, is a glycosylated enzyme containing one heme ion Fe-protoporphyrin (IX) prosthetic group and two Ca(II) ions; the combination of the two types of metal ions produces the active center.7,8 HRP has been widely used in organic synthesis, polymeric synthesis and the treatment of wastewater containing phenolic compounds.6,9 The catalytic mechanism for the degradation of phenolic compounds by HRP is thought to be the formation of phenoxy radicals in the presence of hydrogen peroxide.10
However, the free HRP enzymes are subject to the reaction conditions and solution chemistry and show poor stability in wastewater treatment. In particular, their structure might change and lose catalytic function under adverse conditions. Hence, the full-scale application of enzyme-related environmental remediation strategies has been limited. In order to solve this problem,11,12 a promising method is immobilization, in which the enzymes are loaded on suitable carriers,13–16 producing the following advantages: ease of recycling the enzymes from the reaction mixture, abatement of secondary pollution, improved stability and broadened adaptability of enzymes.17,18 For example, HRP enzymes were encapsulated into silica glass using a sol–gel process to analyze phenolic compounds.19 Similarly, HRP enzymes were immobilized on magnetic core–shell particles (silica-coated Fe3O4 particles modified by NH2) for the removal of 2,4-dichlorophenol from aqueous solutions.20 The results showed that the immobilized HRP was more stable than free HRP. Grapheme oxide/clays and the soil organic matter were used as matrices for immobilizing HRP enzymes, the enzymes could be successfully immobilized on carriers without the use of any additional surface modification or cross-linking reagents.21,22 In comparison to these materials, titanium-based materials are also attractive in a number of fields.23,24 For instance, the titanium sol–gel process can be controlled at near-neutral pH and ambient temperature under mild conditions within a short process period.21–24 Jiang et al. found that HRP enzymes encapsulated in phospholipid-templated titanium with Ti-BALDH as the titanium source were efficient at removing phenolic compounds and dyes.24 Although immobilized enzymes have many advantages over free enzymes, the long aging time during the immobilization process can denature the structures of the enzymes and reduce the enzyme activity. Therefore, the synthetic process should be carefully controlled.
In this paper, HRP enzymes were immobilized on hydrous-titanium coatings through a biomimetic titanification process. The immobilized enzymes were then used to remove phenolic compounds from aqueous solutions. To generate the 3-D open mesoporous structure of the biocatalyst and eliminate the special response of the enzyme to the hydrous titanium forming process, lecithins were selected as the template to protect the enzymes from severe conditions. First, the IM-HRP particles were characterized by FT-IR, TEM, and SEM. The effects of pH and temperature on the activity of the free/immobilized residual activities of the HRP enzymes were evaluated. In addition, the effects of the reaction conditions (time, H2O2, IM-HRP dosage and initial concentration) on phenol removal in the presence of IM-HRP and free HRP were compared.
2. Materials and methods
2.1 Materials
HRP (RZ = 3.0, enzymatic activity ≥ 250 U mg−1) was purchased from Shanghai Roche pharmaceutical Ltd (Shanghai, China). Soybean lecithin (10 g L−1 at 25 °C), solid phenol (analytically pure; content ≥ 99.0%), 4-aminoantipyrine (chemically pure; content ≥ 98.5%), potassium ferricyanide (analytically pure; density ≥ 99.5%), TritonX-100(chemical pure; density ≥ 0.980 at 20 °C), α-lactose monohydrate (analytically pure), anhydrous ethanol (analytically pure; content ≥ 99.7%), and tetrabutyl titanate (chemical pure; content ≥ 98.0%) were purchased from national medicine group Shanghai Chemical Reagent co. (Shanghai, China).
2.2 Immobilization of HRP on hydrous titanium
In the process of preparing hydrous titanium particles as the enzyme carriers, the lecithins were dissolved in absolute ethyl alcohol and formed mixed micelles as the biomimetic templates. The lecithins protected the enzymes from the unfriendly environments24 during the formation of the hydrous titanium particles. The lactose acted as the structure-preserving lyoprotectant, prohibiting enzyme denaturation.25
The immobilized HRP on hydrous titanium process was described in sentences as follows: A first solution of HRP (10 mg) in 2 mL phosphate (pH 7.0) containing 0.05 g lactose was prepared at 25 °C. It was slowly added under mechanical stirring to a second solution, which was prepared by dissolving 0.3 g lecithin with absolute ethyl alcohol (5 mL) under ultrasonication for 15 min. The entire mixture was stirred vigorously for 0.5 h to form soft phospholipid microspheres of HRP enzymes as stock solution A.
The above mixture (solution A) was then added slowly to 3 mL of tetrabutyl titanate (1.76 mol L−1, pH 7.0), which was prepared from tetrabutyl titanate and absolute ethyl alcohol at 25 °C under mechanical stirring (1 h). After titania particles were formed and aged for 15 min, the final particles were washed twice with TritonX-100 (7.5%) and ultra-pure water to remove the lecithins and then dried under vacuum (37 °C).
2.3 Characterization of IM-HRP
2.3.1 Transmission electron microscopy (TEM) and Fourier transform infrared spectroscopy (FT-IR). The IM-HRP samples were ultrasonically treated with absolute ethyl alcohol for 0.5 h and dried on a copper grid at room temperature for determination. The TEM analyses of the IM-HRP samples were carried out using an transmission electron microscope (H-7500, Hitachi, Japan) with an accelerating voltage of 80 kV and a magnification of 1.5 × 105. FT-IR spectra of the IM-HRP samples were measured using a Nexus 670 normal-position analysis FT-IR spectrophotometer (Thermo Fisher, USA). The FT-IR spectra were recorded in the range of 4000 to 400 cm−1.
2.3.2 Scanning electron microscopy (SEM). The IM-HRP samples were dried and coated with gold prior to analysis. A scanning electron microscope (JSM35-CF) was applied to observe the surfaces of the IM-HRP samples under an accelerating voltage of 5.0 kV.
2.4 Measurement of enzyme activity
The enzyme activity of HRP was measured according to the method described by J. Cheng et al.1 One unit enzyme activity (U) was defined as the amount of enzyme required to resolve 1 μmol hydrogen peroxide at pH 7.0 each minute at 25 °C; the relative enzyme activity could be described using the following equation:
The maximum enzyme activity was regarded as the free/immobilized HRP enzymes activity in optimum conditions to be studied respectively.
The reagents required to determine the enzyme activity were a 1.4 mL solution of phenol (0.1723 mol L−1) and 4-APP (2.460 × 10−3 mol L−1), 1.5 mL H2O2 (2.12 μmol L−1) and 0.1 mL 0.05–0.25 U mL−1 enzyme solution.1 The generation rate of the red non-precipitating products was proportional to the enzyme activity. 4-APP was acted as a hydrogen donor to the reaction of enzymatic catalysis of the phenolic substances in the presence H2O2. The relative concentration of red non-precipitating products was determined by the absorbance at 510 nm (25 °C). The coloration reaction rate was measured using a Purkinje TU-1901 UV-visible spectrophotometer (resolution = 1 nm) in the wavelength range from 190 to 900 nm.
2.5 Phenol removal test
In order to determine the reaction time required for efficient phenol removal, comparative experiments were carried out for free/immobilized HRP to remove phenol from aqueous solutions. All experiments were performed in 100 mL conical flasks under vigorous agitation. The effects of the temperature and pH on the residual activity of free/immobilized HRP were tested by incubating enzymes in buffer solutions at pH 7.0 at temperatures ranging from 20 °C to 90 °C and in buffer solutions at 25 °C within the pH range of 3.0 to 11.0 for 2 h. IN addition, the influences of reaction conditions such as catalysis reaction time, H2O2 amount, immobilized HRP dosage and initial phenol concentration on phenol removal efficiency were investigated.
The concentrations of phenol in the assay solutions were measured using a previously reported method.22,26 In brief, the phenol concentration was quantified by measuring the absorbance at 510 nm of the red compounds generated in the presence of 0.20 mL potassium ferricyanide (83.4 mmol L−1 K3Fe(CN)6 in 0.25 mol L−1 NaHCO3) and 0.2 mL 4-APP (20.8 mmol L−1 4-anminoantypyrine in 0.25 mol L−1 NaHCO3).
3. Results and discussion
3.1 Immobilization of HRP on hydrous titanium
On account of the poor stability of free HRP enzymes and the second pollution of the treated water since the free HRP enzymes cannot be separated after treatment, we presented a method for HRP enzymes immobilization (Scheme 1). With the protection of HRP enzymes by lecithin microspheres, hydrous titanium formed around the lecithin microspheres as a template for tetrabutyl titanate, and the lecithins were removed by TritonX-100. The results showed that the stability of IM-HRP enzymes was enhanced under harsh conditions, and the enzymes could be easily separated.
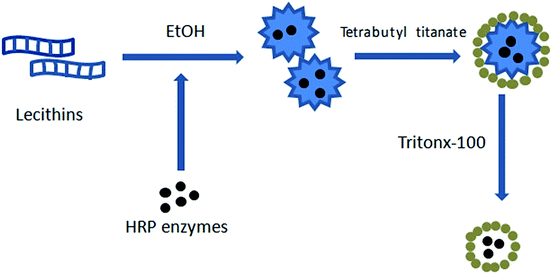 |
| Scheme 1 Immobilization of HRP enzymes. | |
3.2 Characterization of IM-HRP
3.2.1 TEM and SEM characterization. TEM analysis was used to determine the morphological changes in the immobilized HRP enzymes. Fig. 1(A) shows a TEM image of IM-HRP enzymes; the enzymes were clearly wrapped by a layer of hydrated titanium. Fig. 1(B) shows an SEM image of the encapsulated HRP, which presented the images of samples were rules together of large microspheres. As illustrated in Fig. 1(C), the samples were aggregates of microspheres. Since the aggregates had large specific surface areas, this morphology was advantageous to biocatalyst reaction process. This result was in agreement with the properties of biomimetic silica particles reported by Luckarift.17 In addition, well-encapsulated HRP can be clearly observed in the TEM images; the enzymes were wrapped in layers of hydrated titanium, which looked like the TiO2-shell coatings on Fe3O4-core nanoparticles reported by A. Hasanpour et al.26
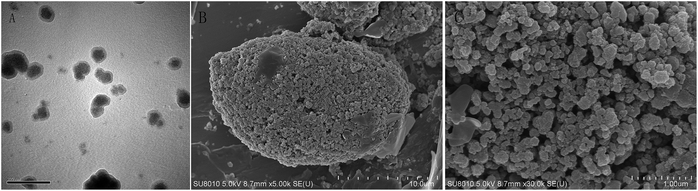 |
| Fig. 1 (A) TEM of immobilized HRP (acceleration voltage = 80 KV, magnification = 1.5 × 105); (B) SEM image of entire unit of immobilized-HRP; and (C) SEM image of the surface of IM-HRP. | |
3.2.2 FT-IR characterization. The FT-IR spectra of free-HRP/hydrous-titania/immobilized-HRP bead surfaces are presented in Fig. 2. For the free-HRP, the characteristic transmittances at 1510 cm−1 and 1244 cm−1 were related to the stretching brand of –CONH– (amide I) and (amide II) vibrations.9 The sharp transmittances of the bands at 1631 cm−1 and 1047 cm−1 were associated with the stretching vibrations of Ti–H bonds, which are features of hydrated TiO2 particles surfaces. The band at 2969 cm−1 represented the stretching of –NH2 groups. On the other hand, after the HRP enzymes were immobilized by hydrated TiO2 coatings, the immobilized HRP particles still exhibited three characteristic stretching bands at 1510 cm−1, 1631 cm−1 and 2969 cm−1, corresponding to –CONH–, Ti–H and –NH2 vibrations, respectively. These results demonstrated that the enzymes were immobilized on hydrated titanium. The SEM, TEM, and FT-IR results all indicate that free HRP enzymes were successfully immobilized on hydrated titanium coatings.
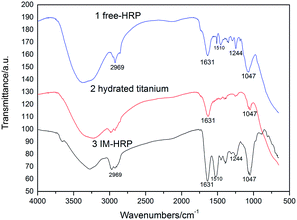 |
| Fig. 2 FT-IR spectra of free HRP, hydrated titanium and IM-HRP. | |
3.3 Effects of reaction conditions on enzyme residual activity in different enzyme-catalyzed systems
3.3.1 Effect of temperature on the residual activity of enzymes. Temperature is thought to be one of the most important influencing factors in enzyme activity. Enzyme structure might be destroyed at certain temperatures, resulting in function loss. Therefore, it is necessary to determine the effects of temperature on the activity of free/immobilized HRP enzyme. In this work, the effects of temperature on the residual activity of the free/immobilized HRP enzymes were investigated. The results are shown in Fig. 3; the optimal temperature for the free/immobilized HRP enzymes was about 37 °C. In order to enable the free/immobilized HRP enzymes to function efficiently in the catalysis reaction process, the temperature of the catalytic reaction should be controlled to 30–40 °C. The results showed that free HRP enzymes almost lost activity at temperatures above 70 °C. However, IM-HRP enzymes exhibited significantly higher stability than the free HRP enzymes in the examined temperature range. The IM-HRP enzymes exhibited 50% residual enzyme activity under the optimal conditions in the temperature range of 60–90 °C. These results might indicate that the IM-HRP enzymes were more resistant to the high-temperature conditions than free HRP enzyme. This phenomenon might be due to the improved stability of HRP enzymes resulting from immobilizing the free HRP enzymes on hydrated titanium coatings.
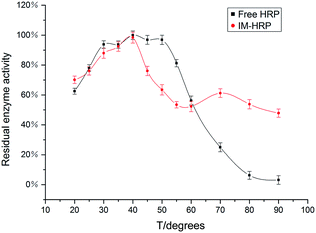 |
| Fig. 3 Residual enzyme activity of initial enzyme at different temperatures; 5 mg of IM-HRP, content of HRP on carrier = 3.11 mg g−1; 0.1 mL free HRP (20 mg L−1). | |
3.3.2 Effect of pH on residual enzyme activity. As depicted in Fig. 4, the optimal pH for both free and immobilized HRP enzymes was about 7.0. At this pH, the residual enzyme activities of IM-HRP and free-HRP were 83.3% and 73.1% of the initial value, respectively. Moreover, the residual activity of immobilized HRP enzymes was more stable under harsh pH conditions (acid and alkaline) compared to that of the free HRP enzymes. These results revealed that the IM-HRP enzymes were more resistant to changes in pH. It was very likely that the stability against changes in pH of the HRP enzymes was improved by loading the enzymes on the surfaces of hydrated titanium.
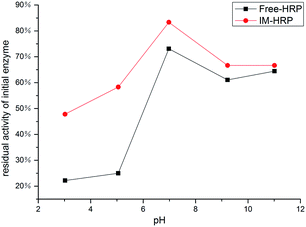 |
| Fig. 4 Residual enzyme activity of the initial enzyme after storage for 4 h at different pH values (temperature = 25 °C). | |
3.4 Catalytic reaction kinetics of free HRP and IM-HRP
In order to understand the enzymatic capacity of free/immobilized HRP enzymes, the enzyme constants km (Michaelis constant) were obtained using phenol compounds as the substrate. For the enzyme-catalyzed process, the reaction rate (v) and the substrate concentration of phenol ([S]) compounds conformed to the Michaelis–Menten equation. The km could be obtained by following equation:
The km of free/immobilized HRP enzymes were obtained by 1/[S] to 1/v ([S] was as the substrate concentration of phenol; [v] was as the reaction rate of the coloration formation).
As shown in Fig. 5, the experimental data conformed well to the Michaelis–Menten equation (R2 > 0.98). The constant km of IM-HRP for phenol degradation was slighter higher than that of free-HRP enzymes in the presence of H2O2. It was demonstrated that the catalytic performance was comparable to that of the free-HRP enzymes. Moreover, the increase in the km value of IM-HRP could be explained by the result of the reduction in enzyme activity after HRP was immobilized,29 or lower rate capacity of IM-HRP enzymes binding substrate in catalysis progress compared with free-HRP.
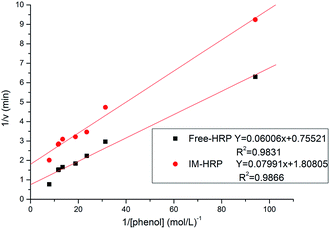 |
| Fig. 5 Kinetic of free and immobilized HRP. | |
3.5 Effects of reaction conditions on phenol removal using different enzyme-catalyzed systems
3.5.1 H2O2 dose. In this paper, experiments were carried out by choosing different dosages of H2O2 (6 g L−1, 30%; 0.2–1.5 mL) and initial phenol substrate concentrations (0.01–0.1 g L−1) to determine the phenol removal efficiency for catalysis with free/immobilized HRP enzymes. As depicted in Fig. 6, the phenol removal efficiencies of IM-HRP and free HRP reached maxima of 94% and 91%, respectively, when the H2O2 dosage was 1.0 mL. It was clear that the phenol removal percentage of IM-HRP enzymes was higher than that of free-HRP. This was very likely the result of phenol adsorption on the surfaces of the IM-HRP particles, which were very porous. The addition of excessive H2O2 could also lead to a decrease in substrate removal. Thus, high H2O2 concentration inhibited the enzyme activity. Some studies have also suggested that excess H2O2 can prohibit enzyme catalysis performance.27–29
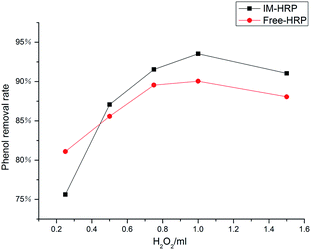 |
| Fig. 6 Phenol removal efficiency as a function of H2O2 (6 g L−1, 30%) dosage; phenol concentration was 20 mg L−1; reaction time was 25 min; temperature was 37 °C; IM-HRP dosage was 8 mg. | |
3.5.2 Initial phenol concentration. The effect of initial substrate concentration is shown in Fig. 7. The phenol removal percentage decreased sharply as the initial phenol concentration increased from 10 to 100 mg L−1. The efficiency of phenol removal with initial phenol concentration was 10 mg L−1 on the H2O2 concentration of 0.212 mol L−1, the phenol removal efficiency was reached by 92.05%. The results demonstrated that phenol degradation was highly dependent on the initial phenol concentration because higher initial phenol concentration led to low catalytic reaction rate and resulted in poor phenol removal. Therefore, more catalyst and H2O2 needed to be added to enhance organic removal.
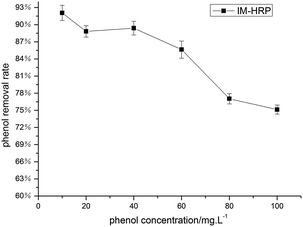 |
| Fig. 7 Phenol removal efficiency as a function of initial phenol concentration; temperature was 37 °C; H2O2 dosage was 1.0 mL (6 g L−1, 30%); reaction time was 25 min; IM-HRP dosage was 8 mg. | |
3.5.3 IM-HRP dose. The effect of IM-HRP dose on phenol removal rate is shown in Fig. 8. The maximum efficiency of phenol removal from aqueous solution was achieved with 18 mg IM-HRP (0.36 g L−1), and no obvious enhancement in phenol removal was observed with further increases in IM-HRP dosage. At the optimal catalyst dosage, the phenol removal percentage reached 92.75% at an initial phenol concentration of 16 mg L−1. This occurred because the oxidative degradation of organics was restricted at low enzyme concentration, leading to a slow reaction rate and poor phenol removal. Moreover, it should be noted that errors in experimental replication (see Fig. 8) might be attributed to the uneven distribution of HRP enzymes during HRP immobilization on hydrated titanium coatings.
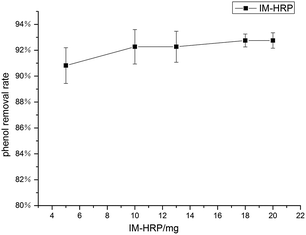 |
| Fig. 8 Phenol removal rate among the dosage of IM-HRP (phenol concentration was 16 mg L−1; 1.0 mL H2O2 (6 g L−1, 30%); reaction time of 25 min under the condition of 37 °C). | |
3.5.4 Effect of reaction time on phenol removal. Fig. 9 shows the change in phenol concentration with reaction time. More than 92.34% of phenol was removed within only 15 min, and no significant further removal was observed as time proceeded further. The phenol concentration was reduced from 16.00 to 1.23 mg L−1 after 15 min of reaction time.
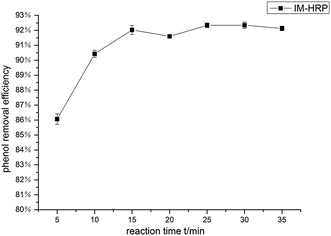 |
| Fig. 9 Phenol removal efficiency as a function of reaction time; temperature = 37 °C; phenol concentration = 16 mg L−1; 1.0 mL H2O2 (6 g L−1, 30%); 15 mg IM-HRP. | |
As shown in Table 1, phenol degradation could be fitted well with both the pseudo-second-order kinetic and pseudo-first-order kinetic equations; the apparent phenol degradation rate constants (k) were 0.02499 L (mg min)−1 and 0.54659 (min−1), and the linear correlation coefficients were 0.95 and 0.93, respectively. The fitting results revealed that the degradation of phenol was likely dependent on the concentrations of substrate and H2O2.
Table 1 Degradation rate of phenol in different reaction time
|
Kobsa |
R2b |
k is the coefficient of determination for the fit of oxidation or adsorption curves to kinetic models. R2 is the pseudo first-order rate constant, pseudo second-order rate constant and pseudo third-order rate constant of the oxidation kinetics. |
Pseudo-1st c/c0 = e−kt |
0.54659 (min−1) |
0.9321 |
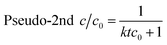 |
0.02499 (L (mg min)−1) |
0.95452 |
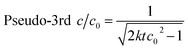 |
0.0066 (L2 (mg2 min)−1) |
0.54084 |
For the substrate, the rate of phenol degradation decreased under the condition of low initial substrate concentration. In addition, phenol degradation was not linear with H2O2 concentration; excessive H2O2 might also hinder the oxidative capacity of enzymes on phenol molecules. As for the effect of IM-HRP dosage (seen in Fig. 8), phenol removal efficiency remained around 90% at different IM-HRP dosages, revealing that the dosage of IM-HRP had no obvious influence on phenol degradation. Hence, the concentrations of both substrate and H2O2 have important effects on phenol degradation, and substrate concentration might be the more crucial factor.
3.6 Reaction mechanism of phenol degradation by IM-HRP in the presence of H2O2
The cycle of phenol removal from aqueous solutions by IM-HRP with H2O2 are summarized in Fig. 10. At first, phenol and H2O2 molecules were adsorbed to the surface of porous IM-HRP and then were involved to the enzyme catalytic process. It was reported that reactions that occurred during enzyme catalysis were involved in the one-electron oxidation of phenol compounds.30 It could be indicated that the free enzyme (IM-HRP, E) was oxidized to an active intermediate enzyme product called compound E1 by hydrogen peroxide (H2O2). Compound E1 then oxidized one phenol molecule to form free phenoxy radical (PhO˙) through a one-electron transfer. Then, another phenol molecule was oxidized into free phenoxy radical. Meanwhile, compound E2 was converted into base enzyme E. Finally, the free phenoxy radicals were released to the water environment through desorption from the surface of IM-HRP, and the free phenoxy radicals may have coupled to each other to form insoluble polymers through non-enzymatic reactions at certain concentrations.31
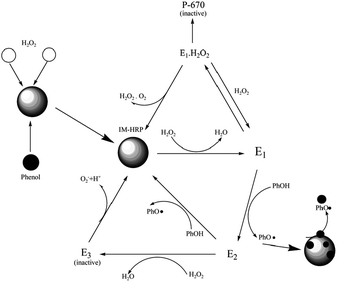 |
| Fig. 10 Model of IM-HRP catalysis on phenolic compounds in the presence of H2O2, resulting in free phenol radical. Enzyme inactive production ended in the formation of P-670 or E3. | |
For the enzyme catalytic process, several side reactions may also take place in enzyme-catalytic reactions. This could result in the denaturation of native enzymes and reduce the activity of IM-HRP enzymes. E1 could be converted to P-670, which was an inactive product of an irreversible reaction. Alternatively, it could transform into E3, which could undergo further decomposition into the native state E. However, the formation reaction of P-670 was very likely inhibited in the presence of hydrous titanium carriers, which contributed to the catalytic stability of HRP enzyme. Hence, HRP enzymes with the protection of hydrous titanium can avoid the direct contact of enzymes with strong acid/alkaline solution and protect enzymes from denaturation under high-temperature conditions to a certain degree.
4. Conclusions
In this study, the feasibility of using a biomimetic process to immobilize enzymes on hydrous titanium and the application of the immobilized enzymes to phenol removal were investigated. The experimental results revealed that the immobilization process could improve HRP enzyme stability in harsh conditions, while the free-HRP enzymes were easily affected under strongly alkaline or high-temperature conditions. The immobilization process had a very limited effect on the catalytic activity of HRP enzymes. The degradation of phenol was dependent on various reaction conditions (e.g., temperature, dosages of IM-HRP and H2O2, and reaction time); 90% of phenol could be removed under the optimal reaction conditions. Phenol was initially adsorbed on the surface of the porous hydrous titanium, and oxidative degradation then occurred with the addition of H2O2. In conclusion, this work developed a novel enzyme immobilization method that can effectively enhance the stability of HRP enzymes under harsh aqueous conditions. It is a very promising strategy for the removal of organic contaminants from drinking and/or waste waters.
Acknowledgements
This work was supported by the National Nature Science Foundation of China (No. 21277130, 51478445, 51338010 and 21477118) and the key program Nature Science Foundation of Hubei Province (2014CFA530).
References
- J. Cheng, S. Ming Yu and P. Zuo, Water Res., 2006, 40, 283–290 CrossRef CAS PubMed.
- K. Abbassian, A. Kargari and T. Kaghazchi, Chem. Eng. Commun., 2014, 202, 408–413 CrossRef.
- H. A. Asmaly, B. Abussaud, Ihasnullah, T. A. Salesh, T. Laoui, V. K. Gupta and M. A. Atieh, Evaluation of micro- and nano-carbon-based adsorbents for the removal of phenol from aqueous solutions, Taylor and Francis, 2015, vol. 97, pp. 1066–1070 Search PubMed.
- W. Wang, Z. Li, W. Liu and J. Wu, Sep. Purif. Technol., 2012, 89, 206–211 CrossRef CAS.
- Q. Huang, J. Tang and W. J. Weber, Water Res., 2005, 39, 3021–3027 CrossRef CAS PubMed.
- L. Mao, J. Lu, M. Habteselassie, Q. Luo, S. Gao, M. Cabrera and Q. Huang, Environ. Sci. Technol., 2010, 44, 2599–2604 CrossRef CAS PubMed.
- M. Monier, D. M. Ayad, Y. Wei and A. A. Sarhan, Biol. Macromol., 2010, 46, 324–330 CrossRef CAS PubMed.
- J. Xu, T. Tang, K. Zhang, S. Ai and H. Du, Process Biochem., 2011, 46, 1160–1165 CrossRef CAS.
- H. J. Kim, Y. Suma, S. H. Lee, J. Kim and H. S. Kim, J. Mol. Catal. B: Enzym., 2012, 83, 8–15 CrossRef CAS.
- M. Wagner and J. A. Nicell, Water Res., 2002, 36, 4041–4052 CrossRef CAS PubMed.
- C. Zhang, S. Chen, P. J. J. Alvarez and W. Chen, Carbon, 2015, 94, 531–538 CrossRef CAS.
- B.-W. Park, K.-A. Ko, D.-Y. Yoon and D.-S. Kim, Enzyme Microb. Technol., 2012, 51, 81–85 CrossRef CAS PubMed.
- M. Celebi, M. A. Kaya, M. Altikatoglu and H. Yildirim, Appl. Biochem. Biotechnol., 2013, 171, 716–730 CrossRef CAS PubMed.
- T.-Y. Lin, C.-H. Wu and J. D. Brennan, Biosens. Bioelectron., 2007, 22, 1861–1867 CrossRef CAS PubMed.
- F.-P. Chang, Y. Hung, J.-H. Chang, C.-H. Lin and C.-Y. Mou, ACS Appl. Mater. Interfaces, 2014, 6, 6883–6890 CAS.
- J. Zhang, F. Zhang, H. Zhang, X. Zhang, H. Liu, J. Zhang and S. Guo, Langmuir, 2010, 26, 6083–6085 CrossRef CAS PubMed.
- H. R. Luckarift, J. C. Spain, R. R. Naik and M. O. Stone, Nat. Biotechnol., 2004, 22, 211–213 CrossRef CAS PubMed.
- Q. Chang and H. Tang, Molecules, 2014, 19, 15768–15782 CrossRef CAS PubMed.
- Y. Li, H. Zhang and F. Cao, J. Sol-Gel Sci. Technol., 2011, 58, 156–161 CrossRef CAS.
- S. Yuan, T. Liu, D. Feng, J. Tian, K. Wang, J. Qing, Q. Zhang, Y. Chen, M. Bosch, L. Zou, S. J. Teat, S. J. Dalgarno and H. C. Zhou, Chem. Sci., 2015, 6, 3926–3930 RSC.
- W. E. Manual, Freehold, NJ, 1972, p. 43 Search PubMed.
- R. Zhang, A. A. Elzatahry, S. S. Al-Deyab and D. Zhao, Nano Today, 2012, 7, 344–366 CrossRef CAS.
- B. Tryba, A. W. Morawski and M. Inagaki, Appl. Catal., B, 2003, 41, 427–433 CrossRef CAS.
- Y. Jiang, W. Tang, J. Gao, L. Zhou and Y. He, Enzyme Microb. Technol., 2014, 55, 1–6 CrossRef CAS PubMed.
- A. M. Klibanov, Nature, 2001, 409, 241–246 CrossRef CAS PubMed.
- A. Hasanpour, M. Niyaifar, H. Mohammadpour and J. Amghian, J. Phys. Chem. Solids, 2012, 73, 1066–1070 CrossRef CAS.
- J. A. Nicell and H. Wright, Enzyme Microb. Technol., 1997, 21, 302–310 CrossRef CAS.
- N. Casa, J. K. Bewtra, N. Biswas and K. E. Taylor, Water Res., 1999, 33, 3012–3018 CrossRef.
- S. Wang, H. Fang, Y. Wen, M. Cai, W. Liu, S. He and X. Xu, RSC Adv., 2015, 5, 57286–57292 RSC.
- Y. Wu, K. E. Taylor, N. Biswas and J. K. Bewtra, Enzyme Microb. Technol., 1998, 22, 315–322 CrossRef CAS.
- U. Khan and J. A. Nicell, J. Chem. Technol. Biotechnol., 2007, 82, 818–830 CrossRef CAS.
Footnote |
† These authors contributed to this paper equally. |
|
This journal is © The Royal Society of Chemistry 2016 |