DOI:
10.1039/C6RA02129H
(Review Article)
RSC Adv., 2016,
6, 43989-44012
Role of functionalization: strategies to explore potential nano-bio applications of magnetic nanoparticles
Received
24th January 2016
, Accepted 13th April 2016
First published on 13th April 2016
Abstract
Advances in nanotechnology enable the production of magnetic nanoparticles (MNPs) with specific morphologies and the tailoring of their surfaces in order to manipulate their characteristics to suit specific applications. Magnetic nanoparticles are well-established nanomaterials that offer controlled size, biospecificity, and the ability to be manipulated externally. As a result, these nanoparticles can be explored with respect to a wider range of applications. It is essential to ensure the proper and specific functionalization of magnetic nanoparticles for their successful use in nano-bio applications. The current review focuses on the theoretical background regarding the functionalization of magnetic nanoparticles for nano-bio applications. The review covers all the important aspects regarding the functionalization of magnetic nanoparticles. The ideal requirements for their functionalization for nano-bio applications and the mechanisms behind this are explained. Different materials used in the functionalization of magnetic nanoparticles are classified and discussed in detail. The principle criteria for bioconjugation and different bioconjugation strategies are overviewed. Functionalization strategies for magnetic nanoparticles for specific bioapplications, such as magnetic hyperthermia, magnetic resonance imaging, target drug delivery, bacteria detection, enzyme immobilization, cell labelling, magnetic separation, and DNA enrichment, are highlighted.
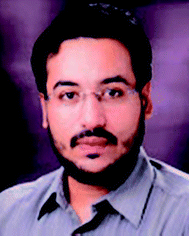 Raghvendra A. Bohara | Dr Raghvendra Ashok Bohara received his MSc degree in 2012 from the Department of Biochemistry, Shivaji University, Kolhapur, India. He obtained his Ph.D. from the Center for Interdisciplinary Research, D. Y. Patil University, Kolhapur in 2016 under the supervision of Prof. Shivaji H. Pawar. His current research interests are in the fields of nanotechnology-assisted cancer treatment, water purification and enzyme immobilization. He has published about 10 research papers to date and filed two Indian patents. |
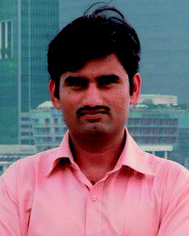 Nanasaheb D. Thorat | Dr Nanasaheb Thorat received his MSc degree in 2008 from Shivaji University, India. He obtained his Ph.D. with a gold medal from D. Y. Patil University, Kolhapur, in 2014 under the supervision of Prof. Shivaji H. Pawar. He then joined the group of Prof. Kyeong Kyu Kim as a research associate in Samsung Biomedical Research Institute, Molecular Cell Biological laboratory, Sungkyunkwan University, South Korea. In 2015, he was awarded a Fellowship and presently he is fellow of the Irish Research Council at the University of Limerick. He has published about 30 research papers to date and his current research interests are in the field of cancer nanotechnology. |
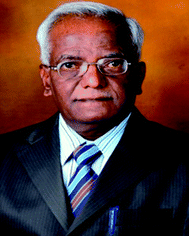 Shivaji H. Pawar | Prof. Dr Shivaji. H. Pawar is presently working as professor of Eminence in the “Centre for Interdisciplinary Research” and is the Vice-Chancellor of D. Y. Patil University, Kolhapur (India). He is an academician with a distinguished record and scholastic recognition in national and international circles of physicists. He has successfully guided 70 Ph.D. (55 in Shivaji University, Kolhapur and 15 in D. Y. Patil University, Kolhapur) students and is presently leading a research group of 50 research scholars in the Centre for Interdisciplinary Research in D. Y. Patil University, Kolhapur in the frontier areas of nano-bio materials science, nanobiotechnology, medical physics, high-Tc superconductivity and renewable energy resources at the Centre for Interdisciplinary Research. He has edited 15 books, written 35 review articles and published more than 700 research papers in national and international journals and proceedings of conferences. He has 8 patents to his credit. |
1. Introduction
The use of magnetic nanoparticles for biological and clinical applications is undoubtedly one of the most challenging research areas in the field of nano-biotechnology. The use of magnetic nanoparticles (MNPs) has witnessed noteworthy advances and holds great potential for biological applications. Magnetic nanoparticle-based biological research has rapidly advanced to a point where the research focus is shifting away from synthesis and characterization to the development and investigation of multifunctional MNPs. MNPs, specifically superparamagnetic nanoparticles, based upon their unique physical, chemical and thermal properties, offer high potential and have been proposed extensively for nano-bio applications. MNPs are eligible for various biomedical applications, such as magnetic hyperthermia, magnetic resonance imaging, target drug delivery, bacteria detection, cell labelling, magnetic separation, and enrichment of DNA.1,2 In recent years, major attention has been focused on the development of MNPs due to their fundamental properties. Firstly, their size, which generally ranges from a few nanometers to tens of nanometers, places them at dimensions that are smaller than or comparable to those of a cell (10–100 μm), a virus (20–450 nm), a protein (5–50 nm) or a gene (2 nm wide and 10–100 nm long). This means that they can ‘get closer’ to a biological entity of interest. Certainly, they can be coated with biomolecules to make them interact with or bind to a biological entity, thereby providing a controllable means of ‘tagging’ or addressing it. Secondly, MNPs exhibit interesting size-dependent superparamagnetism. Such MNPs are highly preferred due to their ability to be magnetized upon exposure to a magnetic field, but they have no permanent magnetization (remanence) once the field is turned off. Thirdly, MNPs can be manipulated by using an external magnetic field, which provides a huge advantage and opens up many nano-bio applications in vivo.3 This can be achieved by functionalization of MNPs, which can be defined as, “The addition of a chemical functional group on the surface of MNPs in order to achieve surface modification that enables their self-organization, renders them compatible and make them potential for various kinds of applications”.4
Advances in nanotechnology enable the production of MNPs with specific morphologies and the tailoring of their surfaces, in order to manipulate their characteristics to suit specific applications. Recent developments in the field of colloid science have extended our ability to fine-tune the physicochemical properties of MNPs, in order to achieve novel magnetic, optical and electronic properties compared to their bulk counterparts. These features enable tailored MNPs to operate at the biomolecular level. However, several drawbacks still need to be overcome in order to ensure an optimal bioperformance. MNPs with appropriate surface functionalization can be used to overcome these drawbacks for numerous nano-bio applications.4,5
There are three major concerns regarding the use of MNPs for nano-bio applications. The first is the stability of MNPs in water, as most biochemical processes occur in an aqueous environment. The second is health and safety issues, while exploring the effects of MNPs on the human body and the environment. The third is the conjugation of MNPs to well-defined biological molecules for targeting applications. Regarding this, there is a gap between the nature of bare MNPs and their usability for nano-bio applications. A strategy for bridging this gap is the surface functionalization and bioconjugation of MNPs to make them suitable for various nano-bio applications. Appropriate surface functionalization increases the water compatibility of MNPs in biological media, making them biocompatible and biospecific, which is a prerequisite for various nano-bio applications. MNPs need to pass through different steps for specific biomedical applications. These steps are illustrated in Fig. 1.
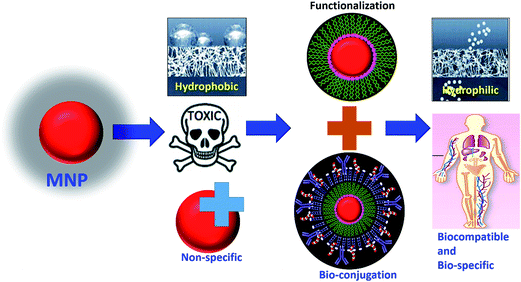 |
| Fig. 1 Strategy to bridge the gap between magnetic nanoparticles for their successful application in biology. | |
2. Ideal requirements for functionalization of magnetic nanoparticles for nano-bio applications
The successful utilization of MNPs for biological applications requires that surface functionalization should ideally impart the following properties to magnetic nanoparticles.
• It should prevent MNPs from aggregation and lead to improved colloidal stability.
• It should increase the water compatibility of MNPs.
• It should promote better magnetic controllability of MNPs.
• It should protect and stabilize the surface of MNPs.
• It should make MNPs biocompatible and nontoxic, when intended for biological and medical applications.
• It should provide bare functional groups to attach biologically active substances for nano-bio applications.
These ideal requirements for the functionalization of magnetic nanoparticles are considered in more detail as follows.
2.1. Prevention of aggregation of magnetic nanoparticles and the improvement of colloidal stability
MNPs show a tendency to self-associate, leading to aggregation and agglomeration, which are governed by thermodynamic factors and magnetism. Surface functionalization of MNPs should prevent their self-association.6 The prevention of aggregation and agglomeration, which improves the colloidal stability of MNPs, is crucial to obtaining magnetic colloidal nanofluids that are stable in both a biological medium and a magnetic field. Colloidal stability can be achieved from an equilibrium between attractive and repulsive forces. Short-range isotropic attractions are induced by van der Waals forces, while electrostatic repulsive forces can be partially screened by adding salt to the suspension. These two forces are described by the Derjaguin–Landau–Verwey-Overbeek (DLVO) theory.7,8 These forces induce anisotropic interactions. Stabilization of MNPs can be achieved by exploiting one or both of the two repulsive forces, which are electrostatic and steric repulsion.9 Controlling the strength of these forces is a key parameter in producing MNPs with good colloidal stability, which is further explained in brief as follows.
2.1.1. Steric stabilization. Steric repulsion forces have to be taken into account for MNPs that are not bare. MNPs intended for applications that require colloidal stability under high salt concentrations and over a wide pH range must be sterically stabilized. Steric stabilization relies on the polymers used for the functionalization of MNPs, and it is difficult to predict and quantify. Coating MNPs with a ligand shell or embedding them with an inorganic or polymeric matrix helps to achieve steric stabilization. The resulting repulsive potential critically depends on the polymer density, reversible binding and the solvent quality with respect to the polymers. It also depends on the molecular weight of the polymer and its density.10,11 The mechanism behind this is that if two sterically stabilized cores approach each other, polymer brushes are confined. This reduces the entropy of polymers and increases the osmotic pressure between MNPs, leading to colloidal stability (Fig. 2a).
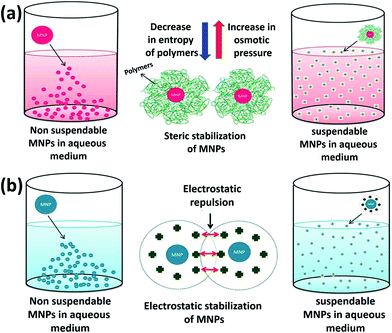 |
| Fig. 2 (a) Steric stabilization and (b) electrostatic stabilization of MNPs. | |
2.1.2. Electrostatic stabilization. Electrostatic stabilization can be understood through knowledge of the diffusion potential, which might be close to the zeta potential and the Debye–Huckel length, which is dependent upon the ionic strength and pH of the solution of MNPs.12,13 The aggregation kinetics of colloidal suspensions should be followed to test electrostatic stability by varying the added salt concentration. This can be achieved by studying the stability factor ‘W’, which reflects the effectiveness of the potential barrier that prevents the particles from aggregation. ‘W’ is the ratio of the number of collisions between particles to the number of collisions resulting in aggregation. It is expressed as W = kfast/k, where kfast is the rate constant describing rapid aggregation, (every collision leads to an aggregation) and k is the aggregation rate constant at the salt concentration used. This stability factor can be obtained through light scattering or turbidimetric measurements. The factor for colloidal stability as a function of added salt gives access to the critical concentration of salt leading to coagulation. At this concentration, the double electrostatic layer is entirely suppressed and k = kfast becomes independent of the salt concentration. Fig. 2b represents electrostatically stabilized MNPs. Consideration of both electrostatic and steric stabilization is thus necessary to obtain colloidal stability of magnetic nanoparticles.14–17
2.2. Water compatibilization of MNPs
A large proportion of MNPs used for biological applications require an organic phase for their synthesis through a high temperature process. This offers many advantages compared to aqueous synthesis. However, the resultant MNPs with apolar ligands are only soluble in organic solvents, for example chloroform, hexane, and toluene. Since, most biological processes occur in an aqueous environment, such MNPs cannot be used directly for most biological applications. Therefore, it is necessary to make MNPs water compatible, prior to their use in nano-bio applications. The terms ‘solubilization’ and ‘solution’ when applied to MNPs do not refer to the solvation of the MNPs as cores; instead, they refer to physically and chemically stable colloidal suspensions, in which MNPs do not aggregate, dissociate, or chemically react with the solvent or any dissolved gas with time. Water compatibilization of MNPs means the conjugation of unsuspended MNPs with hydrophilic ligands to give a stable colloidal aqueous solution. A suitable strategy for the surface functionalization of MNPs is necessary to overcome this problem. Water compatibilization may be carried out at either the final or an intermediate stage of the process of functionalization. To make these MNPs water compatible, their surfaces are often functionalized by either addition of surfactant or exchange by surfactant, as illustrated in Fig. 3. Addition of surfactant is achieved through the adsorption of amphiphilic molecules that contain both a hydrophobic segment and a hydrophilic component. The hydrophobic segment forms a double-layer structure with the original hydrocarbon chain, while hydrophilic groups are exposed to the outside of the NPs, rendering them soluble in water. Exchange by surfactant is the direct replacement of the original surfactant with a new bifunctional surfactant. This bifunctional surfactant has two functional groups; one is for binding to the surface of MNPs and the second, at the other end of the surfactant molecule, has a polar character so that the MNPs can be dispersed in water or be further functionalized.18
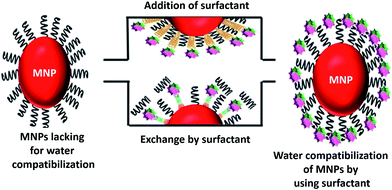 |
| Fig. 3 Water compatibilization of magnetic nanoparticles using a surfactant. | |
2.3. Magnetic controllability of MNPs
Saturation magnetization (Ms) is an essential parameter that describes the magnetic response of MNPs. Magnetic nanoparticles can be magnetically separated and transported to desired locations at ease, if their Ms remains high.19 Functionalization of MNPs makes this possible, allowing the magnetic controllability of MNPs to be achieved through clustering of particles. It is observed that the magnetic controllability of MNP clusters is higher than that of individual core/shell particles.
2.4. Protection and stability of the surface of MNPs
The surface of MNPs should be protected and stabilized from the influence of external factors, which can affect the magnetic properties; this can be achieved by surface functionalization. Functionalization should prevent reactions of MNPs with components of biological media. Materials that form oxygen-impermeable coatings are noted to provide reliable protection to MNPs.14 MNPs introduced into biosystems should be stable and their coatings must remain undamaged. Coating methods depend on the chemical nature of the material used for coating. For example, polymeric coatings can be stabilized through (i) three-dimensional polymerization of monomers on the surface of nanoparticles,20 (ii) formation of cross-links,21 and (iii) formation of a polymeric layer due to chemical bonds formed between functional groups and the surface of MNPs.22,23
2.5. Biocompatibility
The toxicity of MNPs arises from their large surface area and chemical reactivity, which generates reactive oxygen species capable of penetrating cells as well as tissues. Magnetic nanoparticles are observed to be more hazardous than their bulk counterparts, and free particles have been proven to be more toxic than fixed or bound MNPs. The size, hydrophilicity, and charge on the surface of the MNPs are key factors governing their biocompatibility. This also depends on the mass and the charge of the coating molecules. Negatively charged coatings exhibit good biocompatibility, while positively charged MNPs demonstrate better co-operation with biosystems. MNPs introduced into biosystems vary regarding their surface charge, owing to ionization of surface groups and adsorption of charged biological molecules.23–25 After functionalization, MNPs should bear one or more functional groups to attach biologically active substances for specific biological applications.
3. Methods of surface functionalization
3.1. In situ surface functionalization
This is a one-pot synthesis method. By using this method, along with functionalization, a reduced particle size and a narrow particle size distribution could be achieved. Here, within a single step, both the synthesis of MNPs and their surface functionalization are carried out. Precursors for MNPs, as well as the coating material, are simultaneously used in the reaction mixture. During the reaction, the coating process starts as soon as nucleation occurs, preventing further growth of the particles. One limitation of this functionalization method is incompatibility of functional groups with the preparation process. For example, a modifying group may react with the particle surface. For direct functionalization, carboxylates, phosphonates, and thiol groups have commonly been used.
3.2. Post synthesis surface functionalization
In this type of surface modification, the total procedure is divided into two parts, which are the synthesis of the MNPs and their surface modification. This is also known as post-synthesis surface modification. For this, bifunctional compounds are used, where a binding chelating group is initially reacted, followed by conversion of the group of the coupling site to the final functional group. The main advantage of this method is that large numbers of coupling agents are commercially available. In addition, the core–shell structure ensures strong binding of the functional groups, with a high surface coverage. The main concern with this method is that the functional group must possess a high affinity for the surfaces of the MNPs and it should not exist in the form of isolated clusters.
4. Mechanism behind functionalization of MNPs
Surface functionalization of magnetic nanoparticles is accomplished mainly via three mechanisms,26,27 which are ligand addition, ligand exchange, and encapsulation, which are described in brief as follows.
4.1. Ligand addition
Ligand addition involves the addition of a ligand to the external surface of MNPs without removal of any preexisting ligands, as shown in Fig. 4a. The four approaches to ligand addition are listed as follows: (1) addition of ligands to MNPs initially prepared with no capping agent; (2) indirect ligand addition; growth of a layer of inorganic material onto the surface of MNPs with subsequent adsorption of a ligand species directly onto this surface by ionic or other non-specific interactions; (3) exploitation of the ‘hydrophobic attraction force’ to intercalate hydrophobic species into the hydrocarbon shell of MNPs capped by ligands; and (4) formation of a covalent bond between the existing ligand and the incoming ligand.
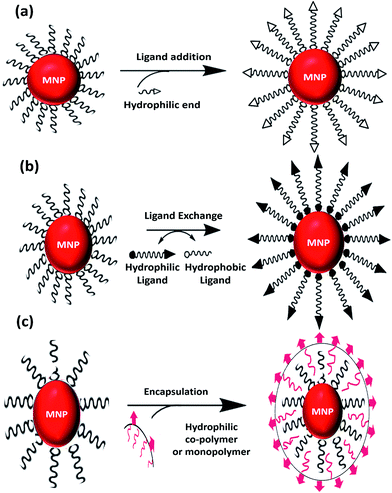 |
| Fig. 4 Surface functionalization mechanism of MNPs by different strategies (a) ligand addition (b) ligand exchange and (c) encapsulation. | |
4.2. Ligand exchange
In ligand exchange, a hydrophobic ligand is replaced by a hydrophilic one. Ligand exchange on MNPs usually involves a process where the initial hydrophobic ligands are replaced by other more strongly binding hydrophilic ligands,28 as shown in Fig. 4b. The transfer of MNPs from the organic phase to the aqueous solution takes place as a result of ligand exchange. A number of hydrophilic ligands have been reported to exchange with the natural ligand on the surface of MNPs, allowing them to form an aqueous solution.
4.3. Encapsulation
MNPs capped with hydrophobic ligands are overcoated with amphiphilic materials in the process of encapsulation. Encapsulation is achieved by intercalating the hydrophobic portion of materials with the initial ligand on the surface of the MNPs and rendering the hydrophilic portion towards the solution, as shown in Fig. 4c. MNPs become water-compatible due to the head groups within the hydrophilic portion of the materials. Moreover, the functional group can further allow for bio-conjugation or bio-modification of MNPs.
5. Materials used for the functionalization of MNPs
Different kinds of nano-bio applications are achieved by functionalization of MNPs, allowing the stabilization, specific targeting and recognition of biochemical species. Different materials can be used to fulfill the required criteria for nano-bio applications of MNPs, which effectively tailor the surface of MNPs. By using different strategies, these materials are successfully used to functionalize MNPs. These materials can be categorized by various means, which are listed in Fig. 5 and are briefly described in the following section.
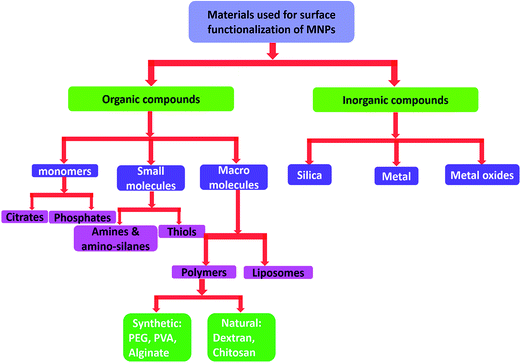 |
| Fig. 5 Materials used for the functionalization of magnetic nanoparticles. | |
5.1. Organic compounds
Organic compounds can be employed to functionalize magnetic nanoparticles during or after the synthesis of MNPs. MNPs functionalized with organic compounds possess not only the basic magnetic characteristics but also the good biocompatibility and biodegradability of these functional organic compounds. MNPs functionalized with organic compounds offer a high potential for nano-bio applications in several areas. Moreover, organic compounds can provide functional reactive groups, e.g. aldehyde, amino, carboxyl, and hydroxyl groups. These groups are able to link to an active bio substance, such as an antibody, DNA, enzyme, or protein, for further nano-bio applications. For organic molecular agents, only a single approach, ligand exchange or ligand adsorption, can be chosen for a given nano-bio application. Functionalization of MNPs with organic compounds is advantageous since, along with preservation of the magnetic properties of the MNPs, the other properties of organic molecules remain unaffected. Considering MNPs as the core, the structure of MNPs functionalized with organic compounds can be divided into three types: core–shell, matrix, and shellX–core–shellY, as presented in Fig. 6.
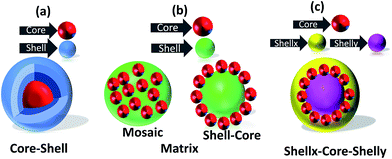 |
| Fig. 6 Presentation of the structure of (a) core–shell (b) matrix (c) shellX–core–shellY, magnetic nanoparticles functionalized with organic compounds. | |
Coating of MNPs by organic material yields a core–shell nanostructure (Fig. 6a). A matrix structure includes two typical structures: mosaic and shell–core. The mosaic structure comprises a shell layer made of organic molecules coated to any uniform MNP, whereas a shell layer, made of organic molecules adsorbing many MNPs, forms a shell–core structure (Fig. 6b). When a shell–core structure is coated again with an organic compound, it forms a shellX–core–shellY structure. The shellX may be a polymer or biomolecules; likewise, the shellY can be the same or a different functional material (Fig. 6c). Moreover, multi-component conducting organic material systems can be used to tailor MNPs to obtain specific mechanical properties, besides novel electrical, magnetic, and optical properties. Among the different organic compounds that can be used to functionalize MNPs, the following types are of particular interest because of their wide range of properties and applicability.27
5.1.1. Monomers.
(A) Citrates. The surface of magnetic nanoparticles can be stabilized in an aqueous dispersion by the adsorption of citric acid. Among various monomers, citrate moieties having multiple carboxylate functionalities have been extensively used for the colloidal stabilization of MNPs. This acid may be adsorbed on the surface of magnetite nanoparticles by coordinating via one or two of the carboxylate functionalities, depending upon steric necessity and the curvature of the surface. The functional groups are adsorbed on the surface of oxide nanoparticles by coordinating via one or two of the carboxylate functionalities, depending upon the size and the shape of the particles, and leaving at least one carboxylic acid group exposed to the solvent. The free carboxylic groups render a sufficient negative charge on the surface of particles, making them hydrophilic.29,30
(B) Phosphates. Alkanesulfonic and alkanephosphonic acid can be used as surfactants, proving to be efficient binding ligands on the surface of MNPs and satisfactory stabilizers for particle dispersion.31–33 Sahoo et al. noted the surface derivatization of MNPs by dodecylphosphonic acid, hexadecylphosphonic acid, lauric acid, oleic acid, and dihexadecyl phosphate. It is seen that alkyl phosphonates and phosphates can be used to obtain dispersions of MNPs that are thermodynamically stable. The ligands formed a quasi-bilayer structure, where the primary layer was strongly bound to the surface of the particles.34 Yee et al. proposed two possible bonding schemes for phosphonate ions on the surface of MNPs, i.e., one oxygen or two oxygen atoms of the phosphonate group binding onto the surface. It was confirmed that the phosphate ions formed bidentate complexes with adjacent sites on the surface of MNPs.35–37 Superparamagnetic magnetite nanoparticles have been prepared by controlling the coprecipitation of Fe2+ and Fe3+, using highly hydrophilic poly(vinylalcohol phosphate) (PVAP). The sizes of particles, size distribution, colloidal stability, and magnetic properties of MNPs have been studied with respect to the impact of different concentrations of PVAP. It was found that an aqueous suspension of MNPs, prepared using 1% PVAP solution, is stable for four weeks at pH 5–8. Well-dispersed cubic MNPs with a size of about 5.8 nm were confirmed by transmission electron microscopy. Dynamic light scattering measurement showed a narrow distribution of hydrodynamic size. The strong Fe–O–P bond on the oxide surface was confirmed by observing infrared spectra.38 It is concluded that phosphonates and phosphate ligands are useful for encapsulating MNPs for nano-bio applications, due to their acceptable biocompatibility.39
5.1.2. Small molecules. Functionalization of MNPs using small-molecule groups is characterized by ease of preparation and simple conjugation chemistry. These show an efficient performance regarding stabilizing the MNPs. Since nano-bio applications require a small hydrodynamic radius, using small molecules for functionalization is advantageous, as they offer a certain degree of physical barrier protection, leaving MNPs with a smaller hydrodynamic radius.40 In addition to this, they provide functional groups on the surface of MNPs, which can be used for linkage of various molecules of interest for biological applications.41
(A) Amines and aminosilanes. Short-chain amines and aminosilanes are commonly used as stabilizing agents in the fabrication of various functionalized MNPs. Barick et al. demonstrated a successful attempt to synthesize amine-functionalized Fe3O4 nanoparticles in a single step. They used the technique of thermal decomposition of Fe-chloride precursors in an ethylene glycol medium in the presence of sodium acetate and ethylenediamine. The synthesized Fe3O4 nanoparticles were found to be highly water-stable and efficient for nano-bio applications.42,43 Various other amino acids and peptides having multiple amine molecules have been used as stabilizers for successful functionalization of MNPs.44,45
(B) Thiols. Among various small molecules, compounds in the thiol category possess a higher binding affinity towards MNPs. The organosulfur compound, 2,3-meso dimercaptosuccinic acid (DMSA), having two carboxylic and two thiol groups, has been extensively used as a stabilizing agent for MNPs. DMSA-stabilized aqueous colloidal MNPs were fabricated by introducing DMSA molecules onto the surface of hydrophobic MNPs through a ligand exchange process.42 They are specifically used for the functionalization of MNPs when particles are intended for targeted drug delivery.46Maurizi et al. developed a method to stabilize free thiols onto the surface of MNPs by a post-functionalization technique, using methoxy-polyethylene glycol 2000 silane. They observed that thiol-functionalized nanoparticles were stable under physiological pH, which is considered as one of the advantages of using them for nano-bio applications.47 They have also demonstrated that the stability of thiols can be increased significantly when DMSA is protected by polyethylene glycol (PEG) chains on the surface of MNPs.
5.1.3. Macromolecules.
(A) Polymers. Polymer coatings provide an alternative option for small-molecule ligands, imparting the properties of macromolecular systems to the particle surface. Nowadays, polymer-functionalized MNPs are receiving more attention, since the polymer coatings give the MNPs excellent colloidal stability. The advantage of polymers for coating is that they increase repulsive forces, balancing the magnetic and the van der Waals attractive forces acting on the MNPs. Polymer-functionalized MNPs have been extensively investigated due to interest in their unique physical or chemical properties. To make use of these materials for nano-bio applications, well-defined MNP samples whose properties can be tuned through chemical modification are necessary. MNPs with tailored and desired properties can be produced by carefully choosing the passivating and activating polymers and/or reaction conditions. The characteristics of different natural and synthetic polymers with respect to their use as functionalizing agents for MNPs are listed in Table 1.48
Table 1 List of polymers and their characteristics regarding their use as functionalizing agents for magnetic nanoparticles48
Types of polymers |
Polymers |
Characteristics |
Natural polymers |
Dextran |
Enables optimum polar interactions with iron oxide surfaces, improves the blood circulation time, stability, and biocompatibility |
Chitosan |
Nontoxic, alkaline, hydrophilic, widely used as non-viral gene delivery system, biocompatible, and hydrophilic |
Starch |
Improves the biocompatibility, good for MRI and drug target delivery |
Gelatin |
Used as a gelling agent, hydrophilic emulsifier, biocompatible |
Synthetic polymers |
Polyethylene glycol (PEG) |
Enhances the hydrophilicity and water solubility, improves the biocompatibility, blood circulation times |
Poly vinyl alcohol (PVA) |
Prevents agglomeration, giving rise to monodispersibility |
Alginate |
Improves the stability and biocompatibility |
Polylactide acid (PLA) |
Improves the biocompatibility, biodegradability, and low toxicity in the human body |
Polymethylmethacrylate (PMMA) |
Generally used as thermosensitive drug delivery and cell separation |
Polyacrylic acid (PAA) |
Improves stability and biocompatibility as well as bioconjugation |
To coat magnetic nanoparticles, several approaches have been developed, including in situ and post-synthesis coatings. In the first approach, the MNPs are coated during synthesis, where reaction mixtures and functionalizing polymers are used simultaneously. In the post-synthesis coating method, the polymers are grafted on the MNPs after synthesis. For functionalization of MNPs, the two approaches, ligand exchange and ligand adsorption are usually applied together to form a more stable structure, due to the complex structure of the polymers.49 By exchanging or adsorbing ligands, polymers with multiple functional groups can be combined with MNPs. Polymer coating usually needs the help of active terminal groups. Regarding this, monomeric species, such as alkoxysilanes, bisphosphonates, and DMSA, have been used to promote attachment of the polymer coatings on the surface of MNPs.50,51 When MNPs are functionalized using polymers, these form a barrier between MNPs to avoid agglomeration and provide different surface properties, which gives them a high potential for nano-bio applications. Functionalizing MNPs with polymers also presents the advantage of linking MNPs with biomolecules. This is also able to change the surface charge or chemical environment. During the use of MNPs for nano-bio applications, the surface performance of the polymer-functionalized MNPs may be influenced by complex structures and characteristic properties, such as molecular weight, properties of terminal groups and the conformation of the polymers. One could use two major procedures to form polymer-functionalized MNPs. The first is manufacturing monodispersed MNPs with well-defined shapes and a controlled composition, and the second is expanding the application range by introducing functional polymers.45,48 Polymer-coating materials can be classified into natural and synthetic polymers, which are further discussed as follows.
(a) Natural polymers.
(i) Dextran.
Dextran is a polysaccharide polymer composed exclusively of R-D glucopyranosyl units with varying chain lengths and degrees of branching. Dextran has been used mostly because of its biocompatibility.51–53 An additional advantage of dextran is that it may be aminated using ammonia, which allows facile conjugation with complementary chemical groups.54 The dextran-coated MNPs are produced by reducing the terminal glucose of dextran, which has demonstrated a significant effect on the particle size, coating stability, and magnetic properties. Particles prepared with reduced dextran yield more stable coatings. Pardoe et al. observed the details of magnetic and structural properties on iron oxide MNPs formed in the presence of dextran. They concluded that the presence of the polymer limits the particle size compared to particles prepared without the polymer.55
The choice of dextran for functionalization of MNPs should be based on the size of dextran chains, which enable either chelation or hydrogen bonding with the MNP surfaces. The total bonding energy of hydrogen bonds over the length of a polysaccharide molecule is very high because of the large number of hydroxyl groups per molecule, which offers efficient bonding.56 A model for polymer adsorption, in which interactions take place at different segments of the polymer, was proposed by Jung.57 However, dextran molecules can be desorbed from the MNP surfaces by specific heating or dilution. The usual way to avoid desorption of dextran is to use agents such as epichlorhydrin, which has the capacity to form cross-links.58
(ii) Chitosan.
Chitosan is an alkaline, biocompatible, biodegradable, hydrophilic, and nontoxic polymer.59 Chitosan-encapsulated magnetic nanoparticles are receiving great interest regarding their synthesis and nano-bio applications.60–63 Lee et al. have prepared spherical MNPs and embedded them in chitosan to synthesize a ferrofluid. They noticed that such MNP-chitosan microspheres showed a strong enhancement of MR image contrast, similar to the ferrofluid in vitro.64 Kim et al. have synthesized ferrofluids containing oleic-acid-coated MNPs, which further dispersed in the chitosan. They concluded that this type of prepared MNP acts as an excellent carrier for nano-bio applications.65
(b) Synthetic polymers.
(i) Polyethylene glycol (PEG).
Polyethylene glycol (PEG) is a biocompatible, flexible, and hydrophilic polymer. The high hydrophilicity of PEG chains imparts water compatibility to the MNPs and improves the stabilization of the MNPs in aqueous media. It is observed that the PEG-coated MNPs show excellent solubility and stability, not only in aqueous solution but also in physiological media. The thickness of the coating on MNPs can be controlled by changing the molecular weight of PEG.66,67 Generally, the post-synthesis coating method is preferred for synthesizing PEG-derivative-functionalized MNPs. The high stability and better dispersion of MNPs in biological media can be achieved by using PEG of high molecular weight with more branched chains. Recently, polymerized polyethylene glycosylated bilayers were used to prepare novel superparamagnetic iron oxide nanoparticles.68 Various methods of polymer coating were developed to prepare small and ultra-small particles without size-separation processes. Cheng et al. successfully modified porous hollow Fe3O4 nanoparticles by synthesizing heterobifunctional PEG ligands using 3-(3,4-dihydroxyphenyl) propanoic acid and PEG as reactants.23 Fang et al. have developed a nanoparticle platform comprised of a superparamagnetic iron oxide core, which was surface-modified with poly(ethylene glycol) (PEG) molecules bearing amine functional groups for further bioconjugation. The stability in biological environments of this system was evaluated and demonstrated to be suitable for further development for nano-bio applications and in vivo use.69
(ii) Polyvinyl alcohol (PVA).
Polyvinyl alcohol (PVA) is a biocompatible, hydrophilic polymer. MNPs functionalized with PVA were prevented from self-agglomeration, which results in monodispersed MNPs.70–73 PVA is a synthetic polymer, which can be transformed into a polymer gel with specific properties.70 The synthesis of an aqueous ferrofluid and the preparation of a magnetic gel with PVA and glutaraldehyde have been reported by Albornoz et al. The magnetic gel can be dried to generate a biocompatible film.74 Lee et al. have modified the surface of magnetic nanoparticles with PVA by precipitation of iron salts in PVA aqueous solution to form a stable dispersion. They found that the PVA irreversibly binds to the surface of MNPs.75 Chastellain et al. have synthesized PVA-coated iron oxide nanoparticles. They concluded that the colloidal stability of the final polymer-coated product as well as the resulting particle size distribution differs with different iron/polymer ratios.76
(iii) Alginate.
Alginate is an electrolytic polysaccharide with many carboxyl groups. The carboxyl groups of alginate and iron ions interact, resulting in electrostatic repulsion. This makes a stable assembly of MNPs-alginate. A preparation of iron oxide nanoparticles with alginate has been developed.77–80 Assembling iron oxide nanoparticles with alginate consists of three steps: (a) gelation of alginate and ferrous ions, (b) in situ precipitation of ferrous hydroxide by the alkaline treatment of alginate, and (c) oxidation of ferrous hydroxide with an oxidizing agent. A new modified two-step coprecipitation method has been developed by Ma et al. They observed that typical iron oxide nanoparticles (Fe3O4) had a core diameter of 5–10 nm and that alginate-coated superparamagnetic iron oxide nanoparticles had a hydrodynamic diameter of 193.8–483.2 nm.81 Morales et al. have also described a new method for synthesizing magnetic iron oxide nanoparticles into alginate beads with a controlled size and desired magnetic properties. Their results suggest that the use of a polymer limits the particle size.82 MNPs coated with different polymers, resulting in different assemblies, are illustrated in Fig. 7. In polysaccharide coating and coating with copolymers, the resulting particles are found to be uniformly encapsulated cores. In another coating approach, polymer molecules anchored to a magnetic particle surface form a brush-like structure.83
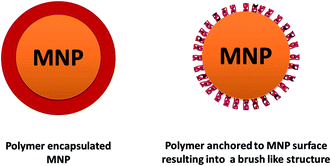 |
| Fig. 7 Illustration of the assembly of different polymers and magnetic nanoparticle cores. | |
(B) Liposomes. Liposomes consist of a lamellar-phase lipid bilayer. They are biocompatible. Amphipathic liposomes can encapsulate MNPs, since they possess a bilayer structure. An advantage of liposome encapsulation is that a certain number of MNPs can be gathered for collective target delivery. For these reasons, liposome complexes could be used efficiently for delivery of contrast agents in MRI.84,85 The main advantage of using liposomes is that they possess a large encapsulation range, so that different biomolecules, such as proteins, DNA and their complexes with MNPs, can all be encapsulated by liposomes as one unit.86
5.2. Inorganic compounds
One of the major challenges in functionalizing MNPs is finding a common ligand for ubiquitously capping the MNPs. However, when organic compounds are considered as functionalizing agents, it is necessary to select specific head groups of ligands that have a high affinity for the MNPs.87 Additionally, when using organic compounds, simultaneous control of the shape, stability, biocompatibility, surface structure, and magnetic properties of MNPs are still subjects of concern, which limits the use of such MNPs for nano-bio applications. On the other hand, coating MNPs with an inorganic layer or shell is of great interest. MNPs functionalized with inorganic compounds can exhibit greatly enhanced antioxidant properties when compared to bare MNPs. In addition, the use of inorganic compounds for functionalization of MNPs extends the scope of nano-bio applications. MNPs functionalized with inorganic compounds are very promising for various nano-bio applications, for example, biolabeling, bioseparation, and catalysis. The inorganic materials include silica, metals, nonmetals, metal oxides, and sulfides. Silica (SiO2), gold (Au) and silver (Ag) are extensively used for surface modification of MNPs, forming core–shell structures and providing stability to the MNPs in solution, further helping to bind various biological molecules and drugs to the surface of the MNPs through suitable functional groups.15,48
5.2.1. Silica. Silica has been extensively used as a coating material for magnetic nanoparticles. Silica-coated MNPs always form a core–shell structure. The functionalization of silica shells is similar to ligand adsorption. Silica has many advantages over organic coatings. Silica-coated MNPs are colloidally stable, photostable, robust, and water-soluble.17,88 Usually, an inert silica coating on the surface of MNPs prevents their aggregation in liquid, improves their chemical stability, and provides better protection against toxicity. The MNPs are stabilized by silica coatings via two different methods. One is to shield the magnetic dipole interaction with the silica shell. The second is imparting MNPs with negative charge, thus enhancing the coulomb repulsion between them.89,90Magnetic silica nanospheres can be prepared by three different methods. The first method is related to the well-known Stöber process, in which silica was formed in situ through the hydrolysis and condensation of a sol–gel precursor, such as tetraethyl orthosilicate (TEOS). For example, Im et al. prepared silica colloids loaded with superparamagnetic iron oxide nanoparticles using this process.91 They observed that the final size of silica colloids depends upon the concentration of iron oxide nanoparticles and the type of solvent. Larger colloids were obtained at lower concentrations of iron oxide nanoparticles. The second method is based on the deposition of silica from silicic acid solution. The silicic acid method is more efficient, covering a higher proportion of the MNP surfaces than the TEOS method. This method is very easy to perform, and by changing the ratio of SiO2/MNPs or repeating the coating procedure, the particle size can be easily controlled. The third method is an emulsion method, where the silica coating is controlled and confined using micelles or inverse micelles. This method requires the separation of the core–shell MNPs from a large amount of surfactants associated with the emulsion system. Yang et al. have used this method for the preparation of monodispersed silica-coated iron oxide superparamagnetic nanoparticles and succeeded in entrapping the biological macromolecules in the pores of the prepared nanoparticles.92 Tartaj et al. have prepared a submicronic silica-coated magnetic-sphere aerosol.93,94 One of the advantages of enrichment of MNPs with silica is the presence of surface silanol groups that can easily react with coupling agents, so that specific ligands can be covalently attached to MNPs.95,96 For example, amine groups have been introduced on the surface of silica-coated magnetite nanoparticles by hydrolysis and condensation of an organosilane, on the surface of MNPs. This method is known as silanization. The silica shell tends to adsorb molecules, but silane-coupling agents can significantly inhibit this process. At one side, these agents consist of siloxy, linked with silica shells and at another side, they consist of biocompatible groups, such as amino and sulfydryl, linked with biomolecules or even biomolecules themselves that already incorporate a silane group. Biomolecules can easily be added to the outer shells by using alkoxysilanes with active groups, such as aminopropylsilane or mercaptopropylsilane.
5.2.2. Metal element coating. Metallic elements used for surface modification act as a protective layer and are relatively inert. The coating metal and MNPs are tightly coupled through a chemical reaction process. The metal coating can be more easily biofunctionalized than the bare surface of the MNPs. Gold (Au) is the major element among noble metal coatings. Gold offers remarkable advantages if coated along with strong conjugation with sulfur. Gold-coated MNPs are completely stable, because of the chemical inertness of gold. Wang et al. synthesized gold-coated iron-oxide nanoparticles by reducing gold precursors on iron oxide nanoparticles.97 Lyon et al. synthesized gold-coated iron-oxide nanoparticles by hydroxylamine seeding.98 The main advantage of the gold coating is that the magnetic properties of the nanoparticles remain independent of the extent of gold addition. A convenient method of synthesizing core@shell structured Fe3O4/Au by reducing HAuCl4 on the surface of Fe3O4 nanoparticle seeds was reported by Xu et al.99 Lin et al. have synthesized core-shell-structured Fe/Au nanoparticles by a reverse-micelle approach.100 Ramasamy et al. designed a new gold nanorod conjugated with a MNP composite.101 The gold nanorod-MNP performed as a photo thermal agent for bacteria.Silver is another element among noble metal coatings. It is found that silver-coating makes MNPs germicidal, since silver has a very strong sterilization ability.102 The study of silver coatings is very similar to that of gold. Silver can form both core–shell structures and heterostructures.103,104 Chen et al. synthesized Fe3O4@C@Ag hybrid nanoparticles. This nanosystem shows a combination of drug release, magnetic resonance imaging, and two-photon fluorescence (TPF) imaging, owing to the carbon and silver on its surface, which leads to a multifunctional system for medical diagnosis and therapy.16 Silver NPs have shown moderate antibacterial properties with various bacteria.105,106 Gold and silver together can produce synergistic effects and better biomedical properties. Some rare-earth elements can also be used in surface modification via the formation of core–shell structures. For example, a Fe3O4@NaLuF4:Yb,Er/Tm core–shell nanostructure with multifunctional properties was developed by stepwise synthesis.107
5.2.3. Metal oxides/sulfides. Metal oxide or metal sulfide-functionalized magnetic nanoparticles possess unique physical or chemical properties. Hong et al. prepared ZnO-coated Fe3O4 nanoparticles by coating Fe3O4 nanoparticles by direct precipitation using zinc acetate and ammonium carbonate. They found that the functionalized nanoparticles possessed better antioxidant ability than the bare Fe3O4 nanoparticles.108 When MNPs are coated with magnetic materials, a dramatic influence on the magnetic properties of the nanoparticles is noticed. The combination of two different magnetic phases will lead to new functionalized MNPs, with many possible applications. Cheon et al. have fabricated highly ordered binary Fe3O4@CoFe2O4 nanoparticles, where the saturation magnetization of the nanoparticles (Ms) has been greatly enhanced and a huge increase in coercivity (Hc) is seen. In addition, MNPs functionalized with optical and electrical materials are enabled for a wider range of applications.109,110
6. Bioconjugation
From the above text, it has been seen that the effect of surface functionalization can help to enhance the water compatibility as well as the colloidal stability of MNPs, and provide desired functional groups and stability in biophysiological environments. MNPs are also required to conjugate with biomolecules of interest (e.g. small bioactive molecules, peptides, proteins, and nucleic acid) for further biomedical applications. Many conjugation strategies have been explored in this direction. The choice of conjugation strategy is initially decided by a combination of factors, which are the size, shape, structure, surface chemistry, and the intrinsic material of the MNPs, along with the nature of the surface ligands on the MNPs and their available functional groups. The choice of conjugation strategy also depends on the type of biological molecule, its size, its chemical composition, and its utility in nano-bio applications.
6.1. The principle criteria for bioconjugation
The principle criteria that would allow controlled attachment of biomolecules to the surface of an MNP are as follows.111 Different types of nano-bio applications have always required a different ratio of biomolecules to MNPs. Monovalency can be used to identify and correlate single binding events, while higher valencies can improve binding interactions and avidity; conversely, over-conjugation can potentially impair binding interactions. Valency should be easily controlled with minimal variation across a bioconjugate. Protein, enzyme, and antibody activities are dependent upon their binding sites having access to the environment. Nonspecific chemistry or electrostatic interactions can result in heterogeneous attachment and impair activity in the final conjugate. For some nano-bio applications, it is necessary that the separation between MNPs and biomolecules should meet rigid distance requirements, for example, sensing schemes utilizing Forster resonance energies. Within some conjugates, the linkage should be permanent, while in others a labile linkage is more desirable, such as in the case of MNP-mediated drug delivery. In all cases, the chemistry should not alter the properties of each participant, for example, causing loss of protein structure or activity or loss of stability or optoelectronic properties of MNPs. Maximum utility will arise from being able to reproduce the previous five criteria between experiments, between different types or batches of MNPs, and with different biological molecules.
6.2. Overview of different bioconjugation strategies
A number of chemical approaches have been used for the conjugation of biomolecules with MNPs for different biomedical applications. These can be categorized into physical interactions and covalent chemistry (Fig. 8). The choice of chemistry is dictated, in part, by the chemical properties, functional groups found on the MNP surfaces, biomolecules to be linked, and end applications for which the assembly is used.
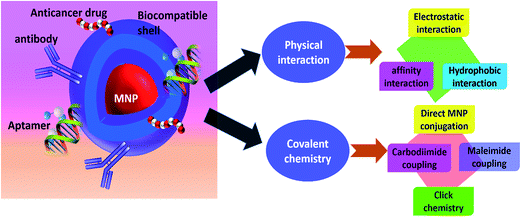 |
| Fig. 8 Different strategies used in the bioconjugation of MNPs. | |
6.2.1. Physical interactions. There are several unique advantages of this type of chemistry, including rapid binding, high efficiency, and no need for intermediate modification steps. This particular category includes electrostatic, hydrophilic/hydrophobic, and affinity interactions (Fig. 9). Among these, the simplest and most widely used bioconjugation approach is an electrostatic attachment, as shown in Fig. 9a. The underlying principle behind the electrostatic attachment is the attraction between opposite charges. Electrostatic interactions have proved particularly useful in the assembly of plasmid DNA onto the surface of MNPs. Here, bioconjugation is facilitated by the strong negative charge associated with the phosphate backbone of most nucleic acids conjugating with positively charged MNPs; however, pH and ionic strength need to be carefully optimized.112 Based on this, several research groups have demonstrated this utility by creating SPIONs coated with cationic polymers of PEI, which then complexes with negatively charged plasmid DNA molecules.113–116
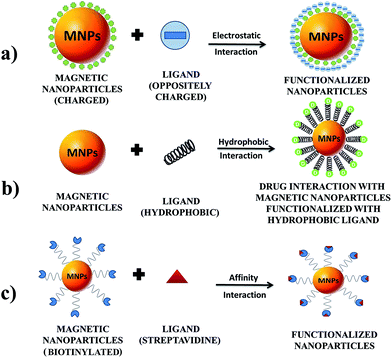 |
| Fig. 9 Different modes of direct absorption bases upon (a) electrostatic interaction (b) hydrophobic interaction and (c) affinity interaction. | |
Hydrophobic/hydrophilic interactions are shown in Fig. 9b; these have proven highly useful when adsorbing hydrophobic drugs onto MNPs. For this application, MNPs are engineered with hydrophobic layers that can adsorb hydrophobic drugs, which are then triggered to release intracellularly when the coating degrades.117 Affinity interactions, on the other hand, have proven to be very effective for bioconjugation of targeting ligands to the surface of MNPs, as shown in Fig. 9c. The surface of MNPs can be modified with streptavidin, which specifically binds biotinylated molecules. The linkage formed is highly stable and is the strongest of all non-covalent linkages. Unlike hydrophobic and electrostatic interactions, affinity binding is not sensitive to environmental conditions, such as changes in pH, salinity, or hydrophilicity.118
6.2.2. Covalent chemistry. Depending upon the particular combination of MNPs with biological molecules, either the MNP surface itself or a surface-bound stabilizing ligand can be directly linked to the biological molecule. Covalent linkages are strong and stable bonds, which can be specifically formed between functional groups, typically amino, carboxylic acid, and thiol groups found on the MNP surface. These functional groups can be cross-linked with different biomolecules either directly or by using various coupling strategies based on the functional group involved. Some of the coupling strategies are discussed below.
(A) Direct magnetic nanoparticle conjugation. In a direct MNP conjugation strategy, the functional groups present on the MNP surfaces are directly involved in forming a bond with a ligand. Fig. 10 shows different direct magnetic nanoparticle conjugating strategies. Here, surfaces of particles are functionalized with different functional groups, such as amine, sulfhydryl and aldehyde, with possible conjugating strategies with different biomolecules [Fig. 10(a–c)]. Generally, these strategies are more suitable for conjugation of small biomolecules, such as dyes, small peptides and antibodies. However, the efficiency of these chemistries may vary. With the exception of amine-functionalized MNPs, direct conjugation methods are susceptible to intercalating or cross-linking and thus direct conjugation has limited applicability for biomolecule attachment.118,119
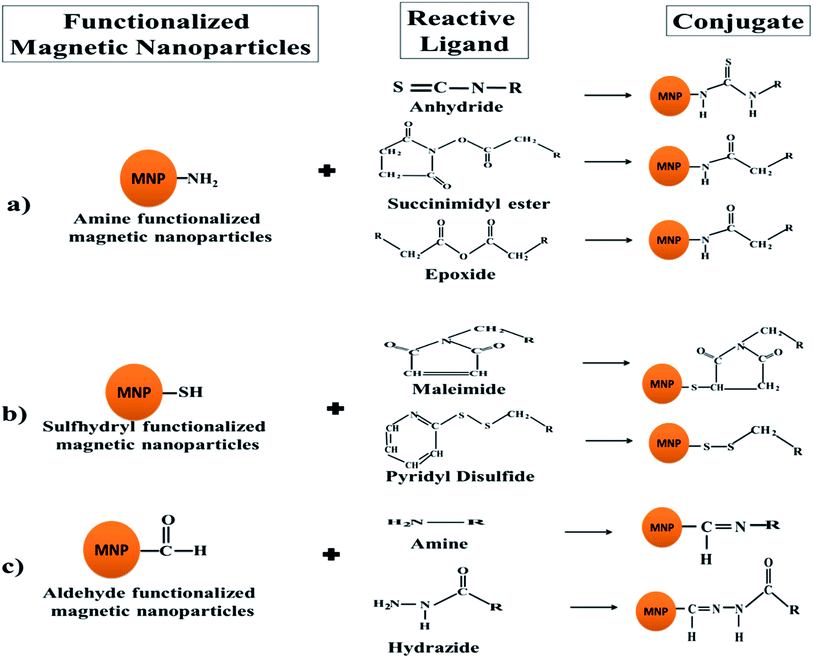 |
| Fig. 10 Different direct magnetic nanoparticle conjugating strategies. | |
(B) Carbodiimide coupling reaction. Carboxylic acids are covalently linked to primary amines via formation of a ‘zero length’ amide bond, by a ‘carbodiimide coupling’ strategy. This procedure is advantageous, since it does not require any lengthy linker species, allowing the hydrodynamic radius of the MNPs to be minimized. For the most common covalent coupling strategy, 1-ethyl-3-dimethylaminopropyl carbodiimide (EDC) is used as a coupling agent.76 This conjugation strategy has been applied for coupling of various proteins (e.g. enzymes and antibodies), amine-terminated nucleic acid, and small molecules with amine groups, to the carboxylic-group-functionalized MNPs.120–122 Coupling between amine-functionalized MNPs and carboxylic groups of biomolecules was also found.122 For more specificity, the coupling efficiency can be enhanced by the addition of stabilizing agents such as n-hydroxysuccinimide (NHS) or sulfo NHS by formation of a succinimide ester intermediate.123 This strategy has been applied for coupling of enzymes to MNPs, with retention of up to 50% to 80% of enzymatic activity, depending on the enzyme that has been used.121,122Fig. 11 represents a reaction scheme for EDC–NHS crosslinking with amine-functionalized MNPs.
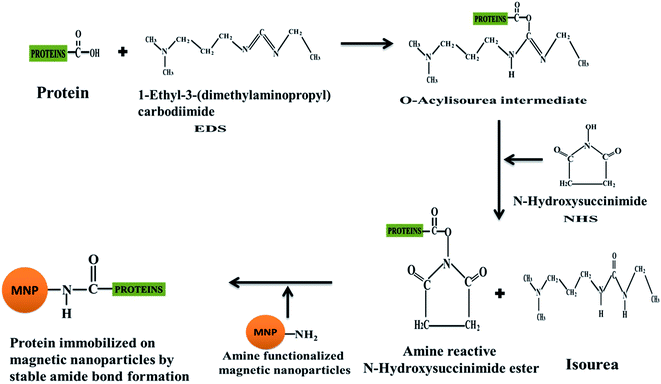 |
| Fig. 11 Reaction scheme for EDC–NHS crosslinking with amine functionalized magnetic nanoparticles. | |
(C) Maleimide coupling. A maleimide may be used to conjugate primary amines to thiol groups, which are present in proteins.124 The most commonly used maleimide-derived coupling reagent is sulfo-SMCC, i.e. sulfosuccinimidyl-4 (maleimidomethyl) cyclohexane-1-carboxylate. Maleimide coupling has been used to conjugate biomolecules, such as DNA and proteins, to the surface of MNPs.125 Fig. 12 shows amine-functionalized NPs activated by sulfo-SMCC and conjugating with the thiol group of protein to give a stable MNP-protein conjugate.
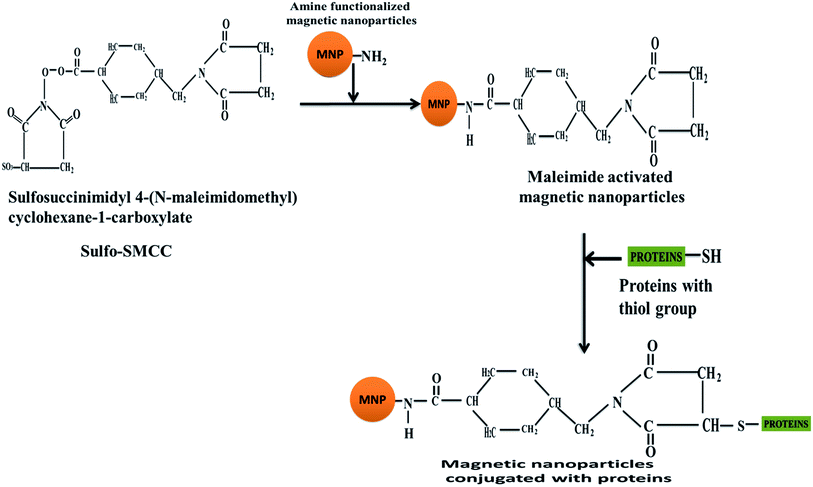 |
| Fig. 12 Reaction scheme for SMCC-mediated conjugation of protein on the surface of magnetic nanoparticles. | |
(D) Click chemistry. Kolb et al. developed a relatively new approach for direct conjugation, known as “click” chemistry.126 The reaction basically involves coupling of an alkyne to an azide, giving a 1,2,3-triazole ring under catalysis by Cu(I) (Fig. 13). By way of click chemistry, bioactive surfaces are easily conjugated to biomolecule ligands with less harsh conditions than with other routes.127 Specifically, click reactions are fast, efficient, require mild reaction conditions, an aqueous environment, and create water-soluble and biocompatible linkages (electron configuration similar to amide bonds) at neutral pH. Compared to other conjugation strategies, this method offers several unique features. Firstly, azide and alkyne reactive groups show high specificity for each other, and remain unreactive with most other functional groups, which ensures specific conjugation on the reactive moiety at the desired location. Secondly, the bonds formed are highly stable, in contrast to amide bonds, which are cleaved by hydrolysis reactions, and disulfide linkages, which are susceptible to cleavage under reducing environments. Thirdly, the linkages formed are extremely rigid, which helps to maintain the conformation of reacting moieties at the MNP surfaces and prevents cross-interactions. Because of these features, the production of linkages with high orientation and engineering to ensure optimal reaction activity and efficiency is possible. This chemistry has been implemented for MNPs, showing 90% efficiency for binding targeting molecules under mild reaction conditions. However, the procedure has the disadvantage that preparation of azide or alkyne-functionalized bioactive species is often lengthy and low-yielding. Even though the formed linkages are biocompatible, the Cu catalyst needed to drive the reaction can create problems in vivo due to improper purification before use. Excessive Cu consumption has been linked to a number of disorders. More investigation is needed on the long-term toxicity of MNPs developed using click chemistries.
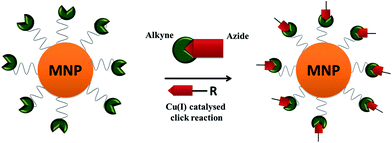 |
| Fig. 13 Example of Cu(I)-catalyzed azide/alkyne-‘click’ chemistry for magnetic nanoparticle surface modification.128 | |
7. Functionalization of MNPs for specific nano bioapplications
The use of magnetic nanoparticles for biological and clinical applications is undoubtedly one of the most challenging research areas in the field of nanomagnetism. In recent years, much attention has been focused on the development of MNPs due to their fundamental properties. MNPs are eligible for various biomedical applications, such as magnetic hyperthermia, magnetic resonance imaging, target drug delivery, bacteria detection, cell labeling and magnetic separation, and enrichment of DNA (Fig. 14). Brief descriptions of these bioapplications of magnetic nanoparticles are given in the following section.
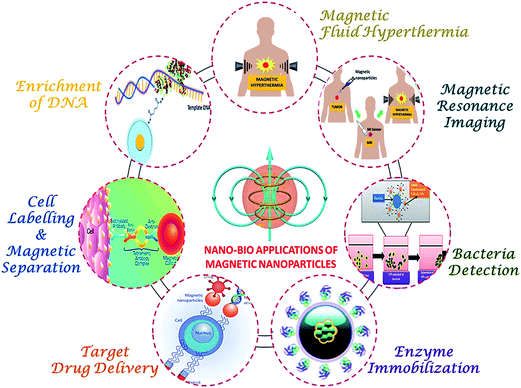 |
| Fig. 14 Representing different nano-bioapplications of magnetic nanoparticles. | |
7.1. Magnetic hyperthermia
Hyperthermia is one of the modes of cancer treatment, where cancer cells are killed by exposing them to high temperatures (42–46 °C). Hyperthermia offers an attractive approach for the treatment of cancer because, as a local therapy, it is associated with fewer side effects in comparison with chemo and radiotherapy. Additionally, it can be used in combination with all conventional modes of treatment. Various clinical trials have demonstrated the efficacy of such combinations.129–132 Functionalized magnetic nanoparticles in fluid form have been used for generating heat for hyperthermia treatment as a promising tool for the treatment of cancer. When such magnetic nanoparticles are injected into a tumor and exposed to an alternating magnetic field, they become a source of heat capable of raising a sufficiently high temperature to weaken or kill the cancerous cells. The absorption efficiency of any material to generate heat due to an applied magnetic field is measured in terms of its specific absorption rate (SAR) or specific loss power (SLP). These terms are generally used to define the transformation of magnetic energy into heat.133,134 MNP-based hyperthermia involves the distribution of particles throughout the targeted tumor site, followed by the generation of heat to the tumor using an external AC magnetic field. A mechanism of heating by magnetic nanoparticles includes three types of processes, which are hysteresis losses, Neel and Brownian relaxation. The crystal size and composition of the particles play an important role in the relative contribution of each process.135 Magnetic nanoparticles with core diameters of less than 20 nm are single-domain particles, which means that they consist of only a single organized crystal. The external rotation (Brownian) and internal (Neel) diffusion of the particle's magnetic moment in combination govern the magnetization relaxation in such small magnetic nanoparticles with a negligible contribution of hysteresis loss.136 The characteristics of well-dispersed magnetic nanoparticles, such as high magnetic crystalline anisotropy, moderate saturation magnetization, and small superparamagnetic size play an important role in the controlled heat generation, which makes them the appropriate choice for hyperthermia.137 The heat generated by applying an AC magnetic field not only depends on the properties of the MNPs (composition, size, and shape) and the frequency and amplitude of the magnetic field, but it also depends on the functionalization of the MNPs.138
An acrypol-934-stabilized γ-MnxFe2−xO3 suspension caused the death of HeLa cells as a result of hyperthermia induced by the application of an AC magnetic field. Significant disruption of the actin and tubulin cytoskeletons was seen in the cells, which led to cell death. It was found that the cell death was proportional to the duration of the applied AC magnetic field and the quantity of the particles.139 An acrypol, dextran, chitosan and pluronic F127-stabilized Tc-tuned biocompatible aqueous suspension of La1−xSrxMnO3 magnetic nanoparticles was synthesized for hyperthermia treatment of cancer.140–143 Cobalt zinc ferrite MNPs functionalized with triethylene glycol were recently studied for hyperthermia application by our group.144 The synthesized MNPs were found to be biocompatible, with a cell viability of more than ∼80%. The same was confirmed by multiple assays performed by using multiple cell lines. Up to 80% of MCF7 cells were found to be killed within 60 min, when subjected to in vitro hyperthermia treatment by triethylene glycol-functionalized cobalt zinc ferrite magnetic nanoparticles. Superparamagnetic iron oxide nanoparticles functionalized with folic acid and cyclodextrin were synthesized for drug conjugation. The heat generated by these particles under a high-frequency magnetic field was found to be useful for hyperthermia treatment and it also acted as a driving force for drug release. The drug release was triggered by induction heating.145 The effect of bimagnetic Fe/Fe3O4 core/shell nanoparticles on the growth of subcutaneous mouse melanomas was evaluated recently. These nanoparticles were protected by functionalizing with organic dopamine-oligoethylene glycol ligands, so that biocorrosion did not take place. After intratumoral injection, the micromolar concentrations of iron given within the modified core–shell Fe/Fe3O4 nanoparticles caused a significant anti-tumor effect on melanoma.146 Meenach et al. prepared hydrogel nanocomposites containing magnetic nanoparticles, which showed remote heating of the tumor tissue upon exposure to an external alternating magnetic field. The swelling behavior of the systems was dependent on the ethylene glycol content and cross-linking density. A larger amount of ethylene glycol and lower crosslinking resulted in higher volume swelling ratios. The entrapped iron oxide nanoparticles and hydrogel nanocomposites showed potential biocompatibility by exhibiting high cell viability for murine fibroblasts. The heating response was dependent on the iron oxide loading in the gels and the strength of the magnetic field. M059K glioblastoma cells were selectively killed on exposure to an alternating magnetic fluid by the hydrogel nanocomposites.147 Poly(N-isopropylacrylamide)-chitosan-based nanohydrogels and iron oxide magnetic nanoparticles encapsulated by magnetic nanohydrogels were investigated by Jaiswal et al. The lower critical solution temperature of poly(N-isopropylacrylamide) increased with an increasing weight ratio of chitosan to N-isopropylacrylamide. It was found that chitosan served as a crosslinker during polymerization as well as playing a critical role in controlling the growth of nanohydrogels and enhancing the lower critical solution temperature of poly(N-isopropylacrylamide). The presence of chitosan makes the composite pH-sensitive, so that the temperature and pH changes trigger the drug release. Additionally, the encapsulation of iron oxide nanoparticles into hydrogels caused an increase in the lower critical solution temperature. The temperature-optimized nanohydrogels and magnetic nanohydrogels were fabricated with a lower critical solution temperature close to 42 °C, which is the hyperthermia temperature. It was found that magnetic nanohydrogels show optimal magnetization, a good specific absorption rate under an external AC magnetic field, and excellent biocompatibility with L929 cell lines. This suggests potential applications in combination therapy involving hyperthermia treatment of cancer and targeted drug delivery.148 Recently, a magnetic hyperthermia application using La1−xSrxMnO3 and bypass gel of La1−xSrxMnO3 and γ-AlxFe2−xO3 was demonstrated. The La1−xSrxMnO3 was found to be useful for self-regulating the temperature and the γ-Al0.07Fe1.93O3 contributed to the biocompatibility and higher SAR values. It was found that with an increase in the ratio of Al-substituted maghemite, the value of the SAR increased. Such biphasic gel was found to have potential with respect to magnetic hyperthermia with control of temperature in vivo.149,150 The magnetic nanoparticles coated with thermoresponsive polymer can be used for simultaneous magnetic drug targeting, involving hyperthermia followed by drug release. Kim et al. found that by using thermo-responsive polymers, the self-heating from magnetic nanoparticles under an AC magnetic field can be used either for hyperthermia or to trigger the release of an anti-cancer drug.151 It was shown that CoFe2O4 nanoparticles acted as heating agents for hyperthermia and thermo-drug delivery. A suspension of γ-Fe2O3 (ref. 152) and γ-AlxFe2−xO3 (ref. 153) nanoparticles in cellulose was synthesized, which appeared to have excellent biocompatibility and applicability for hyperthermia treatment of cancer. By using magnetic nanocrystals and mesoporous silica nanoparticles together, a unique drug delivery system can be developed. Magnetic nanocrystals have the potential to exhibit hyperthermia effects when placed in an oscillating magnetic field, while mesoporous silica nanoparticles can contain and release drugs. This dual ability makes the system very promising. For example, zinc-doped iron oxide nanocrystals can be incorporated within a mesoporous silica framework and the surface can be modified with pseudorotaxanes. When an alternating current magnetic field was applied, the nanocrystals generated local internal heat, disassembling the molecular machines, and allowing the drugs to be released. Heat generated by MNPs under a high-frequency magnetic field is useful for hyperthermia as well as for drug release.154 When composites of maghemite nanoparticles embedded in an ordered mesoporous silica-matrix, forming magnetic microspheres, were exposed to a low-frequency alternating magnetic field, they showed the ability to induce magnetic hyperthermia. These particles showed good biocompatibility for human cells.155
7.2. Magnetic resonance imaging
For proper medical diagnosis and treatments, proper imaging of internal organs with high spatial resolution is essential. Magnetic resonance imaging (MRI) is a very important imaging technique, since it enables extraordinarily high temporal and spatial resolution without any invasive procedures. MRI is the standard clinical test to determine the presence, location and size of a tumor. However, this technique has not been able to reach the resolution required to diagnose metastatic cancer or individual cancer cells. Hence, nanoscale materials have been focused for better imaging.134 In MRI, computer-assisted imaging is done by achieving relaxation signals of proton spins within the human body, excited by radiofrequency waves in a strong magnetic field. Although MRI itself gives detailed images, it may not be accurate, since normal tissues and lesions often show only small differences in relaxation time. To overcome this, MRI contrast agents can be used to clarify images and allow better interpretation. Increased recognition of magnetic nanoparticles as MRI contrast agents is resulting in visualization of disease-specific biomarkers at the molecular and cellular levels. There are two independent relaxation times, known as the longitudinal and transverse relaxation (T1 and T2), which are used to generate the magnetic resonance image.156 Gd-based contrast agents enhance the signal of T1-weighted images.157 On the other hand, superparamagnetic iron oxide (SPIO) provides a strong contrast effect in T2-weighted images, due to its different contrasting mechanism.158 This was the first nanoparticulate MRI contrast agent; it has received great attention since its development as a liver contrasting agent and it is still used clinically.159 In addition to this, the nanoparticulate properties of these materials are advantageous over conventional imaging agents regarding different bio distributions; r1 and r2 are the longitudinal and transverse relaxation rates per millimole of agent, respectively, and can be determined experimentally from the slope of the relaxation rate curves, which represent the contrast agent relaxivities. The r2 relaxivity remains proportional to the saturation magnetization and the concentration of the MNPs. For a T2 contrast agent, a higher value of r2/r1 leads to better T2 contrast efficacy. Among the r2/r1 values for MnFe2O4, Fe3O4 and CoFe2O4, the cobalt ferrite MNPs show higher values and offer excellent contrast. Among these three compositions, the cobalt ferrite nanoparticles have the highest magnetization, initial susceptibility, and anisotropy energy, leading to high r2 proton relaxivity. The cobalt nanoparticles have a larger effect on proton relaxation, resulting in an improved magnetic resonance contrast and allowing smaller particle cores to be used without compromising the sensitivity.160 Along with the basic requirements, the other variables that govern the use of MNPs as a contrast agent in MRI are cytotoxicity, particle size, surface charge, and ability to detect the cancer early. All these variables determine the nanoparticle biodistributions and their ability to overcome biological barriers in vivo.
When magnetic nanoparticles are injected into the body for magnetic resonance imaging, the priority consideration is that degradation of the particles in a biological environment leads to cytotoxicity. Almost all iron oxide-based magnetic nanoparticles that are degraded in a biological environment follow the path of lysosomal digestion. The hydrophilicity of the polymers coating the particles and also the active surface play an important role in the kinetics of the degradation of particles.161 For good cancer imaging, particles should have a prolonged circulation time in blood vessels. The particles should possess enhanced permeation and retention effects regarding tumors so that they can target tumors passively. The particles should not be immediately opsonized and captured by the reticuloendothelial system. All this can be achieved by coating the particles with serum protein, so that effective magnetic resonance imaging is possible.162 The effective imaging and detection of cancer can be elevated by specific localization of magnetic nanoparticles in cancer cells due to the enhanced permeation and retention effect. This can be achieved by introducing specific cancer markers, such as antibodies and glycoproteins, on the surface of magnetic nanoparticles. To immobilize these biomarkers onto nanoparticles, the nanoparticles are initially coated with polymeric materials, which contain functional groups responsible for further coupling with biomarkers.163 The surface charge of the nanoparticles also plays an important role in the opsonization of the particles. Particles with positive or negative charges strongly interact with plasma proteins and are rapidly removed from the bloodstream by macrophages,164 while nanoparticles with a neutral nature, which are coated with hydrophilic polymers such as dextran and polyethylene glycol, avoid interaction with opsonin proteins, such as albumins, fibronectins, and immunoglobulins.165,166 To reduce the particle surface charge, a complex surface should be assembled, where two types of polymer coating, such as polyacrylic acid with polyethylene glycol167 or polyethylene glycol-conjugated branched chitosan,168 are used.
7.3. Targeted drug delivery
Paul Ehrlich, the nobel prize winner in medicine, proposed that if an agent could selectively target a disease-causing organism, then a toxin for that organism could be delivered along with the agent of selectivity. He also proposed a ‘magic bullet,’ which would be able to selectively kill the targeted organism.169 Targeted drug delivery indicates a pharmaceutical dosage form to transport or deliver the drugs to a desired place in the body. Effective, targeted drug delivery systems have four key requirements: to retain, evade, target and release. For example, formulations intended for intravenous administration require efficient drug-loading into some type of delivery vehicle (carrier), so that the drug resides in the circulation in order to reach the sites of the body where it is intended to act. Further drugs should be retained by specific characteristics within intended sites, i.e. targeting. The drug should be released within a specific time for effective function, in response to an external trigger, such as a change in pH, ionic strength, or temperature at the intended site. Ideally, the carrier system, whether it is synthetic or natural, should be able to provide a nontoxic support system that can be manufactured on an industrial scale and can be translated into a cost-effective delivery system. Targeted drug delivery leads to a reduction in the dosages and side effects of drugs, the drug having a uniform effect, and reduced fluctuation in circulating drug levels. Thus, the development of an effective drug delivery system is a great challenge faced by pharmaceutical industries. In this regard, nanoparticles are ideal candidates for drug delivery because they not only act as a nanocarrier for passive targeting but also, their coating makes them useful for active targeting. Magnetic nanoparticles (MNPs) provide dual advantages. Firstly, the drug can be entrapped or loaded on the surface of MNPs as they allows modification of their surfaces by using different surface functionalization strategies. Secondly, the drug can be delivered to tissues by magnetic targeting.170 The morphological difference between normal and cancer tissue surroundings helps the selective penetration of MNPs in the region of the tumor. Usually, solid tumors are characterized by leaky vasculature, which promotes extravasation of MNPs from the blood and their subsequent retention in the tumor interstitium. In contrast, the healthy tissue forms an effective barrier that prevents extravasation of MNPs. A hyperthermia-based controlled drug delivery system can also be a prominent alternative for cancer treatment. In this case, the therapeutic effect can then be triggered by local overheating of the cancer cells via hyperthermia171 and/or upon gated delivery of cancer drugs by the MNPs. For targeted delivery, different kinds of methods are used to attach a drug to the surface of MNPs. For example, a drug molecule can be loaded in magnetic nanoclusters,172 encapsulated in a stimuli-responsive polymer shell,173 covalently linked via a cleavable bond174 or trapped in the magneto liposome.175,176 Various drugs have been successfully incorporated into MNPs, such as paclitaxel, doxorubicin, epirubicin, and methotrexate, on the surface, by using different methods.
7.4. Bacteria detection
Interest in magnetic nanoparticles for detection of bacteria arises due to beneficiary factors, for example, the similar size of nanoparticles, their magnetic behavior, and their ability to attach biomolecules such as proteins and nucleotide probes. It usually takes a long time to detect and capture bacteria at low concentrations by conventional techniques. An important advantage of using magnetic nanoparticles to capture bacteria is the simple separation of bacteria from biological samples using magnets, so that rapid detection is possible. Magnetic nanoparticles have been utilized in the magnetic capture of pathogens in sample preparation, aiding detection and identification, and are promising candidates for microbial decontamination. The wonderful properties of MNPs help to control the location and motion of bacteria with externally applied magnetic fields.177 Since biological media lacks naturally occurring magnetic components, by using magnetic nanoparticles, pathogens can be selectively controlled and detected with excellent specificity and low background noise. Integration of bioconjugated magnetic nanoparticles with different analytical methods has opened new opportunities for bacteria analysis.178 For a specific interaction to occur, magnetic nanoparticles are usually functionalized with biorecognition molecules, such as an antibody, aptamers, bio protein, and carbohydrates, which allow bacteria recognition and targeting.179 Several groups have confirmed superior performances of magnetic nanoparticles (MNPs) and demonstrated exciting and promising applications for capturing bacterial pathogens. For example, Gu et al. showed that E. coli at ultra-low concentrations was effectively captured by vancomycin-conjugated FePt nanoparticles.180,181 Kell et al. also developed a series of vancomycin-modified nanoparticles and employed them in magnetic confinement assays to isolate a variety of bacteria from aqueous solution.182
7.5. Enzyme immobilization
The definition of immobilization is to fix something so that it cannot move. The process of immobilization leads to the physical confinement or localization of the enzyme in a certain region of space with retention of its catalytic activities. By immobilizing an enzyme, some structural changes can occur, thereby changing its properties and activity to some extent. Since enzymes are highly sensitive, their immobilization requires a suitable methodology for keeping or eventually improving their properties, such as stability and activity, thus increasing the possibility of reusing them. The best results have been seen when several magnetic particles and magnetic supports are used. For example, microspheres with various biomaterials encapsulating the magnetic particles and copolymers with magnetic particles were used for enzyme immobilization.183 As a very promising strategy, superparamagnetic nanoparticles based on magnetite (Fe3O4) and maghemite (γ-Fe2O3) have been recently employed. These are used as supporting materials for enzymes, which exhibit characteristics such as a large surface area, mobility and high mass transfer. The bulk material exhibits a direct response to the field, while nanoparticles coherently rotate their single vector to overcome particle anisotropy. In addition, the high surface-to-volume ratio provided by the magnetic nanoparticles favors a high binding capacity and high catalytic specificity of the conjugated enzyme. Magnetic field susceptibility also revealed a mechanism for the efficient recovery of the enzyme complex, thereby preventing enzyme contamination of the final product. Enzyme stability is maximized with nanoscale support with the additional advantages of possible modulation of the catalytic specificity, a lower transfer resistance to solve the diffusion problem and a lower operational cost.184,185
However, since the proteins have been immobilized directly on the surface of such particles, their stability and activity will be greatly influenced by environmental factors, such as the temperature, pH, protease, and immunological responses. Furthermore, pure magnetic particles may not be very useful in practical applications, since they are likely to form a large aggregation, altering their magnetic properties, and may undergo rapid biodegradation when they are directly exposed to the biological system. Therefore, a suitable coating is essential to prevent the occurrence of such limitations.92 In addition, due to anisotropic dipolar attraction, unmodified nanoparticles of ferrites tend to form agglomerates and lose their single-domain associated with specific properties, when subjected to a biological system. It has been shown that aggregation of particles can be prevented and chemical stability can be improved by coating the surface of nanoparticles. Another advantage of coating relates to terminal groups present on the surface, which can be modified with coupling agents so that they covalently bind to multiple ligands specific to the surfaces of these magnetic nanoparticles.186 Ansari and Husain reviewed the potential applications of enzymes immobilized on/in nanomaterials. They described how magnetic nanoparticles were used for bioapplications by immobilized enzymes, which are mentioned in Table 2.187
Table 2 Use of MNPs for bioapplications of immobilized enzymes
Enzyme |
Nanoparticle utilized |
Applications |
α-Amylase |
Cellulose-coated magnetite |
Starch degradation |
Cholesterol oxidase |
Fe3O4 |
Analysis of total cholesterol in serum |
Haloalkane dehalogenase |
Silica coated iron oxide |
Production of fusion proteins containing dehalogenase sequences |
Keratinase |
Fe3O4 |
Synthesis of keratinase |
Laccase |
Chitosan–MNPs |
Bioremediation of environmental pollutants |
Lipase |
Fe3O4 |
Hydrolysis of para-nitrophosphophenol (pNPP) |
Netto and Toma did an interesting review on the use of superparamagnetic particles for the immobilization of enzymes belonging to the categories of hydrolases, oxidoreductases, and transferases. They found that almost all enzymes could be successfully immobilized onto magnetic nanoparticles, which could be used further, and much progress has been achieved in this field.188 A two-step method of polydopamine surface modification and lipase immobilization was illustrated by Ren et al. In the first step, iron oxide MNPs prepared by alkaline co-precipitation of Fe(II) and Fe(III) were initially incubated in an alkaline dopamine solution for several hours to create an adherent polydopamine film on MNPs. In the second step, the enzyme was immobilized by exposure of polydopamine-MNPs to a lipase-containing solution.189 The immobilization of cellulases was successfully carried out by using 1-ethyl-3-[3-dimethylaminopropyl] carbodiimide hydrochloride (EDS) and N-hydroxysuccinimide (NHS), i.e. a EDC–NHS coupling reaction. The enzyme, cellulase, immobilized on MNPs, can be easily separated from the reaction mixture by using a magnetic field. The immobilization technique satisfactorily improves the thermal and pH stability of the enzyme. The immobilized cellulase retains more than ∼64% of its activity after six cycles. The amine-functionalized cobalt ferrite nanoparticles were found to be a reliable medium for covalent immobilization of cellulase enzyme and may be considered for large-scale industrial applications for commercial purposes.190
7.6. Cell labeling and magnetic separation
In biomedicine, it is often advantageous to separate out specific biological entities from their native environment in order to prepare concentrated samples for subsequent analysis or other use. Magnetic separation using biocompatible nanoparticles is one of the ways to achieve this. It is a two-step process, involving (i) the tagging or labeling of the desired biological entity with magnetic material, and (ii) the separating out of these tagged entities via a fluid-based magnetic separation device. Tagging is made possible through chemical modification of the surface of the magnetic nanoparticles. This is usually done by coating MNPs with biocompatible molecules (e.g. dextran, phospholipids, or polyvinyl alcohol) as well as by providing a link between the particle and the target site on a cell or molecule. In addition, coating increases the colloidal stability of the magnetic fluid. Functionalizing magnetic nanoparticles by using antibodies or other biological macromolecules, such as hormones or folic acid, equips them for specific binding towards an enzyme. For example, as antibodies specifically bind to their matching antigen, this provides a highly accurate way to label cells. Magnetic particles coated with immune-specific agents have been successfully bound to red blood cells, golgi vesicles, urological and lung cancer cells, and bacteria.158
Magnetic separation has been successfully applied to many aspects of biomedical and biological research. The increasing use of magnetic carriers in biochemical and molecular biology processes has many advantages, compared to other non-magnetic separation processes.191 It has proven to be a highly sensitive technique for the selection of rare tumor cells from blood, and is especially well suited to the separation of low numbers of target cells.192 For example, this has led to the enhanced detection of malarial parasites in blood samples, either by utilizing the magnetic properties of the parasite193 or through labeling the red blood cells with an immunospecific magnetic fluid.194 The DNA of a sample is amplified and identified through polymerase chain reactions by using this as a pre-processing technology.195 Cell-counting techniques have also been developed. One method estimates the location and number of cells tagged by measuring the magnetic moment of the microsphere tags,196 while another uses a giant magneto-resistive sensor to measure the location of microspheres attached to a surface layered with a bound analyte.197 In another application, magnetic separation has been used in combination with optical sensing to perform ‘magnetic enzyme-linked immunosorbent assays’.198,199 These assays use fluorescent enzymes to optically determine the number of cells labeled with the assay enzymes, where the target material must bind to a solid matrix. To modify the procedure, the magnetic microspheres can be used as a surface for the initial immobilization of the target material and magnetic separation can be used to increase the concentration of the material. The mobility of the magnetic nanoparticles allows a shorter reaction time and a greater volume of the reagent to be used than in standard immunoassays, where the antibody is bound to a plate. Holzinger et al. illustrated a procedure for immunoassay by using MNPs, where the captured analytes are isolated by precipitation of the magnetic nanoparticles in an external magnetic field. The nanoparticles are redispersed as soon as this magnetic field is removed. The analyte-labeled nanoparticles remained immobilized on the surfaces, which are modified with a secondary antibody and thus quantified by a diagnostic magnetic resonance (DMR) technique.200
7.7. Enrichment of DNA
Highly sensitive and selective DNA sensors are a prior requirement regarding genetic engineering. These have a wide role in the diagnosis of genetic diseases, detection of infectious agents, gene therapy, and identification in forensic and environmental cases. In recent years, many studies have been devoted to developing DNA sensors due to the need for simplicity, specificity, exceptional sensitivity and selectivity in the detection of specific genes. Some conventional techniques, such as PCR and real-time PCR, have the ability of amplification from small amounts of DNA into readable quantities and are applied mostly in the fields of biomedicine. However, these techniques demand more time, sample preparation, high-tech equipped apparatus and well-educated operators. Nano-bio sensors have opened a perspective to overcome most of the disadvantages of conventional detection methods for applications in agriculture, clinics, drugs, environment, and food. Magnetic nanoparticle-DNA interactions can open up new possibilities in various biomedical applications, which are based on the fundamental properties of DNA and magnetic nanoparticles.
Nucleic acid-MNP assembly holds great potential for genetic tests and nanomedicine at the molecular level. For successful achievement of genetically engineered biomedical applications, a prior requirement is the enrichment of DNA, which can be effectively achieved by using magnetic nanoparticles and specifically tailoring them for DNA.200 For example, both modified and unmodified cobalt ferrite nanoparticles are suitable for the isolation and purification of genomic DNA. It is possible to achieve DNA adsorption and desorption on modified cobalt ferrite surfaces, which are similar to those for silica-based supports.201 Some key factors play an important role in the enrichment of DNA by using magnetic nanoparticles. These are the types of interaction between DNA and magnetic nanoparticles, the surface modification of magnetic nanoparticles, functional groups, and the mode of adsorption of DNA on the surface of magnetic nanoparticles. By altering these factors, many researchers have reported potential nano-bio applications, where nucleic materials are enriched in such a fashion so that they can possess integrity and the potentiality for a variety of applications. From various reported applications, some are listed in Table 3.
Table 3 List of nano-bio applications by enrichment of nucleic acid using MNPsa
MNPs |
Surface modifier |
Functional group |
Nucleic acid |
Applications |
Ref. |
Note. TEOS stands for tetraethylorthosilicate; CEPA stands for carboxyl ethyl phosphonic acid; HBV stands for hepatitis B virus; APTMS stands for (3-aminopropyl) trimethoxysilane; MRI stands for magnetic resonance imaging; PEI stands for polyethylenimine; ODN stands for antisense oligo (deoxy) nucleotides; and PAA stands for poly(acrylic acid). |
Fe3O4 |
TEOS |
Si–O |
Genomic DNA of HBV |
Pathogen detection |
202 |
Fe3O4 |
2-CEPA |
–COOH |
mRNA, pDNA |
Isolation and extraction |
203 |
Fe3O4 |
Chitosan |
–NH2 |
Plasmid DNA |
DNA isolation |
204 |
Fe3O4 |
TEOS and APTMS |
–NH2 |
Human genomic DNA |
DNA separation |
205 |
Fe2O3, CoFe2O4 |
2-Pyrrolidone |
— |
λ Phage DNA |
MRI contrast agent |
206 |
Fe3O4 |
PAA, PEI |
–NH2 |
Plasmid DNA |
Gene therapy |
207 |
Fe3O4 |
Hemin |
ODN |
Poly-nucleotide |
Cell cycle monitoring with MRI |
208 and 209 |
8. Conclusion
Magnetic nanoparticles gained much interest due to their ability to be manipulated upon application of a magnetic field. MNPs are now being exposed extensively for multiple functions that are much needed for biological applications. Factors such as biocompatibility, toxicity, in vivo and in vitro targeting efficiency and long-term stability of MNPs, should always be addressed with respect to their use in bioapplications. The increasing demands and versatile requirements imposed on MNPs intended for bioapplications require close control over the size, structure, and surface properties of MNPs. The key requirements for MNPs are colloidal stability under physiological conditions and tunable surfaces, which can only be met through surface modification and are of pivotal importance, especially for bioapplications. Hence, there is a great demand for efficient surface functionalization. The design of MNPs with multifunctional coatings for bioapplications is a rapidly progressing area and is being researched accordingly. Surface functionalization of MNPs allows them to attach to various biomolecules, making them a promising candidate for bioapplications. Different chemical modalities (synthetic and natural) have been studied and found to be efficient for the functionalization of MNPs.
However, several important aspects of the surface functionalization of MNPs need to be addressed when translating from experimental success to actual practice for bioapplications. On the synthetic front, the surface functionalities should be fabricated to impart biomimetic properties, such as stability in complex biological media, nontoxicity, and specificity for particular biomolecules, cells, and tissues. Other properties of MNPs, such as efficiency, stability, safety, and economy, as well as multifunctionality, should be comprehensively considered. There is also an immense requirement for different varieties of surface-modifying materials. More materials and techniques should be developed and exposed with respect to the functionalization of MNPs, since the type of functionalization has a dramatic effect on the end use of the functionalized MNPs.
Acknowledgements
R. A. B. is thankful to D. Y. Patil University for financial assistance. N. D. T. is thankful for financial support from the Irish Research Council under the Government of Ireland Postdoctoral Fellowship-2015, Grant No. GOIPD/2015/320.
References
- B. E. Kashevsky, V. E. Agabekov, S. B. Kashevsky, K. A. Kekalo, E. Y. Manina, I. V. Prokhorov and V. S. Ulashchik, Particuology, 2008, 6, 322–333 CrossRef CAS.
- H. Zhou, W. Qian, F. M. Uckun, L. Wang, Y. A. Wang, H. Chen, D. Kooby, Q. Yu, M. Lipowska, C. A. Staley, H. Mao and L. Yang, ACS Nano, 2015, 9, 7976–7991 CrossRef CAS PubMed.
- K. McNamara and S. A. M. Tofail, Phys. Chem. Chem. Phys., 2015, 17, 27981–27995 RSC.
- D. T. Nguyen and K.-S. Kim, Korean J. Chem. Eng., 2014, 31, 1289–1305 CrossRef CAS.
- D. Yoo, J.-H. Lee, T.-H. Shin and J. Cheon, Acc. Chem. Res., 2011, 44, 863–874 CrossRef CAS PubMed.
- R. A. Bohara, N. D. Thorat, H. M. Yadav and S. H. Pawar, New J. Chem., 2014, 38, 2979 RSC.
- B. Derjaguin and L. Landau, Prog. Surf. Sci., 1993, 43, 30–59 CrossRef.
- E. J. W. Verwey, J. T. G. Overbeek and J. T. G. Overbeek, Theory of the Stability of Lyophobic Colloids, Courier Corporation, 1999 Search PubMed.
- U. Schwertmann and R. M. Cornell, Iron Oxides in the Laboratory: Preparation and Characterization, John Wiley & Sons, 2008 Search PubMed.
- D. Napper, J. Colloid Interface Sci., 1970, 32, 106–114 CrossRef CAS.
- G. Fritz, V. Schädler, N. Willenbacher and N. J. Wagner, Langmuir, 2002, 18, 6381–6390 CrossRef CAS.
- J. L. Ortega-Vinuesa, A. Martín-Rodríguez and R. Hidalgo-Álvarez, J. Colloid Interface Sci., 1996, 184, 259–267 CrossRef CAS PubMed.
- M. Kobayashi, M. Skarba, P. Galletto, D. Cakara and M. Borkovec, J. Colloid Interface Sci., 2005, 292, 139–147 CrossRef CAS PubMed.
- A. V. Bychkova, O. N. Sorokina, M. A. Rosenfeld and A. L. Kovarski, Russ. Chem. Rev., 2012, 81, 1026–1050 CrossRef.
- S. Chandra, K. C. Barick and D. Bahadur, Adv. Drug Delivery Rev., 2011, 63, 1267–1281 CrossRef CAS PubMed.
- J. Chen, Z. Guo, H.-B. Wang, M. Gong, X.-K. Kong, P. Xia and Q.-W. Chen, Biomaterials, 2013, 34, 571–581 CrossRef CAS PubMed.
- Y. Chen, Q. Yin, X. Ji, S. Zhang, H. Chen, Y. Zheng, Y. Sun, H. Qu, Z. Wang, Y. Li, X. Wang, K. Zhang, L. Zhang and J. Shi, Biomaterials, 2012, 33, 7126–7137 CrossRef CAS PubMed.
- R. Hao, R. Xing, Z. Xu, Y. Hou, S. Gao and S. Sun, Adv. Mater., 2010, 22, 2729–2742 CrossRef CAS PubMed.
- D. S. Nikam, S. V. Jadhav, V. M. Khot, R. A. Bohara, S. S. Mali, C. H. Hong and S. H. Pawar, RSC Adv., 2015, 5, 2338–2345 RSC.
- W.-C. Wang, K.-G. Neoh and E.-T. Kang, Macromol. Rapid Commun., 2006, 27, 1665–1669 CrossRef CAS.
- P. F. Du, L. X. Song, J. Xiong, Z. Q. Xi, J. J. Chen, L. H. Gao and N. Y. Wang, J. Nanosci. Nanotechnol., 2011, 11, 7723–7728 CrossRef CAS PubMed.
- H. Bin Na, I. S. Lee, H. Seo, Y. Il Park, J. H. Lee, S.-W. Kim and T. Hyeon, Chem. Commun., 2007, 5167–5169 Search PubMed.
- K. Cheng, S. Peng, C. Xu and S. Sun, J. Am. Chem. Soc., 2009, 131, 10637–10644 CrossRef CAS PubMed.
- S. E. McNeil, Wiley Interdiscip. Rev.: Nanomed. Nanobiotechnol., 2009, 1, 264–271 CrossRef CAS PubMed.
- H. Xu, F. Yan, E. E. Monson and R. Kopelman, J. Biomed. Mater. Res., Part A, 2003, 66, 870–879 CrossRef PubMed.
- N. T. K. Thanh and L. A. W. Green, Nano Today, 2010, 5, 213–230 CrossRef CAS.
- X. Chu, J. Yu and Y.-L. Hou, Chin. Phys. B, 2015, 24, 014704 CrossRef.
- R. M. Patil, N. D. Thorat, P. B. Shete, S. V. Otari, B. M. Tiwale and S. H. Pawar, Mater. Sci. Eng., C, 2016, 59, 702–709 CrossRef CAS PubMed.
- Y. Sahoo, A. Goodarzi, M. T. Swihart, T. Y. Ohulchanskyy, N. Kaur, E. P. Furlani and P. N. Prasad, J. Phys. Chem. B, 2005, 109, 3879–3885 CrossRef CAS PubMed.
- S. Laurent, D. Forge, M. Port, A. Roch, C. Robic, L. Vander Elst and R. N. Muller, Chem. Rev., 2008, 108, 2064–2110 CrossRef CAS PubMed.
- D. Portet, B. Denizot, E. Rump, F. Hindre, J.-J. Le Jeune and P. Jallet, Drug Dev. Res., 2001, 54, 173–181 CrossRef CAS.
- D. I. Kreller, G. Gibson, W. Novak, G. W. Van Loon and J. H. Horton, Colloids Surf., A, 2003, 212, 249–264 CrossRef CAS.
- M. Czakler, C. Artner and U. Schubert, Eur. J. Inorg. Chem., 2014, 2014, 2038–2045 CrossRef CAS PubMed.
- Y. Sahoo, H. Pizem, T. Fried, D. Golodnitsky, L. Burstein, C. N. Sukenik and G. Markovich, Langmuir, 2001, 17, 7907–7911 CrossRef CAS.
- M. I. Tejedor-Tejedor and M. A. Anderson, Langmuir, 1990, 6, 602–611 CrossRef CAS.
- P. Persson, N. Nilsson and S. Sjöberg, J. Colloid Interface Sci., 1996, 177, 263–275 CrossRef CAS PubMed.
- C. Yee, G. Kataby, A. Ulman, T. Prozorov, H. White, A. King, M. Rafailovich, J. Sokolov and A. Gedanken, Langmuir, 1999, 15, 7111–7115 CrossRef CAS.
- S. Mohapatra, N. Pramanik, S. K. Ghosh and P. Pramanik, J. Nanosci. Nanotechnol., 2006, 6, 823–829 CrossRef CAS PubMed.
- D. Roberts, W. L. Zhu, C. M. Frommen and Z. Rosenzweig, J. Appl. Phys., 2000, 87, 6208 CrossRef CAS.
- M. Gunay, A. Baykal and H. Sozeri, J. Supercond. Novel Magn., 2012, 25, 2415–2420 CrossRef.
- M. Longmire, P. L. Choyke and H. Kobayashi, Nanomedicine, 2008, 3, 703–717 CrossRef CAS PubMed.
- K. C. Barick, M. Aslam, Y.-P. Lin, D. Bahadur, P. V. Prasad and V. P. Dravid, J. Mater. Chem., 2009, 19, 7023 RSC.
- K. C. Barick, M. Aslam, P. V. Prasad, V. P. Dravid and D. Bahadur, J. Magn. Magn. Mater., 2009, 321, 1529–1532 CrossRef CAS PubMed.
- Y. Lai, W. Yin, J. Liu, R. Xi and J. Zhan, Nanoscale Res. Lett., 2009, 5, 302–307 CrossRef PubMed.
- J. Xie, K. Chen, H.-Y. Lee, C. Xu, A. R. Hsu, S. Peng, X. Chen and S. Sun, J. Am. Chem. Soc., 2008, 130, 7542–7543 CrossRef CAS PubMed.
- C. R. A. Valois, J. M. Braz, E. S. Nunes, M. A. R. Vinolo, E. C. D. Lima, R. Curi, W. M. Kuebler and R. B. Azevedo, Biomaterials, 2010, 31, 366–374 CrossRef CAS PubMed.
- L. Maurizi, H. Bisht, F. Bouyer and N. Millot, Langmuir, 2009, 25, 8857–8859 CrossRef CAS PubMed.
- W. Wu, Q. He and C. Jiang, Nanoscale Res. Lett., 2008, 3, 397–415 CrossRef CAS PubMed.
- R. M. Patil, P. B. Shete, N. D. Thorat, S. V. Otari, K. C. Barick, A. Prasad, R. S. Ningthoujam, B. M. Tiwale and S. H. Pawar, J. Magn. Magn. Mater., 2014, 355, 22–30 CrossRef CAS.
- C. Zhang, B. Wängler, B. Morgenstern, H. Zentgraf, M. Eisenhut, H. Untenecker, R. Krüger, R. Huss, C. Seliger, W. Semmler and F. Kiessling, Langmuir, 2007, 23, 1427–1434 CrossRef CAS PubMed.
- E. Amstad, T. Gillich, I. Bilecka, M. Textor and E. Reimhult, Nano Lett., 2009, 9, 4042–4048 CrossRef CAS PubMed.
- L. F. Gamarra, G. E. S. Brito, W. M. Pontuschka, E. Amaro, A. H. C. Parma and G. F. Goya, J. Magn. Magn. Mater., 2005, 289, 439–441 CrossRef CAS.
- K. M. Lee, S.-G. Kim, W.-S. Kim and S. S. Kim, Korean J. Chem. Eng., 2002, 19, 480–485 CrossRef CAS.
- M. Lewin, N. Carlesso, C. H. Tung, X. W. Tang, D. Cory, D. T. Scadden and R. Weissleder, Nat. Biotechnol., 2000, 18, 410–414 CrossRef CAS PubMed.
- H. Pardoe, W. Chua-anusorn, T. G. St Pierre and J. Dobson, J. Magn. Magn. Mater., 2001, 225, 41–46 CrossRef CAS.
- Handbook for Magnetic materials, ed. K. H. J. Buschow, Elsevier, 2006, vol. 6 Search PubMed.
- C. W. Jung, Magn. Reson. Imaging, 1995, 13, 675–691 CrossRef CAS PubMed.
- C. Fournier, M. Leonard, I. Le Coq-Leonard and E. Dellacherie, Langmuir, 1995, 11, 2344–2347 CrossRef CAS.
- P. B. Shete, R. M. Patil, N. D. Thorat, A. Prasad, R. S. Ningthoujam, S. J. Ghosh and S. H. Pawar, Appl. Surf. Sci., 2014, 288, 149–157 CrossRef CAS.
- S. R. Bhattarai, K. C. R. Bahadur, S. Aryal, M. S. Khil and H. Y. Kim, Carbohydr. Polym., 2007, 69, 467–477 CrossRef CAS.
- E. H. Kim, Y. Ahn and H. S. Lee, J. Alloys Compd., 2007, 434–435, 633–636 CrossRef CAS.
- P. Sipos, J. Inorg. Biochem., 2003, 95, 55–63 CrossRef CAS PubMed.
- J. Zhi, Y. Wang, Y. Lu, J. Ma and G. Luo, React. Funct. Polym., 2006, 66, 1552–1558 CrossRef CAS.
- H. S. Lee, E. Hee Kim, H. Shao and B. Kook Kwak, J. Magn. Magn. Mater., 2005, 293, 102–105 CrossRef CAS.
- E. Hee Kim, H. Sook Lee, B. Kook Kwak and B.-K. Kim, J. Magn. Magn. Mater., 2005, 289, 328–330 CrossRef.
- L. Sandiford, A. Phinikaridou, A. Protti, L. K. Meszaros, X. Cui, Y. Yan, G. Frodsham, P. A. Williamson, N. Gaddum, R. M. Botnar, P. J. Blower, M. A. Green and R. T. M. de Rosales, ACS Nano, 2013, 7, 500–512 CrossRef CAS PubMed.
- J. Xie, C. Xu, N. Kohler, Y. Hou and S. Sun, Adv. Mater., 2007, 19, 3163–3166 CrossRef CAS.
- H. W. Kang, L. Josephson, A. Petrovsky, R. Weissleder and A. Bogdanov, Bioconjugate Chem., 2002, 13, 122–127 CrossRef CAS PubMed.
- C. Fang, N. Bhattarai, C. Sun and M. Zhang, Small, 2009, 5, 1637–1641 CrossRef CAS PubMed.
- M. Sairam, B. V. K. Naidu, S. K. Nataraj, B. Sreedhar and T. M. Aminabhavi, J. Membr. Sci., 2006, 283, 65–73 CrossRef CAS.
- B. Schöpf, T. Neuberger, K. Schulze, A. Petri, M. Chastellain, M. Hofmann, H. Hofmann and B. von Rechenberg, J. Magn. Magn. Mater., 2005, 293, 411–418 CrossRef.
- B. Xue and Y. Sun, J. Chromatogr. A, 2001, 921, 109–119 CrossRef CAS PubMed.
- H. Yokoi and T. Kantoh, Bull. Chem. Soc. Jpn., 1993, 66, 1536–1541 CrossRef CAS.
- C. Albornoz and S. E. Jacobo, J. Magn. Magn. Mater., 2006, 305, 12–15 CrossRef CAS.
- J. Lee, T. Isobe and M. Senna, J. Colloid Interface Sci., 1996, 177, 490–494 CrossRef CAS.
- M. Chastellian, A. Petri and H. Hofman, J. Colloid Interface Sci., 2004, 278, 353 CrossRef PubMed.
- P. V. Finotelli, M. A. Morales, M. H. Rocha-Leão, E. M. Baggio-Saitovitch and A. M. Rossi, Mater. Sci. Eng., C, 2004, 24, 625–629 CrossRef.
- E. Kroll, F. M. Winnik and R. F. Ziolo, Chem. Mater., 1996, 8, 1594–1596 CrossRef CAS.
- F. Llanes, D. H. Ryan and R. H. Marchessault, Int. J. Biol. Macromol., 2000, 27, 35–40 CrossRef CAS PubMed.
- Y. Nishio, A. Yamada, K. Ezaki, Y. Miyashita, H. Furukawa and K. Horie, Polymer, 2004, 45, 7129–7136 CrossRef CAS.
- H. L. Ma, Y. F. Xu, X. R. Qi, Y. Maitani and T. Nagai, Int. J. Pharm., 2008, 354, 217–226 CrossRef CAS PubMed.
- M. A. Morales, P. V. Finotelli, J. A. H. Coaquira, M. H. M. Rocha-Leão, C. Diaz-Aguila, E. M. Baggio-Saitovitch and A. Rossi, Mater. Sci. Eng., C, 2008, 28, 253–257 CrossRef CAS.
- O. Veiseh, J. W. Gunn and M. Zhang, Adv. Drug Delivery Rev., 2010, 62, 284–304 CrossRef CAS PubMed.
- M.-J. Kim, D.-H. Jang, Y.-I. Lee, H. S. Jung, H.-J. Lee and Y.-H. Choa, J. Nanosci. Nanotechnol., 2011, 11, 889–893 CrossRef CAS PubMed.
- W. J. M. Mulder, G. J. Strijkers, G. A. F. van Tilborg, A. W. Griffioen and K. Nicolay, NMR Biomed., 2006, 19, 142–164 CrossRef CAS PubMed.
- D.-H. Kim, E. A. Vitol, J. Liu, S. Balasubramanian, D. J. Gosztola, E. E. Cohen, V. Novosad and E. A. Rozhkova, Langmuir, 2013, 29, 7425–7432 CrossRef CAS PubMed.
- N. D. Thorat, R. M. Patil, V. M. Khot, A. B. Salunkhe, A. I. Prasad, K. C. Barick, R. S. Ningthoujam and S. H. Pawar, New J. Chem., 2013, 37, 2733 RSC.
- N. Erathodiyil and J. Y. Ying, Acc. Chem. Res., 2011, 44, 925–935 CrossRef CAS PubMed.
- S. Nigam, K. C. Barick and D. Bahadur, J. Magn. Magn. Mater., 2011, 323, 237–243 CrossRef CAS.
- Y. Sun, L. Duan, Z. Guo, Y. DuanMu, M. Ma, L. Xu, Y. Zhang and N. Gu, J. Magn. Magn. Mater., 2005, 285, 65–70 CrossRef CAS.
- S. H. Im, T. Herricks, Y. T. Lee and Y. Xia, Chem. Phys. Lett., 2005, 401, 19–23 CrossRef CAS.
- H.-H. Yang, S.-Q. Zhang, X.-L. Chen, Z.-X. Zhuang, J.-G. Xu and X.-R. Wang, Anal. Chem., 2004, 76, 1316–1321 CrossRef CAS PubMed.
- P. Tartaj, T. González-Carreño and C. J. Serna, Adv. Mater., 2001, 13, 1620–1624 CrossRef CAS.
- P. Tartaj, T. González-Carreño and C. J. Serna, Langmuir, 2002, 18, 4556–4558 CrossRef CAS.
- Q. Liu, Z. Xu, J. A. Finch and R. Egerton, Chem. Mater., 1998, 10, 3936–3940 CrossRef CAS.
- A. Ulman, An Introduction to Ultrathin Organic Films: From Langmuir–Blodgett to Self–Assembly, Elsevier Science, 2013 Search PubMed.
- L. Wang, J. Luo, Q. Fan, M. Suzuki, I. S. Suzuki, M. H. Engelhard, Y. Lin, N. Kim, J. Q. Wang and C.-J. Zhong, J. Phys. Chem. B, 2005, 109, 21593–21601 CrossRef CAS PubMed.
- J. L. Lyon, D. A. Fleming, M. B. Stone, P. Schiffer and M. E. Williams, Nano Lett., 2004, 4, 719–723 CrossRef CAS.
- Z. Xu, Y. Hou and S. Sun, J. Am. Chem. Soc., 2007, 129, 8698–8699 CrossRef CAS PubMed.
- J. Lin, W. Zhou, A. Kumbhar, J. Wiemann, J. Fang, E. E. Carpenter and C. J. O'Connor, J. Solid State Chem., 2001, 159, 26–31 CrossRef CAS.
- M. Ramasamy, S. S. Lee, D. K. Yi and K. Kim, J. Mater. Chem. B, 2014, 2, 981 RSC.
- P. Gong, H. Li, X. He, K. Wang, J. Hu, W. Tan, S. Zhang and X. Yang, Nanotechnology, 2007, 18, 285604 CrossRef.
- J. Jiang, H. Gu, H. Shao, E. Devlin, G. C. Papaefthymiou and J. Y. Ying, Adv. Mater., 2008, 20, 4403–4407 CrossRef CAS.
- D. Tang, R. Yuan and Y. Chai, J. Phys. Chem. B, 2006, 110, 11640–11646 CrossRef CAS PubMed.
- S. V. Otari, R. M. Patil, S. J. Ghosh, N. D. Thorat and S. H. Pawar, Spectrochim. Acta, Part A, 2015, 136, 1175–1180 CrossRef CAS PubMed Pt B.
- S. V. Otari, H. M. Yadav, N. D. Thorat, R. M. Patil, J. K. Lee and S. H. Pawar, Mater. Lett., 2016, 167, 179–182 CrossRef CAS.
- X. Zhu, J. Zhou, M. Chen, M. Shi, W. Feng and F. Li, Biomaterials, 2012, 33, 4618–4627 CrossRef CAS PubMed.
- R. Y. Hong, S. Z. Zhang, G. Q. Di, H. Z. Li, Y. Zheng, J. Ding and D. G. Wei, Mater. Res. Bull., 2008, 43, 2457–2468 CrossRef CAS.
- Y. Jun, J. Choi and J. Cheon, Chem. Commun., 2007, 1203–1214 RSC.
- J. Cheon, J.-I. Park, J. Choi, Y. Jun, S. Kim, M. G. Kim, Y.-M. Kim and Y. J. Kim, Proc. Natl. Acad. Sci. U. S. A., 2006, 103, 3023–3027 CrossRef CAS PubMed.
- I. Medintz, Nat. Mater., 2006, 5, 842 CrossRef CAS PubMed.
- K. E. Sapsford, W. R. Algar, L. Berti, K. B. Gemmill, B. J. Casey, E. Oh, M. H. Stewart and I. L. Medintz, Chem. Rev., 2013, 113, 1904–2074 CrossRef CAS PubMed.
- B. Steitz, H. Hofmann, S. W. Kamau, P. O. Hassa, M. O. Hottiger, B. von Rechenberg, M. Hofmann-Amtenbrink and A. Petri-Fink, J. Magn. Magn. Mater., 2007, 311, 300–305 CrossRef CAS.
- M. Chorny, B. Polyak, I. S. Alferiev, K. Walsh, G. Friedman and R. J. Levy, FASEB J., 2007, 21, 2510–2519 CrossRef CAS PubMed.
- I.-K. Park, C.-P. Ng, J. Wang, B. Chu, C. Yuan, S. Zhang and S. H. Pun, Biomaterials, 2008, 29, 724–732 CrossRef CAS PubMed.
- S. C. McBain, H. H. P. Yiu, A. El Haj and J. Dobson, J. Mater. Chem., 2007, 17, 2561 RSC.
- Y. Liu, B. Zhang and B. Yan, Int. J. Mol. Sci., 2011, 12, 4395–4413 CrossRef CAS PubMed.
- J. Gunn, H. Wallen, O. Veiseh, C. Sun, C. Fang, J. Cao, C. Yee and M. Zhang, Small, 2008, 4, 712–715 CrossRef CAS PubMed.
- R. A. Sperling and W. J. Parak, Philos. Trans. R. Soc., A, 2010, 368, 1333–1383 CrossRef CAS PubMed.
- S. Mohapatra, S. R. Rout, S. Maiti, T. K. Maiti and A. B. Panda, J. Mater. Chem., 2011, 21, 9185 RSC.
- J. Jordan, C. S. S. R. Kumar and C. Theegala, J. Mol. Catal. B: Enzym., 2011, 68, 139–146 CrossRef CAS.
- S. Kumar, A. K. Jana, M. Maiti and I. Dhamija, J. Nanopart. Res., 2014, 16, 2233 CrossRef.
- A. A. Bogdanov, A. L. Klibanov and V. P. Torchilin, FEBS Lett., 1988, 231, 381–384 CrossRef CAS PubMed.
- M. Brinkley, Bioconjugate Chem., 1992, 3, 2–13 CrossRef CAS PubMed.
- B. Dubertret, P. Skourides, D. J. Norris, V. Noireaux, A. H. Brivanlou and A. Libchaber, Science, 2002, 298, 1759–1762 CrossRef CAS PubMed.
- H. C. Kolb, M. G. Finn and K. B. Sharpless, Angew. Chem., Int. Ed. Engl., 2001, 40, 2004–2021 CrossRef CAS.
- M. A. White, J. A. Johnson, J. T. Koberstein and N. J. Turro, J. Am. Chem. Soc., 2007, 129, 4504 CrossRef CAS.
- N. Li and W. H. Binder, J. Mater. Chem., 2011, 21, 16717 RSC.
- P. K. Sneed, P. R. Stauffer, M. W. McDermott, C. J. Diederich, K. R. Lamborn, M. D. Prados, S. Chang, K. A. Weaver, L. Spry, M. K. Malec, S. A. Lamb, B. Voss, R. L. Davis, W. M. Wara, D. A. Larson, T. L. Phillips and P. H. Gutin, Int. J. Radiat. Oncol., Biol., Phys., 1998, 40, 287–295 CrossRef CAS.
- P. Wust, B. Hildebrandt, G. Sreenivasa, B. Rau, J. Gellermann, H. Riess, R. Felix and P. Schlag, Lancet Oncol., 2002, 3, 487–497 CrossRef CAS PubMed.
- B. Thiesen and A. Jordan, Int. J. Hyperthermia, 2008, 24, 467–474 CrossRef CAS PubMed.
- R. K. Gilchrist, R. Medal, W. D. Shorey, R. C. Hanselman, J. C. Parrott and C. B. Taylor, Ann. Surg., 1957, 146, 596–606 CrossRef CAS PubMed.
- W. J. Curran, C. B. Scott, J. Horton, J. S. Nelson, A. S. Weinstein, A. J. Fischbach, C. H. Chang, M. Rotman, S. O. Asbell, R. E. Krisch and D. F. Nelson, JNCI, J. Natl. Cancer Inst., 1993, 85, 704–710 CrossRef PubMed.
- C. S. Brazel, Pharm. Res., 2009, 26, 644–656 CrossRef CAS PubMed.
- X. Li Liu, E. S. Guang Choo, A. S. Ahmed, L. Y. Zhao, Y. Yang, R. V. Ramanujan, J. Xue, D. D. Fan, H. M. Fan and J. Ding, J. Mater. Chem. B, 2014, 2, 120–128 RSC.
- N. D. Thorat, K. P. Shinde, S. H. Pawar, K. C. Barick, C. A. Betty and R. S. Ningthoujam, Dalton Trans., 2012, 41, 3060–3071 RSC.
- T. Kobayashi, Biotechnol. J., 2011, 6, 1342–1347 CrossRef CAS PubMed.
- R. A. Bohara, H. M. Yadav, N. D. Thorat, S. S. Mali, C. K. Hong, S. G. Nanaware and S. H. Pawar, J. Magn. Magn. Mater., 2015, 378, 397–401 CrossRef CAS.
- N. K. Prasad, K. Rathinasamy, D. Panda and D. Bahadur, J. Mater. Chem., 2007, 17, 5042 RSC.
- N. K. Prasad, K. Rathinasamy, D. Panda and D. Bahadur, J. Biomed. Mater. Res., Part B, 2008, 85, 409–416 CrossRef CAS PubMed.
- N. D. Thorat, V. M. Khot, A. B. Salunkhe, R. S. Ningthoujam and S. H. Pawar, Colloids Surf., B, 2013, 104, 40–47 CrossRef CAS PubMed.
- N. D. Thorat, S. V. Otari, R. A. Bohara, H. M. Yadav, V. M. Khot, A. B. Salunkhe, M. R. Phadatare, A. I. Prasad, R. S. Ningthoujam and S. H. Pawar, Mater. Sci. Eng., C, 2014, 42, 637–646 CrossRef CAS PubMed.
- N. D. Thorat, S. V. Otari, R. M. Patil, R. A. Bohara, H. M. Yadav, V. B. Koli, A. K. Chaurasia and R. S. Ningthoujam, Dalton Trans., 2014, 43, 17343–17351 RSC.
- R. A. Bohara, N. D. Thorat, A. K. Chaurasia and S. H. Pawar, RSC Adv., 2015, 5, 47225–47234 RSC.
- K. Hayashi, K. Ono, H. Suzuki, M. Sawada, M. Moriya, W. Sakamoto and T. Yogo, ACS Appl. Mater. Interfaces, 2010, 2, 1903–1911 CAS.
- S. Balivada, R. S. Rachakatla, H. Wang, T. N. Samarakoon, R. K. Dani, M. Pyle, F. O. Kroh, B. Walker, X. Leaym, O. B. Koper, M. Tamura, V. Chikan, S. H. Bossmann and D. L. Troyer, BMC Cancer, 2010, 10, 119 CrossRef PubMed.
- S. A. Meenach, J. Z. Hilt and K. W. Anderson, Acta Biomater., 2010, 6, 1039–1046 CrossRef CAS PubMed.
- M. K. Jaiswal, R. Banerjee, P. Pradhan and D. Bahadur, Colloids Surf., B, 2010, 81, 185–194 CrossRef CAS PubMed.
- N. D. Thorat, S. V. Otari, R. M. Patil, V. M. Khot, A. I. Prasad, R. S. Ningthoujam and S. H. Pawar, Colloids Surf., B, 2013, 111, 264–269 CrossRef CAS PubMed.
- N. K. Prasad, L. Hardel, E. Duguet and D. Bahadur, J. Magn. Magn. Mater., 2009, 321, 1490–1492 CrossRef CAS.
- D.-H. Kim, D. E. Nikles, D. T. Johnson and C. S. Brazel, J. Magn. Magn. Mater., 2008, 320, 2390–2396 CrossRef CAS.
- N. K. Prasad, D. Panda, S. Singh, M. D. Mukadam, S. M. Yusuf and D. Bahadur, J. Appl. Phys., 2005, 97, 10Q903 CrossRef.
- N. K. Prasad, D. Panda, S. Singh and D. Bahadur, IEEE Trans. Magn., 2005, 41, 4099–4101 CrossRef CAS.
- C. R. Thomas, D. P. Ferris, J.-H. Lee, E. Choi, M. H. Cho, E. S. Kim, J. F. Stoddart, J.-S. Shin, J. Cheon and J. I. Zink, J. Am. Chem. Soc., 2010, 132, 10623–10625 CrossRef CAS PubMed.
- F. M. Martín-Saavedra, E. Ruíz-Hernández, A. Boré, D. Arcos, M. Vallet-Regí and N. Vilaboa, Acta Biomater., 2010, 6, 4522–4531 CrossRef PubMed.
- H. M. Joshi, Y. P. Lin, M. Aslam, P. V. Prasad, E. A. Schultz-Sikma, R. Edelman, T. Meade and V. P. Dravid, J. Phys. Chem. C, 2009, 113, 17761–17767 CAS.
- X. Cai, X. Jia, W. Gao, K. Zhang, M. Ma, S. Wang, Y. Zheng, J. Shi and H. Chen, Adv. Funct. Mater., 2015, 25, 2628 CrossRef.
- Q. A. Pankhurst, J. Connolly, S. K. Jones and J. Dobson, J. Phys. D: Appl. Phys., 2003, 36, R167–R181 CrossRef CAS.
- R. Qiao, C. Yang and M. Gao, J. Mater. Chem., 2009, 19, 6274 RSC.
- H. Wu, G. Liu, X. Wang, J. Zhang, Y. Chen, J. Shi, H. Yang, H. Hu and S. Yang, Acta Biomater., 2011, 7, 3496–3504 CrossRef CAS PubMed.
- L. Lartigue, D. Alloyeau, J. Kolosnjaj-Tabi, Y. Javed, P. Guardia, A. Riedinger, C. Péchoux, T. Pellegrino, C. Wilhelm and F. Gazeau, ACS Nano, 2013, 7, 3939–3952 CrossRef CAS PubMed.
- M. Elsabahy and K. L. Wooley, Chem. Soc. Rev., 2012, 41, 2545–2561 RSC.
- T. Tanaka, S. Shiramoto, M. Miyashita, Y. Fujishima and Y. Kaneo, Int. J. Pharm., 2004, 277, 39–61 CrossRef CAS PubMed.
- M. A. Dobrovolskaia and S. E. McNeil, Nat. Nanotechnol., 2007, 2, 469–478 CrossRef CAS PubMed.
- R. Y. Hong, B. Feng, L. L. Chen, G. H. Liu, H. Z. Li, Y. Zheng and D. G. Wei, Biochem. Eng. J., 2008, 42, 290–300 CrossRef CAS.
- M. Roser, D. Fischer and T. Kissel, Eur. J. Pharm. Biopharm., 1998, 46, 255–263 CrossRef CAS PubMed.
- N. Pothayee, S. Balasubramaniam, N. Pothayee, N. Jain, N. Hu, Y. Lin, R. M. Davis, N. Sriranganathan, A. P. Koretsky and J. S. Riffle, J. Mater. Chem. B, 2013, 1, 1142–1149 RSC.
- O. Veiseh, C. Sun, C. Fang, N. Bhattarai, J. Gunn, F. Kievit, K. Du, B. Pullar, D. Lee, R. G. Ellenbogen, J. Olson and M. Zhang, Cancer Res., 2009, 69, 6200–6207 CrossRef CAS PubMed.
- M. Arruebo, R. Fernández-Pacheco, M. R. Ibarra and J. Santamaría, Nano Today, 2007, 2, 22–32 CrossRef.
- B. Chertok, B. A. Moffat, A. E. David, F. Yu, C. Bergemann, B. D. Ross and V. C. Yang, Biomaterials, 2008, 29, 487–496 CrossRef CAS PubMed.
- P. Guardia, R. Di Corato, L. Lartigue, C. Wilhelm, A. Espinosa, M. Garcia-Hernandez, F. Gazeau, L. Manna and T. Pellegrino, ACS Nano, 2012, 6, 3080–3091 CrossRef CAS PubMed.
- A. Bakandritsos, A. Papagiannopoulos, E. N. Anagnostou, K. Avgoustakis, R. Zboril, S. Pispas, J. Tucek, V. Ryukhtin, N. Bouropoulos, A. Kolokithas-Ntoukas, T. A. Steriotis, U. Keiderling and F. Winnefeld, Small, 2012, 8, 2381–2393 CrossRef CAS PubMed.
- M. K. Jaiswal, M. Gogoi, H. Dev Sarma, R. Banerjee and D. Bahadur, Biomater. Sci., 2014, 2, 370–380 RSC.
- M. Magro, R. Campos, D. Baratella, G. Lima, K. Holà, C. Divoky, R. Stollberger, O. Malina, C. Aparicio, G. Zoppellaro, R. Zbořil and F. Vianello, Chemistry, 2014, 20, 11913–11920 CrossRef CAS PubMed.
- Y. Sun, Z.-L. Chen, X.-X. Yang, P. Huang, X.-P. Zhou and X.-X. Du, Nanotechnology, 2009, 20, 135102 CrossRef PubMed.
- K. Hola, Z. Markova, G. Zoppellaro, J. Tucek and R. Zboril, Biotechnol. Adv., 2015, 33, 1162–1176 CrossRef CAS PubMed.
- R. A. Bohara and S. H. Pawar, Appl. Biochem. Biotechnol., 2015, 176, 1044–1058 CrossRef CAS PubMed.
- P. C. Ray, S. A. Khan, A. K. Singh, D. Senapati and Z. Fan, Chem. Soc. Rev., 2012, 41, 3193–3209 RSC.
- Y.-F. Huang, Y.-F. Wang and X.-P. Yan, Environ. Sci. Technol., 2010, 44, 7908–7913 CrossRef CAS PubMed.
- H. Gu, P.-L. Ho, K. W. T. Tsang, L. Wang and B. Xu, J. Am. Chem. Soc., 2003, 125, 15702–15703 CrossRef CAS PubMed.
- H. Gu, P.-L. Ho, K. W. T. Tsang, C.-W. Yu and B. Xu, Chem. Commun., 2003, 1966 RSC.
- A. J. Kell, G. Stewart, S. Ryan, R. Peytavi, M. Boissinot, A. Huletsky, M. G. Bergeron and B. Simard, ACS Nano, 2008, 2, 1777–1788 CrossRef CAS PubMed.
- L. Zeng, K. Luo and Y. Gong, J. Mol. Catal. B: Enzym., 2006, 38, 24–30 CrossRef CAS.
- N. A. Frey, S. Peng, K. Cheng and S. Sun, Chem. Soc. Rev., 2009, 38, 2532–2542 RSC.
- T. Kuroiwa, Y. Noguchi, M. Nakajima, S. Sato, S. Mukataka and S. Ichikawa, Process Biochem., 2008, 43, 62–69 CrossRef CAS.
- Z. Ma, Y. Guan and H. Liu, J. Magn. Magn. Mater., 2006, 301, 469–477 CrossRef CAS.
- S. A. Ansari and Q. Husain, Biotechnol. Adv., 2012, 30, 512–523 CrossRef CAS PubMed.
- C. G. C. M. Netto, H. E. Toma and L. H. Andrade, J. Mol. Catal. B: Enzym., 2013, 85–86, 71–92 CrossRef CAS.
- Y. Ren, J. G. Rivera, L. He, H. Kulkarni, D.-K. Lee and P. B. Messersmith, BMC Biotechnol., 2011, 11, 63 CrossRef CAS PubMed.
- R. A. Bohara, N. D. Thorat and S. H. Pawar, Korean J. Chem. Eng., 2015, 33, 216–222 CrossRef.
- S. Berensmeier, Appl. Microbiol. Biotechnol., 2006, 73, 495–504 CrossRef CAS PubMed.
- P. A. Liberti, C. G. Rao and L. W. M. Terstappen, J. Magn. Magn. Mater., 2001, 225, 301–307 CrossRef CAS.
- F. Paul, D. Melville, S. Roath and D. Warhurst, IEEE Trans. Magn., 1981, 17, 2822–2824 CrossRef.
- N. Seesod, P. Nopparat, A. Hedrum, A. Holder, S. Thaithong, M. Uhlen and J. Lundeberg, Am. J. Trop. Med. Hyg., 1997, 56, 322–328 CAS.
- W.-K. Hofmann, S. de Vos, M. Komor, D. Hoelzer, W. Wachsman and H. P. Koeffler, Blood, 2002, 100, 3553–3560 CrossRef CAS PubMed.
- C. Del Gratta, S. Della Penna, P. Battista, L. Di Donato, P. Vitullo, G. L. Romani and S. Di Luzio, Phys. Med. Biol., 1995, 40, 671–681 CrossRef CAS PubMed.
- R. Edelstein, Biosens. Bioelectron., 2000, 14, 805–813 CrossRef CAS PubMed.
- M. Kala, K. Bajaj and S. Sinha, Anal. Biochem., 1997, 254, 263–266 CrossRef CAS PubMed.
- S. Pour Yazdankhah, A.-L. Hellemann, K. Rønningen and E. Olsen, Vet. Microbiol., 1998, 62, 17–26 CrossRef.
- M. Holzinger, A. Le Goff and S. Cosnier, Front. Chem., 2014, 2, 63 Search PubMed.
- M. A. Tuan and N. H. Hai, J. Phys.: Conf. Ser., 2009, 187, 012059 CrossRef.
- N. H. Luong, D. Van Quy, N. M. Hieu, P. T. Tra, N. H. Nam, N. H. Hai, N. T. Son, P. T. Nghia, N. T. Van Anh and T. T. Hong, J. Nanomater., 2013, 2013, 6 Search PubMed.
- T. R. Sarkar and J. Irudayaraj, Anal. Biochem., 2008, 379, 130–132 CrossRef CAS PubMed.
- R. M. Patil, P. B. Shete, S. M. Patil, S. P. Govindwar and S. H. Pawar, RSC Adv., 2015, 5, 88375–88381 RSC.
- K. Kang, J. Choi, J. H. Nam, S. C. Lee, K. J. Kim, S.-W. Lee and J. H. Chang, J. Phys. Chem. B, 2009, 113, 536–543 CrossRef CAS PubMed.
- H. Jaganathan, R. L. Gieseck and A. Ivanisevic, Nanotechnology, 2010, 21, 245103 CrossRef PubMed.
- S. Prijic, L. Prosen, M. Cemazar, J. Scancar, R. Romih, J. Lavrencak, V. B. Bregar, A. Coer, M. Krzan, A. Znidarsic and G. Sersa, Biomaterials, 2012, 33, 4379–4391 CrossRef CAS PubMed.
- K. Tanaka, N. Kitamura and Y. Chujo, Nucleic Acids Symp. Ser., 2008, 52, 693–694 CrossRef CAS PubMed.
- A. P. Tiwari, S. J. Ghosh and S. H. Pawar, Anal. Methods, 2015, 7, 10109–10120 RSC.
|
This journal is © The Royal Society of Chemistry 2016 |
Click here to see how this site uses Cookies. View our privacy policy here.