DOI:
10.1039/C6RA00750C
(Paper)
RSC Adv., 2016,
6, 24780-24785
Sensitive electrochemiluminescence detection of cancer cells based on a CdSe/ZnS quantum dot nanocluster by multibranched hybridization chain reaction on gold nanoparticles†
Received
10th January 2016
, Accepted 1st February 2016
First published on 29th February 2016
Abstract
In this work, we prepared a novel amplified electrochemiluminescence (ECL) signal probe based on a CdSe/ZnS quantum dot (QD) nanocluster by multibranched DNA hybridization chain reaction (HCR) on gold nanoparticles (GNPs), and developed a sensitive ECL biosensor for the detection of cancer cells. Firstly, a new gold nanorod–hematite nanostructure was used as a magnetic nano-amplified platform to assemble abundant capture DNA (aptamer) on the electrode. Next, the probe DNA was hybridized with the aptamers and multibranched HCR was initiated on the GNPs. Thus the numerous QD nanoclusters showed highly intense ECL signal. Finally, the electrodes were incubated in target cancer cells for 50 min. Upon specific recognition of target cells to aptamers, the signal probes were released from the electrode. Thus a small amount of target cells can trigger the release of a large number of QDs, leading to a remarkable amplification of ECL signal, which was used for sensitive detection of cancer cells. The change of ECL signal was linear to the cell concentrations in the range of 500–10
000 cells mL−1, and the detection limit was 230 cells mL−1 at 3σ. A series of five duplicate measurements of 2000 cells mL−1 were used for estimating the precision, and the relative standard was 6.1%. Importantly, the proposed strategy combines the excellent ECL of QDs with cooperative amplification of HCR and the nano-enhanced effect of GNPs, which enables sensitive and selective detection of cancer cells. So far, the QD nanocluster has been used as an ECL signal probe for assays of cancer cells for the first time, and has great potential for early clinical applications in the diagnosis of cancer.
Introduction
Electrochemiluminescence (ECL) has remarkable features, such as simplicity, rapidity, high sensitivity and easy controllability. ECL sensors, as powerful devices, have been used extensively for the sensitive detection of a wide variety of analytes.1–3 Recently, the biosensors and bioassays, by virtue of the intrinsic ECL of a variety of QD nanostructures, have been extensively developed for diagnostic purposes, due to their efficient ECL emission and facile bioconjugation, as well as nano-enhanced signal amplification.4,5 A mass of methods involving immunoassays,6,7 aptamer8,9 or DNA binding,10 and cytosensing11,12 have been developed for sensitive detection of biological targets using ECL of nanostructures.
Cancer is today's most pressing health concern, the research of accurate and sensitive recognition and detection of cancer cells is extremely important for cancer diagnosis and therapy. Up to now, several approaches have been developed for tumor cells detection, such as flow cytometry,13 polymerase chain reaction (PCR),14 microassay-based techniques,15 and cell enrichment methods.16 Aptamers are single-stranded nucleic acids isolated from random-sequence DNA or RNA libraries by an in vitro selection process termed SELEX (systematic evolution of ligands by exponential enrichment).17 The high affinity and specificity of aptamers make aptamers attractive as sensing elements for bioanalytical applications.18 Worth noticing is that aptamer-based specific recognition of tumor cells and detection technique has become an attractive field in recent years.19 However, there has been rarely ECL biosensors to achieve accurate and sensitive diagnosis of human cancer at molecular level, it is in urgent need to develop highly sensitive and selective ECL method for the detection of cancer cells at their earliest stages. So far, various DNA-amplification techniques were utilized in bioanalysis for signal amplification, such as polymerase chain reaction (PCR)20,21 rolling circle amplification (RCA),22 strand displacement amplification (SDA)23 and exonuclease/nicking endonuclease-assistant amplification,24,25 where signal amplification requires the use of a thermo-stable or a strand-displacement polymerase or other enzymes. Hybridization chain reaction (HCR) as a DNA self-polymerization process has been reported recently,26,27 which allows for selective and specific extension without enzyme. Among the enzyme-free amplification methods, HCR is one of the most attractive,28,29 and has been applied to nucleic acid and protein sensing assays.30–32 However, there has been rare report on HCR-based amplification technique to achieve sensitive ECL detection of cancer cells.
Herein, we prepared a novel CdSe/ZnS QDs nanocluster by multibranched HCR on GNPs, and used as amplified signal probe for sensitive ECL detection of cancer cells. The gold nanorod–hematite nanostructure with excellent electrical and magnetic properties was used as nano-amplified platform to assemble a large number of capture DNA on the electrode. With hybridization of probe DNA with c-DNA1 and HCR on GNPs, the abundant QDs on nanocluster showed greatly amplified ECL. The specific recognition of target cells to aptamers resulted in much decrease in ECL signal, which enables sensitive detection of cancer cells. So far, it is for the first time that the HCR-based QDs nanocluster was fabricated and used as ECL signal probe for assays of cancer cells, which has promising applications in diagnosis of cancer.
Results and discussion
Characterization of the CdSe/ZnS QDs
Fig. 1A presents the transmission electron microscopy (TEM) image of the CdSe/ZnS QDs, the average diameter was about 10 nm. Fig. 1B shows the photoluminescence (PL) spectrum of the CdSe/ZnS QDs in aqueous solution, the PL emission peak (Ex = 450 nm) was at 600 nm, and the peak intensity was very high, indicating that the QDs possess excellent luminescent properties.
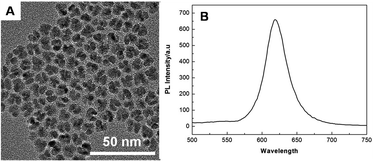 |
| Fig. 1 (A) TEM image and (B) photoluminescence (PL) of the CdSe/ZnS QDs. | |
Principle of the HCR-based ECL assay of cancer cells
The fabrication principle for amplified ECL detection of cancer cells using CdSe/ZnS QDs nanocluster by multibranched HCR on GNPs is depicted in Scheme 1. The probe-DNA (p1) and initiator DNA (s1) are previously conjugated to the GNPs in a molar ratio of 1
:
10 (step a). In addition, the hairpin DNA H1 and H2 are both labelled with the CdSe/ZnS QDs. After the gold nanorod–hematite nanostructure is immobilized on the Au electrode, the aptamers for cancer cells are linked to the nanostructure (step b). Then the probe-DNA (p1) on the GNPs hybridized with the aptamers (step c), and DNA s1 acts as not only bio-bar codes to avoid cross-linking reaction, but also as the initiator to carry out the subsequent HCR with H1* and H2* labelled by QDs. In the HCR process, DNA s1 hybridizes with the sticky end of H1* and opens the hairpin. The released sticky sequence of H1* will further hybridize with H2* to open its hairpin and expose a new sticky end on H2*. This sticky end is identical in sequence to the initiator strands (s1). In this manner, each DNA s1 can trigger a chain reaction of hybridization events between H1* and H2*, and yield long dsDNA with large number of QDs (step d). Thus numerous QDs nanowires are produced by multibranched HCR on GNPs, which greatly amplified ECL signal of QDs. Importantly, in the absence of the initiator strands, both hairpin DNA molecules (H1* and H2*) are in the closed form and cannot be conjugated onto the QDs nanowires. In the presence of target cancer cells, with the specific recognition of target cells to aptamers (step e), the double strands aptamer/probe DNA dehybridized, and the QDs nanowires signal probe were released, which resulted in great decrease in ECL signal. Thus the ECL signal change was much amplified by the dual amplification strategy of HCR and nano-enhanced effect of GNPs. The ECL signal change was proportional to the concentration of target cells, which could be used for the sensitive detection of cells concentration.
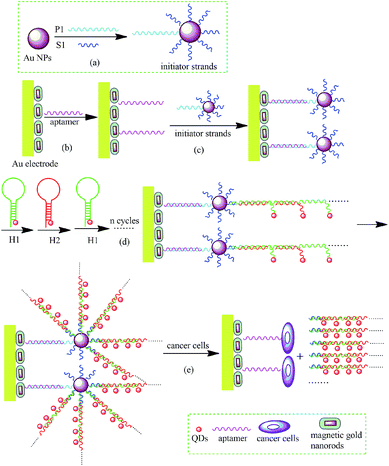 |
| Scheme 1 Schematic representation strategy for amplified ECL assay of cancer cells based on CdSe/ZnS QDs by HCR on gold nanoparticles. | |
Characterization of the HCR-based CdSe/ZnS QDs nanocluster
As described above, the amplification of the ECL signal was implemented by the formation of the long QDs nanowires via multibranched HCR on GNPs. To realize our design, the formed QDs nanowires were characterized by atomic force microscopy (AFM) imaging and UV-vis spectra.
Fig. 2 presents the representative atomic force microscope (AFM) images of the pure gold nanoparticles (A and B) and HCR-induced QDs nanowires (C and D). Marked difference in the morphology and height was observed between the pure GNPs and QDs nanowires on the GNPs. In contrast, image A showed numerous spherical GNPs, the height was approximately 40 nm. While image B displayed many long DNA–QDs nanowires, and the height increased slightly. The results revealed that the QDs nanowires structure was formed via HCR of H1* and H2* on GNPs.
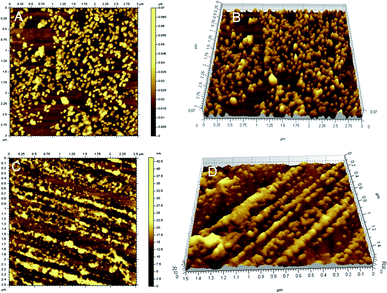 |
| Fig. 2 Representative AFM images of the pure gold nanoparticles (A and B) and HCR-based CdSe/ZnS QDs nanowires on the GNPs (C and D). | |
In addition, the DNA–QDs nanowires were also characterized using UV-vis absorption spectroscopy (Fig. S1†). There were two characteristic peaks at 254 and 474 nm for the DNA–QDs nanowires on GNPs, which were attributed to DNA molecules and GNPs, respectively. By comparison, inset in Fig. S1† displayed the typical absorption spectrum of the pure GNPs, the absorption peak was at 519 nm. Specially, the obvious difference in the absorption peak maybe due to a little energy transfer between the nearest QDs and GNPs in DNA–QDs nanowires, which could influence the position of the plasmon band.9
In summary, the above methods demonstrated that the DNA–QDs nanowires were successfully prepared by HCR on GNPs.
Preparation and characterization of the modified electrodes for ECL detection
Fig. 3 shows the field-emission scanning electron microscopy (FE-SEM) images of the modified electrode for ECL assay. As shown in Fig. 3A, when the gold nanorod–hematite nanostructure was firstly immobilized on the Au electrode, some nanorods in the coating layer were observed. Herein, the magnetic gold nanorods were more stable and promising for modifying the magnetic Au electrode to develop the ECL biosensor. Subsequently, the aptamers were linked to the nanostructure, and the p1-GNPs-s1 probe was assembled on the electrode by hybridization reaction, it was found that a thick film with many nanoparticles was homogeneously formed on the electrode (Fig. 3B), indicating the successful fabrication of the ECL biosensor.
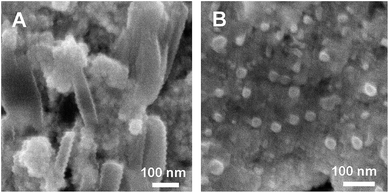 |
| Fig. 3 SEM images of the modified electrode for ECL assay. (A) The gold nanorod–hematite nanostructure on the Au electrode, (B) the p1-GNPs-s1 probe assembled on the electrode. | |
Electrochemical and ECL behaviors of the CdSe/ZnS QDs nanowires
Fig. 4 shows an ECL–potential curve (b) and cyclic voltammogram (CV, inset) of the CdSe/ZnS QDs nanowires as ECL signal probe on the electrode. There is one cathodic peak at −0.93 V in the CV response, corresponding to the reduction of S2O82−. One ECL peak was observed at −1.49 V in the ECL–potential curve (b), resulting from the reaction of the QDs with S2O82−. According to previous reports, electrochemically reduced and oxidized Si NCs33 or CdSe NCs34 can react with coreactants to produce ECL. In this case, upon the potential scan with an initial negative direction, CdSe/ZnS QDs immobilized on the electrode were reduced to nanocrystalline species (QD−˙), while the coreactant S2O82− was reduced to strong oxidant SO4−˙. Then SO4−˙ reacted with the negatively charged QD−˙ by injecting a hole into the highest occupied molecular orbital (HOMO) to form the excited state (QD*) that emits light. The possible ECL mechanisms are described in Scheme 2 with the following equations:35 |
S2O82− + e− → SO42− + SO4−˙
| (2) |
|
QD−˙ + SO4−˙ → QD* + SO42−
| (3) |
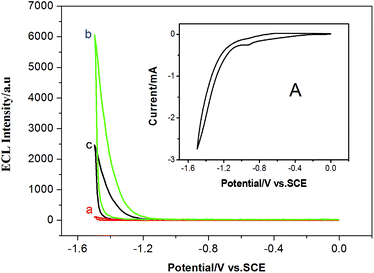 |
| Fig. 4 ECL signals of (a) the bare electrode, (b) the CdSe/ZnS QDs ECL probe on the electrode, (c) in the presence of target Ramos cells (8000 cells mL−1). 0.1 M PBS (pH 7.4) containing 0.1 M KCl and 0.05 M K2S2O8. | |
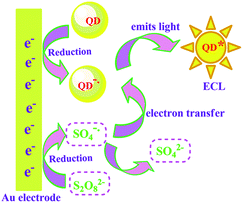 |
| Scheme 2 | |
The ECL signal from the CdSe/ZnS QDs nanowires as ECL probe was very high, suggesting that the QDs nanowires exhibit excellent ECL properties and have promising potential in ECL biosensing.
HCR-based ECL assay of cancer cells
The strategy feasibility for amplified ECL assay of cancer cells by HCR-based QDs nanowires was investigated. As shown in Fig. 4, the background ECL signal of the bare Au electrode was very low (curve a). However, after the CdSe/ZnS QDs nanowires as ECL signal probe were assembled on the electrode by multibranched HCR, much higher ECL signal was observed (curve b), indicating that the CdSe/ZnS QDs nanowires probe was successfully fabricated and used for ECL detection. In the presence of target Ramos cells, the QDs nanowires were released from the electrode due to the specific recognition of target cells to aptamers, the ECL signal obviously decreased (curve c). The results indicate that the strategy could be applied to the detection of cancer cells.
Analytical performance of ECL biosensor
Fig. 5 showed the ECL signals that were responsive to different concentrations of target Ramos cells. The ECL peak intensity gradually decreased with increasing concentrations of cells. As the specific binding of more cells with aptamers induced the release of more QDs signal probe off the electrode, thus the ECL signal gradually decreased. The changes of ECL peak intensity increased with the cell concentrations in the range of 500–20
000 cells mL−1 (inset). The results suggested that the cells concentration could be determined with the ECL biosensor.
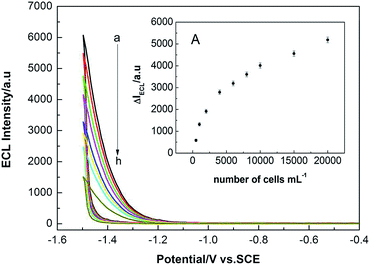 |
| Fig. 5 ECL signal responses upon different concentrations of Ramos cells. The concentrations of Ramos cells (cells mL−1): (a) 0; (b) 500; (c) 1000; (d) 2000; (e) 4000; (f) 6000; (g) 8000; (h) 15 000. Inset A: relationship between ΔI and Ramos cells concentration. | |
The standard calibration curve for cell detection was shown in Fig. 6. The changes of ECL peak intensity was linear to the cell concentrations in the range of 500–10
000 cells mL−1, and the detection limit was calculated to be 230 cells mL−1 at 3σ. As 100 μL of cells was used in our experiment detection, thus this detection limit was equivalent to 23 cells/100 μL, suggesting that this enzyme-free amplified strategy is more sensitive and has greater potential for early detection of cancer cells compared with other ECL biosensors.11,33 A series of five duplicate measurements of 2000 cells mL−1 were used for estimating the precision, and the relative standard was 6.1%.
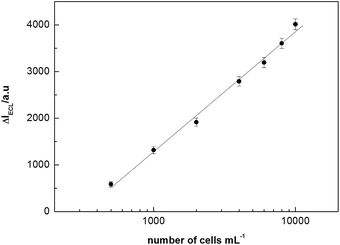 |
| Fig. 6 The logarithmic calibration curve for detection of Ramos cells (cells mL−1). | |
Selectivity of the ECL biosensor. To assess the specificity of the ECL assay for target Ramos cells, the same experiment was repeated with the control CEM cells at the same concentration (2000 cells mL−1) (Fig. S2A,† graph b). The ECL response change was almost the same with the blank solution without cells (Fig. S2A,† graph a), while the target cells clearly show a significantly higher response change of ECL signal than that of the control cells (Fig. S2A,† graph c). The reason may be that the control CEM cells has no specific interaction with the aptamers, which could not lead to the release of QDs probe from the electrode, and thus there is no response change in ECL signal. By comparison, the specific binding of target cells to aptamers enable the QDs probe leave from the electrode, which result in significant change of ECL signal. The results indicate that the method has good selectivity for assay of target Ramos cells.
Detection of target cells in complex samples. In order to evaluate the potential application of the proposed method, the assay was performed using artificial complex samples by mixing equal amounts of Ramos target cells, control CEM cells and K562 cells at 35 °C (pH 7.4 PBS), the ECL responses were shown in Fig. S2B.† The control cells produced no noticeable difference in the sample versus pure target cells. The results suggest the assay was able to collect the Ramos cells in complex sample, showing good selectivity and actual application of the assay.
Stability and reproducibility of the ECL biosensor. The ECL biosensor was stored in pH 7.4 PBS at 4 °C over 2 weeks, the analytical performances did not show an obvious decline, demonstrating that the ECL biosensor had good stability. The reason is that the gold nanorod–hematite nanostructure with good magnetic property can strongly bind to the magnetic electrode, and then the aptamers and QDs probe were linked to the electrode with high stability. In addition, the reproducibility of the biosensor was estimated by determining 2000 cells mL−1 of target cells with four biosensors made at the same electrode. Four measurements from the batch resulted in a relative standard deviation of 5.2%, indicating good reproducibility of the developed ECL biosensor.In summary, a novel CdSe/ZnS QDs nanocluster was fabricated by multibranched hybridization chain reaction, and used as amplified ECL signal probe for sensitive ECL detection of cancer cells for the first time. This study has several significant advantages. Firstly, a large number of CdSe–ZnS QDs were assembled onto the novel nanocluster due to the multibranched DNA HCR on GNPs, taking advantages of cooperative amplification of HCR and nano-enhanced capability, which could greatly amplify the ECL signal of QDs. Secondly, a novel nano-amplified and magnetic platform based on the magnetic gold nanorod–hematite nanostructure was employed to assemble abundant capture DNA on the magnetic electrode, which greatly improved the sensitivity and stability. Thirdly, high specificity of aptamers to target cells much favored for the improvement of selectivity and sensitivity. To the best of our knowledge, this is the first report that the QDs nanocluster based on multibranched DNA HCR on nanoparticles was developed, and applied to the ECL assay of cells, which has promising applications for the early and accurate detection of cancer cells.
Experimental
Preparation of the Au–Fe2O3 heterostructures
A previously published procedure with slight modifications was used.36 The seed solution was made as follows. HAuCl4 (0.01 M, 0.25 mL) was added into cetyltrimethylammonium bromide (CTAB, 0.1 M, 9.75 mL) in a 15 mL plastic tube with gentle mixing. A freshly prepared, ice-cold NaBH4 solution (0.01 M, 0.6 mL) was then injected quickly into the mixture solution, followed by rapid inversion for 2 min. The resultant seed solution was kept at room temperature for 2 h before use. To grow the Au nanorods, HAuCl4 (0.01 M, 2.0 mL) and AgNO3 (0.01 M, 0.4 mL) were mixed with CTAB (0.1 M, 40 mL) in a 50 mL plastic tube. HCl (1.0 M, 0.8 mL) was then added to adjust the pH of the solution to 1–2, followed by the addition of ascorbic acid (0.1 M, 0.32 mL). Finally, the CTAB-stabilized seed solution (0.02 mL) was injected into the growth solution. The solution was gently mixed for 10 s and left undisturbed overnight.
Typically, the as-grown Au nanorod solution (10 mL) was first mixed together with an acetonitrile solution of ferric acetylacetonate (0.05 M, 0.12 mL). The pH of the mixture solution was then adjusted to ∼9 with an aqueous NaOH solution (1.0 M, ∼0.3 mL). After being stirred mildly for 10 min, the solution was transferred into a Teflon-lined, 25 mL autoclave. The autoclave was then placed into an isothermal oven, which was preset at 190 °C. After being kept in the oven for 3 h, the autoclave was taken out and cooled down naturally to room temperature. The product was washed by two cycles of centrifugation and redispersion in deionized water.
Synthesis of CdSe/ZnS QDs
Sodium borohydride (0.0950 g) were added to a small flask, then 4 mL of ultrapure water was added. The solution was stirred and degassed with nitrogen for 30 min. Then 0.0947 g of selenium powder was quickly injected into the reaction flask and stirred under room temperature. After the selenium powder disappeared completely, the clear NaHSe of 0.1 M was obtained.
CdCl2·2.5H2O (0.5709 g), ultrapure water (50.0 mL), and mercaptoacetic acid (400 μL) were placed in a 100 mL round flask, degassed with N2 gas, and the pH was adjusted to 11 with 0.5 mol L−1 NaOH. Then the NaHSe solution was quickly injected into the reaction flask, heated to 100 °C, and further reacted for 30–40 min. The resulting yellow and clear solution was cooled to 45 °C. At this temperature, 0.21 g of Na2S·9H2O and 0.25 g of ZnSO4 were dissolved in 5 mL of ultrapure water respectively, and then injected into the reaction flask alternately. After reacted for 30 min and cooled to room temperature, the resulting CdSe/ZnS QDs solution was obtained.
ECL detection of cancer cells by multibranched hybridization chain reaction
Conjugation of p1 and s1 with gold nanoparticles. The biobarcode-DNA (s1) (500 μL, 1.0 × 10−5 M) and probe-DNA (p1) (50 μL, 1.0 × 10−5 M) were added to 1 mL of gold colloid, and incubated at 37 °C overnight with gentle shaking. To remove excess DNA, the solution was centrifuged at 15
000 rpm for 30 min. The oily precipitate was then washed with 10 mM phosphate buffer two times, recentrifuged, and redispersed in 100 μL of PBS, and stored in a refrigerator (4 °C). The resulting solution was marked as a1.
Conjugation of H1 and H2 with the CdSe/ZnS QDs. After 100 μL of CdSe/ZnS QDs were activated by EDC (0.1 M) and NHS (0.025 M) for 30 min, 10 μL of H1 and H2 was added to the QDs. Then these solutions were mixed and incubated at 25 °C for 12 h, centrifuged at 10
000 rpm and redispersed in 100 μL of PBS. The solution was marked as b1.
HCR-based ECL assay of cancer cells. The magnetic Au electrodes were polished with alumina slurries (1, 0.3, 0.05 μm), sonicated in deionized water, and dried with nitrogen gas. After 7 μL of the magnetic Au–Fe2O3 heterostructure solution was dropped to the electrode surface and dried naturally at the room temperature, 10 μL of the capture DNA (aptamer) solution was dropped to the electrode surface and reacted in a moist environment for 10 h.The electrodes modified with aptamers were immersed in the above solution (a1), incubated at 37 °C for 2 h, and washed twice with PBS (pH 7.4). Then the electrodes were immersed in the above solution (b1), incubated at 37 °C for 2 h, and washed twice with PBS (pH 7.4). For ECL detection, the electrodes were immersed in 100 μL of target cancer cells with different concentrations, incubated for 50 min, and washed twice for ECL measurement.
The ECL emission was detected with a model MPI-A electrochemiluminescence analyzer using a three-electrode system at room temperature. The electrodes were a modified magnetic Au disk working electrode (4 mm diameter), a saturated calomel reference electrode, and a Pt counter electrode. The modified electrodes above were in contact with 0.1 M PBS (pH 7.4) containing 0.05 M K2S2O8 and 0.1 M KCl and scanned from 0 to −1.5 V. The spectral width of the photomultiplier tube (PMT) was 200–800 nm, and the voltage of the PMT was −800 V in the detection process. ECL signals related to the cells concentrations were measured.
Acknowledgements
This work was supported by the National Natural Science Foundation of China (No. 21175078, 21575072), and the Financial Support by Open Research Fund of Top Key Discipline of Chemistry in Zhejiang Provincial Colleges and Key Laboratory of the Ministry of Education for Advanced Catalysis Materials (Zhejiang Normal University) (No. ZJHX201508).
References
- D. J. E. Piper, G. J. Barbante, N. Brack, P. J. Pigram and C. F. Hogan, Langmuir, 2011, 27, 474–480 CrossRef CAS PubMed.
- Q. H. Cai, L. F. Chen, F. Luo, B. Qiu, Z. Y. Lin and G. N. Chen, Anal. Bioanal. Chem., 2011, 400, 289–294 CrossRef CAS PubMed.
- Z. Y. Lin, J. H. Chen and G. N. Chen, Electrochim. Acta, 2008, 53, 2396–2401 CrossRef CAS.
- J. Lei and H. Ju, Trends Anal. Chem., 2011, 30, 1351–1359 CrossRef CAS.
- H. Huang, J. Li and J. Zhu, Anal. Methods, 2011, 3, 33–42 RSC.
- G. F. Jie, P. Liu and S. S. Zhang, Chem. Commun., 2010, 8, 1323–1325 RSC.
- L. Li, K. Liu, G. Yang, C. Wang, J. Zhang and J. Zhu, Adv. Funct. Mater., 2011, 21, 869–878 CrossRef CAS.
- J. Wang, Y. Shan, W. Zhao, J. Xu and H. Chen, Anal. Chem., 2011, 83, 4004–4011 CrossRef CAS PubMed.
- G. F. Jie, J. X. Yuan and J. Zhang, Biosens. Bioelectron., 2012, 31, 69–76 CrossRef CAS PubMed.
- F. Divsar and H. Ju, Chem. Commun., 2011, 47, 9879–9881 RSC.
- G. F. Jie, L. Wang, J. X. Yuan and S. S. Zhang, Anal. Chem., 2011, 83, 3873–3880 CrossRef CAS PubMed.
- E. Han, L. Ding, S. Jin and H. Ju, Biosens. Bioelectron., 2011, 26, 2500–2505 CrossRef CAS PubMed.
- M.-S. L. Raddatz, A. Dolf, E. Endl, P. Knolle, M. Famulok and G. Mayer, Angew. Chem., Int. Ed., 2008, 47, 5190–5193 CrossRef CAS PubMed.
- X. Zhang, R. M. K. Yu, P. D. ones and G. K. W. Lam, Environ. Sci. Technol., 2005, 39, 2777–2785 CrossRef CAS PubMed.
- Y. Xu, J. A. Phillips, J. Yan, Q. Li, Z. H. Fan and W. Tan, Anal. Chem., 2009, 81, 7436–7442 CrossRef CAS PubMed.
- J. A. Phillips, Y. Xu, Z. Xia, Z. H. Fan and W. Tan, Anal. Chem., 2009, 81, 1033–1039 CrossRef CAS PubMed.
- A. D. Ellington and J. W. Szostak, Nature, 1990, 346, 818–822 CrossRef CAS PubMed.
- R. Freeman, E. Sharon, R. Tel-Vered and I. Willner, J. Am. Chem. Soc., 2009, 131, 5028–5029 CrossRef CAS PubMed.
- X. Chen, M. C. Estévez, Z. Zhu, Y. F. Huang, Y. Chen, L. Wang and W. Tan, Anal. Chem., 2009, 81, 7009–7014 CrossRef CAS PubMed.
- G. Wan, Q. EnLim and H. P. Too, RNA, 2010, 16, 1436–1445 CrossRef CAS PubMed.
- A. Csordas, A. E. Gerdon, J. D. Adams, J. Qian, S. S. Oh, Y. Xiao and H. T. Soh, Angew. Chem., Int. Ed., 2010, 49, 355–358 CrossRef CAS PubMed.
- Y. Cheng, X. Zhang, Z. Li, X. Jiao, Y. Wang and Y. Zhang, Angew. Chem., Int. Ed., 2009, 48, 3268–3272 CrossRef CAS PubMed.
- L. P. Qiu, Z. S. Wu, G. L. Shen and R. Q. Yu, Anal. Chem., 2011, 83, 3050–3057 CrossRef CAS PubMed.
- G. F. Jie and J. X. Yuan, Anal. Chem., 2012, 4, 2811–2817 CrossRef PubMed.
- H. Zhang, F. Li, B. Dever, X. F. Li and X. C. Le, Chem. Rev., 2013, 113, 2812–2841 CrossRef CAS PubMed.
- Y. Peng, H. M. T. Choi, C. R. Calvert and N. A. Pierce, Nature, 2008, 451, 318–322 CrossRef PubMed.
- H. M. T. Choi, J. Y. Chang, L. A. Trinh, J. E. Padilla, S. E. Fraser and N. A. Pierce, Nat. Biotechnol., 2010, 28, 1208–1212 CrossRef CAS PubMed.
- S. Venkataraman, R. M. Dirks, P. W. K. Rothemund, E. Winfree and N. A. Pierce, Nat. Nanotechnol., 2007, 2, 490–494 CrossRef PubMed.
- Y. Peng, H. M. T. Choi, C. R. Calvert and N. A. Pierce, Nature, 2008, 451, 318–322 CrossRef PubMed.
- G. Zhu, J. Zheng, E. Song, M. Donovan, K. Zhang, C. Liu and W. Tan, Proc. Natl. Acad. Sci. U. S. A., 2013, 110, 7998–8003 CrossRef CAS PubMed.
- Y. Chen, J. Xu, J. Su, Y. Xiang, R. Yuan and Y. Q. Chai, Anal. Chem., 2012, 84, 7750–7755 CrossRef CAS PubMed.
- X. Liu, F. Wang, R. Aizen, O. Yehezkeli and I. Willner, J. Am. Chem. Soc., 2013, 135, 11832–11839 CrossRef CAS PubMed.
- Z. Ding, B. M. Quinn, S. K. Haram, L. E. Pell, B. A. Korgel and A. J. Bard, Science, 2002, 296, 1293–1297 CrossRef CAS PubMed.
- G. Nie, Z. Bai, W. Yu and J. Chen, Biomacromolecules, 2013, 14, 834–839 CrossRef CAS PubMed.
- N. Myung, Z. Ding and A. J. Bard, Nano Lett., 2002, 2, 1315–1319 CrossRef CAS.
- Z. H. Bao, Z. H. Sun, Z. F. Li, L. W. Tian, T. Ngai and J. Wang, Langmuir, 2011, 27, 5071–5075 CrossRef CAS PubMed.
Footnote |
† Electronic supplementary information (ESI) available: Experimental details, UV-vis, TEM and the optimization and selectivity are included and additional figures. See DOI: 10.1039/c6ra00750c |
|
This journal is © The Royal Society of Chemistry 2016 |
Click here to see how this site uses Cookies. View our privacy policy here.