DOI:
10.1039/C5RA25673A
(Paper)
RSC Adv., 2016,
6, 20058-20066
Photoelectrochemical glucose biosensor based on a dehydrogenase enzyme and NAD+/NADH redox couple using a quantum dot modified pencil graphite electrode†
Received
2nd December 2015
, Accepted 24th January 2016
First published on 27th January 2016
Abstract
A simple, disposable and economical modified electrode was prepared by electrodeposition of hybrid quantum dots (ZnS–CdS) onto a pencil graphite electrode (PGE) surface and subsequent immobilization of glucose dehydrogenase (GDH) onto the quantum dot modified electrode (GDH/ZnS–CdS/PGE). The prepared electrode was effectively used for the photoelectrochemical determination of glucose in a flow injection analysis (FIA) system using a new home-made flow cell which was designed for PGEs for the first time. Results from the cyclic voltammetric and FI amperometric measurements revealed that the GDH/ZnS–CdS/PGE is capable of signaling photoelectrocatalytic activity involving NADH when the surface of the GDH/ZnSr–CdS/PGE is irradiated with a light source with a fiber optic cable (250 W halogen lamp). The currents of NADH produced by the enzymatic reaction in the photoamperometric FIA system under optimized conditions (carrier stream: 0.1 M phosphate buffer solution (pH 7.0) containing 1.0 M KCl and 10.0 mM NAD+, applied potential: +0.8 V vs. Ag/AgCl/KCl(sat.); flow rate: 0.6 mL min−1, sample loop: 100 μL; transmission tubing length: 10 cm) were linearly correlated with the glucose concentration. Calibration curves were obtained for glucose concentrations within a range from 0.2 to 8.0 mM. The detection limits were found to be 0.09 and 0.05 mM for the amperometric and photoamperometric methods, respectively. The relative standard deviations (n = 7) for 0.5 mM glucose were 4.5% and 3.5% from the photoamperometric and amperometric results respectively. The photoelectrochemical biosensor was applied to real samples successfully. The results with this biosensor showed good selectivity, repeatability and sensitivity for monitoring glucose in amperometric and photoamperometric FIA studies.
Introduction
Semiconductor quantum nanoparticles (QNPs) have received great attention in electrochemical and optical sensor/biosensor studies due to their special electronic and photophysical properties, such as broad adsorption, narrow emission and a high quantum yield.1-5 Especially, an increasingly growing area of electrochemical sensing studies is the construction of photoelectrochemical sensors/biosensors based on quantum dot (QD) modified electrodes, which exhibit a powerful way to detect chemical/biochemical molecules compared to other sensor types.1,2 The reasons for this can be explained from some significant advantages of QD-based photoelectrochemical sensors such as a fast response, remarkable sensitivity, the simple, cheap and portable design of the sensor system, easy integration, extension to light addressable sensors etc.2,6,7 Photoelectrochemical sensor operations consist of a number of steps: (i) immobilization of QDs onto an electrode, (ii) illumination of the electrode surface and (iii) generation of a photocurrent, which depends on the type and concentration of the respective analyte in the supporting electrolyte. When the QD modified electrode surface is illuminated using a light source, photoexcitation of the semiconductor quantum dots yields electron–hole pairs in the conduction- and valence-band levels. If the electrons of the electrode transfer to a hole in the valence band of the QDs and the electrons in the conduction band transfer to electron acceptors (oxidant molecules) in the surrounding solution simultaneously, a cathodic photocurrent is thus generated. On the contrary, if the electrons of the conduction band transfer to the electrode and at the same time electron donors in the solution transfer their electrons to a hole in the valance band, an anodic photocurrent is generated. If the analytes behave as electron donors/acceptors or react with the electron donors/acceptors in the solution, the photocurrent of the electrode can be monitored using the analyte concentration. Based on this principle, many electrochemical/photoelectrochemical sensors and biosensors have been developed for the sensitive detection of biological, environmentally important molecules.2,6,7
One of the important applications of semiconductor nanoparticles is in the photoelectrocatalytic oxidation of NADH,8–13 and the construction of enzyme based electrochemical and photoelectrochemical biosensors dependent on the NAD+/NADH redox couple and dehydrogenase enzymes.11–14 Moreover, recently core–shell QDs or QNPs, such as CdS–ZnS and CdSe–CdS, or hybrid nanomaterials such as carbon nanotube-QDs or -QNPs, graphene-QDs or -QNPs etc. have been preferred instead of using only QDs or a nanomaterial in the electrochemical studies.2,8–14 Core–shell QDs or hybrid nanomaterials have better charge separation than using a single QD or nanomaterial and therefore they are ideal candidates for sensing and biosensing applications due to their high quantum yield, photostability for photoelectrochemical studies, extremely large surface-to-atom ratio and sensitivity to surface ligands.2,15–17 For example, dopamine sensitized nanoporous TiO2 on an indium tin oxide (ITO) electrode,8 a poly(4,4′-diaminodiphenyl sulfone)/nano TiO2 composite film modified ITO electrode9 and a graphene-TiO2 nanohybrid modified glassy carbon electrode (GCE)10 have been successfully used for the photoelectrocatalytic oxidation of NADH under visible light irradiation. In addition, Jafari et al. studied electrocatalytic and photoelectrocatalytic oxidation of NADH using a reduced graphene oxide/CdS-QD/poly-Nile blue nanocomposite modified GCE and also photoelectrochemical sensing of glucose using glucose dehydrogenase immobilized onto a nanocomposite modified electrode surface.11 They reported that photoelectrochemical sensing is more sensitive than electrochemical sensing for the detection of NADH and also glucose using the proposed nanocomposite electrodes. In another study, CdSe/ZnS hybrid QDs modified onto a gold electrode by chemisorption via benzene dithiol were used for photoelectrochemical sensing of NADH and also glucose.13 The current signal was triggered by illumination of the electrode surface.
In this study, a ZnS–CdS hybrid QNP modified pencil graphite electrode (PGE) is proposed for photoelectrochemical sensing of NADH and glucose for the first time. When compared with other carbon based electrodes, PGEs have the same advantages such as high electrochemical reactivity, commercial availability, good mechanical rigidity, disposability, a lower cost, a lower level of technology, and ease of modification.18–30 It has been reported that the pencil lead electrodes offer a renewable surface which is simpler and faster to achieve than polishing procedures, common with solid electrodes, and provides useful and reproducible results for the individual surfaces.18 Thus PGEs have been extensively used in various electroanalytical studies due to the useful properties of PGEs.18–30 In addition, a novel part of this study is that the photoelectrochemical biosensing of glucose using glucose dehydrogenase (GDH) immobilized onto the ZnS–CdS/PGE was performed in a FIA system by using a new home-made photoelectrochemical flow cell which was designed for PGEs for the first time. According to a search of the literature, photoelectrochemical biosensing of glucose in a FIA system using GDH immobilized onto a QD modified PGE has not been reported yet, although a ZnS–CdS modified PGE has been used for the electrochemical biosensing of glucose based on glucose oxidase (GOD) enzymes in a FIA system by using a new home-made photoelectrochemical flow cell.31 The superior aspect of the proposed study is the construction of a photoelectrochemical biosensor for a FIA system using a GDH modified PGE compared with the study of ref. 31, in which only an electrochemical biosensor was constructed. Thus, this study uses a combination of QDs, PGEs, photoelectrochemistry and FIA for photoelectrochemical biosensing of glucose, which offers some advantages such as (i) a disposable, practical, easy-to-use and low cost biosensor due to the useful properties of PGEs, (ii) fast and economic analysis (FIA exhibits fast analysis and a lower cost because of lower consumption of the reactant) and (iii) very good immobilization of GDH, resulting in the construction of a photoelectrochemical biosensor with good selectivity and sensitivity due to the unique functions of QDs and advantages of photoelectrochemistry.
Results and discussion
Characterization of the CdS–ZnS/MAA/PGE
Electrochemical impedance spectroscopy (EIS) is a useful technique providing detailed information on the impedance changes of an electrode surface, which gives useful information about the modification of electrode surfaces as well as their use in electrochemical sensing applications.32 Therefore, EI spectra of the bare and QD modified PGEs were recorded in 10.0 mmol L−1 [Fe(CN)6]3−/4− containing 0.10 M KCl (Fig. 1B). The semicircle diameter for the pretreated bare PGE (about 222 ohm, Fig. 1B-A) was very small, indicating that charge transfer was relatively facile at the bare electrode. However, the Rct value slightly increased after CdS (about 600 ohm, Fig. 1B-B) and also CdS–ZnS (about 900 ohm, Fig. 1B-C) were modified onto the PGE surface, indicating that the CdS and also the ZnS on the PGE surface decreased the electron transfer rate between the redox probe and the electrode surface. QDs have semiconductor properties and their conductivities are lower than that of the bare PGE. These results indicated that CdS and ZnS were electrochemically precipitated onto the pretreated PGE surface.
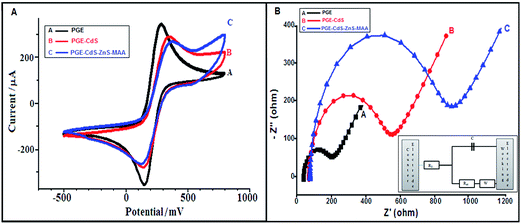 |
| Fig. 1 (A) Cyclic voltammograms (scan rate: 50 mV s−1), and (B) electrochemical impedance spectra of the modified electrodes in 0.1 M KCl containing 10.0 mM K3[Fe(CN)6]/K4[Fe(CN)6]. (Inset: electrochemical impedance circuit model and representative faradaic signal. Rs, solution resistance; Rct, charge transfer resistance; C, capacitance; W, Warburg impedance). | |
Cyclic voltammograms of each electrode were also recorded in 10.0 mmol L−1 [Fe(CN)6]3−/4− containing 0.10 M KCl (Fig. 1A). It can be seen that the anodic (+0.288 V) and cathodic (+0.156 V) peaks of the Fe3+/Fe2+ redox couple recorded with the bare PGE were clear and sharp. The anodic peak potentials of the QD modified electrodes were shifted to more positive values (+0.350 V and +0.355 V for the CdS/PGE and CdS–ZnS/MAA/PGE respectively), while the cathodic peak potentials were shifted to more negative values (+0.140 V for both the CdS/PGE and CdS–ZnS/MAA/PGE). Also, the peak currents of each electrode were decreased compared with the bare PGE. The shifting of the peak potentials can be attributed to the semiconductor surface properties of QDs.
The surface morphologies of the bare pretreated PGE, CdS/MAA/PGE and CdS–ZnS/MAA/PGE were also examined with scanning electron microscopy (SEM). Fig. 2B and C show SEM images of the CdS/MAA/PGE and CdS–ZnS/MAA/PGE, respectively, which are different from that of the pretreated PGE (Fig. 2A). A generally porous structure was observed from the SEM images of the CdS/MAA/PGE and CdS–ZnS/MAA/PGE, and a number of spongy sites were evident for the formation of the QDs. Although the ZnS–CdS was coagulated, interpretable results were obtained for the glucose biosensor tests.
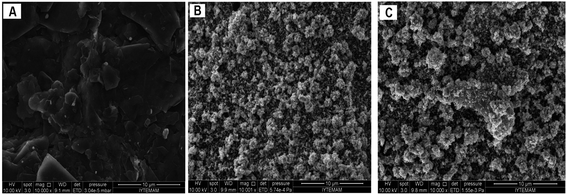 |
| Fig. 2 SEM images of the (A) bare PGE, (B) CdS/MAA/PGE, and (C) CdS–ZnS/MAA/PGE. | |
Oxidation of NADH at the CdS–ZnS/MAA/PGE
Before obtaining the response of the enzyme immobilized electrode in the biosensing of glucose, electrochemical oxidation of NADH at the QD modified PGE was investigated, due to its significance both as a cofactor for the dehydrogenase enzyme and as having a role in the electron transfer chain in biological systems, and also due to the need to develop a biosensor dependent on the NAD+/NADH redox couple and dehydrogenase enzymes. To check the electrochemical characteristics of the modified electrode toward the oxidation of NADH, cyclic voltammograms of the bare PGE and QD modified PGE were recorded with/without irradiation of the electrode surface in the absence and presence of 2.0 mM NADH (Fig. S1 and S2†). In the cyclic voltammograms of the pretreated PGE in the absence of NADH, no peaks were observed and irradiation of the electrode surface did not affect the voltammogram (inset of Fig. S1†). However, the cyclic voltammogram of the CdS–ZnS/PGE in pH 7.0 PBS changed with irradiation of the electrode surface (inset of Fig. S2†). Moreover, NADH was oxidized at about +520 mV (Fig. S1-1†) and +500 mV (Fig. S2-1†) at the bare PGE and CdS–ZnS/PGE, respectively. While a small increment in the oxidation peak current of NADH was observed with irradiation of the electrode surface (Fig. S1-2†) for the bare PGE, the peak current increased significantly (approximately from 16 μA (without light) to 58 μA (under irradiation, Fig. S2-2†)) for the CdS–ZnS/PGE. Therefore it can be said that the CdS–ZnS/PGE showed photoelectrocatalytic effects in the oxidation of NADH, due to an increase of the peak current, even though acceptable shifting of the oxidation potential was not observed.
In order to obtain the best amperometric and photoamperometric response of the CdS–ZnS/PGE towards NADH using a FIA system, the effect of the applied potential and flow rate on the current of 0.10 mM NADH was investigated by recording current–time curves. The results are presented in Fig. S3 to S5† with plots of peak current versus applied potential and flow rate. From the optimization studies, the most suitable applied potential, flow rate, sample volume, and transmission tube length were determined as +600 mV vs. Ag/AgCl, 1.75 mL min−1, 100 μL, and 10 cm, respectively. Plots of both the amperometric and photoamperometric current vs. various concentrations of NADH were recorded using the CdS–ZnS/PGE using these optimum conditions. Fig. S6† shows the current–time curves for the amperometric and photoamperometric FIA responses to various concentrations of NADH. Although the peak current increased depending on the NADH concentration for both the amperometric and the photoamperometric methods, the responses from the photoamperometric method were higher than that from the amperometric for all concentrations. The inset of Fig. S7† shows a plot of catalytic current vs. NADH concentration. From this figure, a linear relationship between the NADH concentration and the peak current was obtained over the concentration range 8.0 × 10−7 to 8.0 × 10−5 M using the amperometric and also the photoamperometric FIA method, with the CdS–ZnS/PGE. The linearity of these methods is described by the equation I (μA) = 21.11C (mM) + 0.075, R2 = 0.9997 and I (μA) = 38.99C (mM) + 0.058, R2 = 0.9991 for the amperometric and photoamperometric studies, respectively, where I is the peak current, C is the NADH concentration, and R is the regression coefficient. If these equations are compared in terms of their slopes, it is obvious that the sensitivity of the photoelectrocatalytic FIA procedure is better than that of the amperometric method and the extent of the improvement is about two fold.
Biosensing of glucose at the GDH/CdS–ZnS/MAA/PGE
Firstly, the electrochemical and photoelectrochemical responses of the dehydrogenase immobilized PGE (GDH/PGE without CdS–ZnS) toward glucose were investigated using cyclic voltammetry. Cyclic voltammograms of the GDH/PGE were recorded using 0.10 M PBS (pH 7.0) containing 0.10 M KCl and 10.0 mM NAD+ in the absence and in the presence of 40 mM glucose at a 20 mV s−1 scan rate (Fig. 3A). In the first voltammogram (Fig. 3A-a), an irreversible peak was observed at about 720 mV for the GDH/PGE, which was attributed to oxidation of enzymatically produced NADH to NAD+ (reactions 1 and 2). When the electrode surface was irradiated with the light source, the peak current increased a little (Fig. 3A-b).
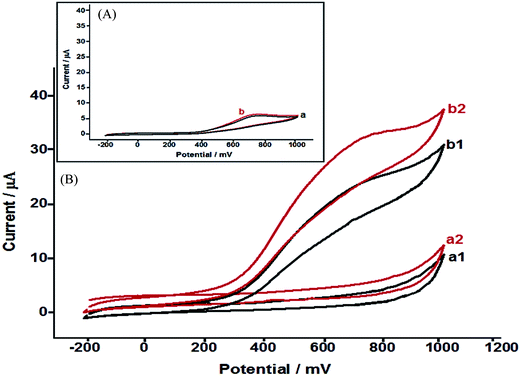 |
| Fig. 3 Cyclic voltammograms of the GDH/CdS–ZnS/MAA/PGE in the absence (a1 and a2) and in the presence of 40 mM glucose (b1 and b2). (a1) and (b1): without light, (a2) and (b2): with light. (Inset: cyclic voltammograms of the GDH/PGE in the presence of 40 mM glucose (a): without light, (b): with light). Supporting electrolyte: 0.10 M PBS (pH 7.0) containing 0.1 M KCl and 10.0 mM NAD+, scan rate: 20 mV s−1. | |
Cyclic voltammograms of the GDH/CdS–ZnS/MAA/PGE in the absence and in the presence of 40 mM glucose are also shown in Fig. 3. It can be seen that no peak was observed (Fig. 3B-a1) in the absence of glucose, however the capacitive current was changed very little by irradiation of the electrode surface (Fig. 3B-a2). In the presence of 40 mM glucose, oxidation of the NADH, which was formed from enzymatic reactions between NAD+ and glucose catalyzed by GDH, was observed at about 720 mV (Fig. 3B-b1). When the surface of the GDH/CdS–ZnS/MAA/PGE was irradiated (Fig. 3B-b2), the irreversible peak current was significantly increased. These results show that the GDH/CdS–ZnS/PGE can be successfully used for photoelectrochemical biosensing of glucose, due to an increase of the oxidation peak current under irradiation of the electrode surface, even though acceptable shifting of the oxidation potential was not observed.
After the cyclic voltammetric studies, photoelectrochemical biosensing of glucose was also investigated using an amperometric technique and a FIA system. In order to obtain the best amperometric and photoamperometric response of the GDH/CdS–ZnS/MAA/PGE toward glucose in the FIA system, the effect of the applied potential and flow rate on the current for 0.5 mM glucose containing 10.0 mM NAD+ and 1.0 M KCl was investigated by recording the diagrams that are presented in Fig. S8 to S10† and plots of peak current versus applied potential and flow rate. The best currents for electrocatalytic and photoelectrocatalytic oxidation of NADH produced by enzymatic reactions of glucose in the FIA system were found at about 800 mV. In addition, the current values obtained from the photoamperometric method were about 50 to 40% higher than that obtained from the amperometric method. Thus an applied potential of 800 mV was selected as the optimum potential value. The maximum peak current was observed at a flow rate of 0.6 mL min−1, since the biosensors and photoelectrochemical biosensors had enough time for the occurrence of the enzymatic reaction and also photoexcitation of the mediator at a low flow rate. The peak current decreased on increasing the flow rate to more than 0.6 mL min−1. Thus, a flow rate of 0.6 mL min−1 was selected as the optimum flow rate even though the sample frequency is very low.
To establish that a reliable analytical response could be achieved for glucose, under the optimized conditions using a GDH/CdS–ZnS/MAA/PGE, a calibration study was carried out over a range of 0.2 mM to 30 mM glucose concentration, with two injections of each concentration being made via a 100 μL sample loop. Fig. 4 shows the diagrams for the amperometric and photoamperometric FI responses to the various concentrations of glucose. Although the peak current increased depending on the glucose concentration for both the amperometric and the photoamperometric methods, the responses of the photoamperometric method were higher than those of the amperometric for all concentrations.
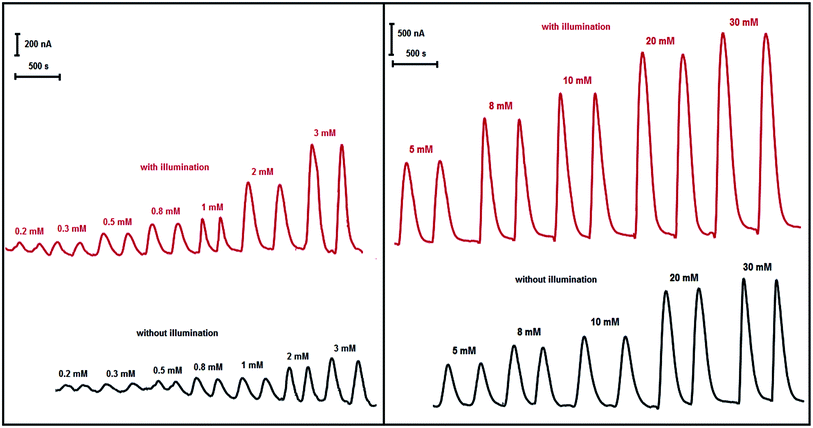 |
| Fig. 4 Current–time diagrams using glucose of different concentrations with the GDH/CdS–ZnS/MAA/PGE and the FIA system for the amperometric and photoamperometric methods. (Carrier stream: 0.1 M PBS (pH 7.0) containing 1.0 M KCl and 10 mM NAD+, applied potential: +0.8 V; flow rate: 0.6 mL min−1, sample loop: 100 μL; transmission tubing length: 10 cm). | |
Fig. 5A shows a plot of catalytic current versus glucose concentration. From this figure, a linear relationship between the glucose concentration and the peak current was obtained over the concentration range from 0.2 to 8.0 mM glucose for both the photoamperometric and amperometric FIA method with the glucose biosensor (Fig. 5B). The linearity of these methods is described by the equations I (μA) = 0.118C (mM) + 0.052, R2 = 0.9923, and I (μA) = 0.245C (mM) + 0.089, R2 = 0.9971 for the amperometric and photoamperometric studies, respectively, where I is the peak current and C is the concentration of glucose. If these equations are compared in terms of their slopes, it is clear that the sensitivity of the photoelectrocatalytic FIA procedure is better than that of the amperometric method and the extent of the improvement is about 2.0 fold.
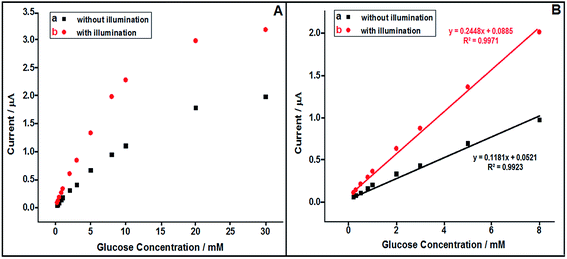 |
| Fig. 5 (A) The curve of the catalytic current vs. the concentration of the injected glucose solution for the amperometric (a) and photoamperometric (b) methods.(B) Calibration curve of peak current versus glucose concentration. | |
The limit of detection (LOD) was calculated as 0.09 mM and 0.05 mM glucose for the amperometric and photoamperometric glucose biosensors, respectively, based on 3sb/m where sb is the standard deviation of the blank response and m is the slope of the calibration curve.
The precision of the electrochemical and photoelectrochemical biosensors was investigated by performing 7 repeat injections of a 0.5 mM glucose solution. The RSD for the electrochemical and photoelectrochemical biosensors was calculated to be 3.5% and 4.5% respectively. These results indicate that the GDH/CdS–ZnS/MAA/PGE has a very good repeatability for electrochemical and photoelectrochemical biosensing of glucose.
Interference studies
The effects of common interfering species such as ascorbic acid (AA), uric acid (UA), dopamine (DA), L-cysteine (L-Cyst), galactose, saccharose and glutamic acid, which may affect the response of the electrode, were investigated. The amperometric responses of the GDH/CdS–ZnS/MAA/PGE using the FIA system under the optimized conditions toward glucose (0.5 mM) were recorded in the presence of these interfering species. The experimental results showed that no significant change in the oxidation peak current could be observed for saccharose, glutamic acid (one hundred fold higher than glucose) and galactose (ten fold higher than glucose) (data not shown), which shows a good selectivity of the enzyme electrode in the presence of other monosaccharides and also disaccharides. However, equimolar amounts of AA, DA, UA and L-Cyst to the glucose concentration showed serious interference (increase of the oxidation current), because their oxidation potentials are close to that of NADH. It has been reported that the interference of AA can be eliminated and the biosensor selectivity towards glucose enhanced by using Nafion as an outer layer coated on the biosensor surface33,34 or by using ascorbate oxidase. Another possible way of removing the interfering effect of all these compounds is that a very small amount of lead(IV) acetate as an oxidizing agent can be added to the Nafion layer, which causes preoxidation reactions of these interfering compounds before they reach the electrode surface.35
Real sample analysis
One real sample (a commercial dextrose solution) and spiked serum samples were used for the determination of glucose at the GDH/CdS–ZnS/MAA/PGE as described in the literature.36,37 For this, 250 μL samples of the serum were diluted to 5.0 mL with 0.1 M PBS (pH 7.0) containing 10 mM NAD+ and 1.0 M KCl. Glucose detection was performed by spiking a known volume and concentration of a glucose standard solution into the diluted serum samples in order to obtain various target concentrations, and then by measuring the amperometric and photoamperometric response of the electrode using the FIA system. For determination of the glucose in the commercial dextrose solution (containing 5% glucose), it was diluted 555 times (about 0.5 mM glucose) with 0.1 M PBS (pH 7.0) containing 10 mM NAD+ and 1.0 M KCl. Glucose detection was also performed for this sample as described for the spiked serum samples. The results of the recovery tests are given in Table 1. It can be seen that acceptable recoveries were obtained for the spiked glucose in serum plasma and the commercial dextrose solution samples.
Table 1 The results of glucose determination for spiked serum samples (n = 3) and a dextrose solution
Sample |
Glucose (mM) |
FIA amperometric |
FIA photoamperometric |
Found (mM) |
Recovery |
RSD% |
Found (mM) |
Recovery |
RSD% |
Spiked serum |
0.25 |
0.24 ± 0.01 |
93 |
4.1 |
0.26 ± 0.01 |
101 |
3.7 |
0.50 |
0.46 ± 0.05 |
91 |
9.4 |
0.51 ± 0.02 |
98 |
3.9 |
0.75 |
0.77 ± 0.04 |
101 |
5.4 |
0.74 ± 0.015 |
98 |
2.0 |
Dextrose solution |
As label claimed (5% = 277.5 mM) |
255.5 ± 5.5 |
— |
— |
283 ± 5.4 |
— |
— |
Experimental
Chemicals
Glucose dehydrogenase (from Pseudomonas sp. 338.7 U mg−1), β-nicotinamide adenine dinucleotide sodium salt (from Saccharomyces cerevisiae, C21H26N7NaO14P2, NaNAD+, MW: 685.41 g mol−1), bovine serum albumin (BSA), glutaraldehyde (GA, d: 1.061 g mL−1, MW: 100.12 g mol−1, 25% w/w in water), D-(+)-glucose, reduced β-nicotinamide adenine dinucleotide disodium salt (MW: 709.40 g mol−1 C21H27N7Na2O14P2, NADHNa2), KCl, H3PO4, NaH2PO4, Na2HPO4, CH3COOH, NaOH, HCl, mercapto acetic acid (MAA), Na2S2O3, ZnCl2, the sodium salt of EDTA and CdCl2·2.5H2O were supplied by Merck, Sigma or Carlo Erba.
Stock solutions of glucose (1.0 M) and NAD+ (0.01 M) were freshly prepared with deionized water daily. A stock solution of NADH (5.0 × 10−2 M) was prepared daily using a pH 7.0 phosphate buffer solution (PBS). The concentration of NADH in the diluted solutions was checked using a Perkin Elmer Lambda 35 UV-vis spectrometer. The absorbance of the solution was monitored at 340 nm, considering a molar extinction coefficient of 6600 cm−1 M−1.38
Apparatus
All the solutions were prepared with ultrapure water from an Elga Option Q7B water purification system (18.2 MΩ cm). All electrochemical experiments were carried out using an Autolab PGSTAT 128N potentiostat/galvanostat equipped with a FRA2 frequency response analyzer. A traditional three-electrode system was used with a platinum wire as the counter electrode, Ag/AgCl/KCl(sat.) as the reference electrode, and a PGE as the working electrode.19–24 A pencil lead with a diameter of 0.5 mm (Ultra-Polymer, 2B) and a total length of 60 mm (Tombow, Japan), and a mechanical pencil model T 0.5 (Rotring, Germany), which was used as the holder for the pencil lead, were purchased from a local bookstore. Electrical contact to the lead was obtained by wrapping a metallic wire round the metallic part of the holder. For each measurement, a total of 10 mm of lead (area of about 15.9 mm2) was immersed into the solution. Cyclic voltammograms and electrochemical impedance curves were recorded in a static cell while the amperometric experiments were performed in a FIA system. A new home-made photoelectrochemical flow cell for only PGEs, which was constructed from TEFLON, was used.31 The depth of the pencil lead in this flow cell was arranged as 10 mm. The pH values of the solutions were adjusted using a HI 221 Hanna pH-meter with a combined glass electrode (Hanna Instrument HI-1332). In order to perform the FIA experiments, an eight-channel Ismatec, Ecoline peristaltic pump with polyethylene tubing (0.75 mm i.d.) and a Rheodyne 8125 sample injection valve were used. In order to perform the photoelectrochemical experiments, a fiber optic illuminator with a 250 W halogen bulb and a Foi-5 Light Guide (Titan Tool Supply Inc., USA) was used to illuminate the electrode surface.
Preparation of the modified electrodes and the glucose biosensor
After the surface of the PGE was pretreated by applying a potential of +1.40 V for 60 s in the pH 7.0 PBS, ZnS–CdS was constructed on the PGE using an electrochemical precipitation method according to previous reports with small modifications.39,40 Briefly, the pretreated PGE was immersed 10.0 mm into pH 6.0 PBS containing 15.0 mmol L−1 CdCl2, 8.0 mmol L−1 Na2S2O3, 8.0 mmol L−1 EDTA and 0.05 mmol L−1 MAA, which was used for minimizing coagulation of the QDs,41 for electrochemical deposition with a deposition potential of −1.00 V vs. Ag/AgCl for 1000 s at 30 °C. After the CdS was prepared on the PGE, similarly, ZnS was prepared on the CdS/PGE using the same conditions but with 15 mmol L−1 ZnCl2. The modified electrode was designated as CdS–ZnS/MAA/PGE.
The GDH enzyme was immobilized onto the modified PGE using a cross-linking procedure. Typically, 1.0% bovine serum albumin (BSA) was first mixed with GDH (80.0 mg mL−1 in PBS, pH 7.0) in a volume ratio of 1 to 1, and then 5 μL of this mixture was mixed with 4 μL of 20.0 mM glutaraldehyde (GA) as the cross linking reagent. The CdS–ZnS/MAA/PGE was immersed into the final solution for 1 h and then dried for 10 min at +4 °C. Finally, the obtained GDH/CdS–ZnS/MAA/PGE was stored at +4 °C.
Electrochemical procedure
In order to characterize the modified electrode, electrochemical impedance spectra of the bare and modified PGEs were recorded using pH 7.0 PBS containing 10.0 mM K3Fe(CN)6, 10.0 mM K4Fe(CN)6 and 0.10 M KCl at a formal potential of 180 mV with a frequency range of 10.000 to 0.05 Hz and a signal amplitude of 5 mV (Fig. 1). In addition, cyclic voltammograms were also recorded in pH 7.0 PBS containing 10.0 mM K3Fe(CN)6, 10.0 mM K4Fe(CN)6 and 0.10 M KCl between −0.5 and +0.8 V at 50 mV s−1. The surface morphologies of the bare and modified PGEs were also examined by recording their scanning electron microscopy (SEM) images.
Electrochemical and also photoelectrochemical biosensing of glucose at the GDH/CdS–ZnS/MAA/PGE were investigated using cyclic voltammetric techniques. Firstly, a cyclic voltammogram of the GDH/CdS–ZnS/MAA/PGE was recorded using 0.10 M PBS (pH 7.0) containing 10.0 mM NAD+ in the potential range between −200 and 1000 mV versus Ag/AgCl at a scan rate of 20 mV s−1 in the absence of glucose. To investigate the response of the biosensor towards glucose, cyclic voltammograms for the modified electrodes were recorded under the same conditions but in the presence of 40.0 mM glucose. Cyclic voltammograms were also recorded for a GDH immobilized bare PGE. Finally, photoelectrochemical biosensor studies were carried out under irradiation of the working electrode surface with a fiber optic illuminator with a 250 W halogen bulb.
Photoelectrochemical biosensor studies using the FIA system
Electrochemical and especially photoelectrochemical biosensor studies using the FIA system were performed using a home-made photoamperometric flow cell which was constructed for a PGE for the first time.31 In all FIA experiments, 0.10 M PBS (pH 7.0) containing 1.0 M KCl was used as the carrier solution. After the GDH/MAA/ZnS–CdS/PGE had been inserted into a flow cell, optimization studies for the GDH/CdS–ZnS/MAA/PGE were performed using the FIA system. After a steady-state background current was obtained under the optimum conditions (sample loop, flow rate, applied potential, length of tubing), glucose solutions of various concentrations containing 10.0 mM NAD+ were injected into the system (three successive injections) and current–time curves were recorded. Current–time curves were also recorded for the photoamperometric FIA study through irradiation of the electrode surface throughout the experiment. All the supporting electrolytes were deaerated by allowing highly pure argon to pass through them for 5 min before the electrochemical experiments.
In order to show the practical applicability of the proposed biosensor, a real sample (commercial dextrose solution including 5% glucose) and a spiked serum sample were selected for the determination of glucose as described in the literature.36,37
Conclusions
In this study, a constructed photoelectrochemical glucose biosensor dependent on the NAD+/NADH redox couple and GDH using a FIA system was proposed using an enzyme modified PGE. Although photoelectrochemical biosensors dependent on the NAD+/NADH redox couple–dehydrogenase enzymes have been reported,11–13,36,42 according to our search of the literature, the use of PGEs for photoelectrochemical biosensors using a FIA system had not yet been reported. The GDH/CdS–ZnS/MAA/PGE exhibited a good photoelectrocatalytic response for the detection of glucose and a linear relationship was obtained between 0.2 and 8.0 mM glucose with a detection limit of 0.05 mM. The sensitivity of the photoamperometric biosensor in the FIA system was improved by about two fold in comparison with that of the amperometric procedure. It can be concluded that the GDH/CdS–ZnS/PGE can be successfully used for photoelectrochemical biosensing of glucose due to an increasing of the oxidation peak current under irradiation of the electrode surface even though the oxidation potential of the enzymatically produced NADH is very high (about −800 mV) and an acceptable shifting of the oxidation potential was not observed. In addition, the PGE was successfully used for photoelectrochemical biosensing of glucose in a FIA system by using a new home-made photoelectrochemical flow cell for the first time. As a result, a novel, fast and facile sensor was reported in this study, which is expected to offer new prospects for the development of cheap, sensitive, selective, disposable and faster photoelectrochemical biosensors in the future.
Acknowledgements
We thank The Scientific and Technological Research Council of Turkey (TÜBİTAK) for financial support (project number: 112T375).
References
- G. M. Duran, A. M. Contento and A. Rios, Curr. Org. Chem., 2015, 19, 1134 CrossRef CAS.
- Z. Yue, F. Lisdat, W. J. Parak, S. G. Hickey, L. Tu, N. Sabir, D. Dorfs and N. C. Bigall, ACS Appl. Mater. Interfaces, 2013, 5, 2800 CAS.
- Q. Ma and X. G. Su, Analyst, 2011, 136, 4883 RSC.
- F. Wang and S. S. Hu, Microchim. Acta, 2009, 165, 1 CrossRef CAS.
- I. Willner, R. Baron and B. Willner, Biosens. Bioelectron., 2007, 22, 1841 CrossRef CAS PubMed.
- G. L. Wang, J. J. Xu and H. Y. Chen, Sci. China, Ser. B: Chem., 2009, 52, 1789 CrossRef CAS.
- Z. X. Zhan and C. Z. Zhao, Chin. J. Anal. Chem., 2013, 41, 436 CrossRef.
- G. L. Wang, J. J. Xu and H. Y. Chen, Biosens. Bioelectron., 2009, 24, 2494 CrossRef CAS PubMed.
- Y. Ho, A. P. Periasamy and S. M. Chen, Sens. Actuators, B, 2011, 156, 84 CrossRef CAS.
- K. Wang, J. Wu, Q. Liu, Y. Jin, J. Yan and J. Cai, Anal. Chim. Acta, 2012, 745, 131 CrossRef CAS PubMed.
- F. Jafari, A. Salimi and A. Navaee, Electroanalysis, 2014, 26, 1782 CrossRef CAS.
- F. Jafari, A. Salimi and A. Navaee, Electroanalysis, 2014, 26, 530 CrossRef CAS.
- K. Schubert, W. Khalid, Z. Yue, W. J. Parak and F. Lisdat, Langmuir, 2010, 26, 1395 CrossRef CAS PubMed.
- X. Q. Liu, R. Yan, J. Zhu, X. Huo and X. Wang, Electrochim. Acta, 2015, 173, 260 CrossRef CAS.
- X. Peng, M. C. Schlamp, A. V. Kadavanich and A. P. Alivisatos, J. Am. Chem. Soc., 1997, 119, 7019 CrossRef CAS.
- J. Li, Y. A. Wang, W. Z. Guo, J. C. Keay, T. D. Mishima, M. B. Johnson and X. Peng, J. Am. Chem. Soc., 2003, 125, 12567 CrossRef CAS PubMed.
- F. Huang, F. Wang, S. Feng, Y. Li, S. Li and Y. Li, J. Solid State Electrochem., 2013, 17, 1295 CrossRef CAS.
- J. Wang, A. N. Kawde and E. Sahlin, Analyst, 2000, 125, 5 RSC.
- Y. Dilgin, B. Ertek, B. Kizilkaya, D. G. Dilgin and H. I. Gokcel, Sci. Adv. Mater., 2012, 4, 920 CrossRef CAS.
- Y. Dilgin, B. Kızılkaya, B. Ertek, N. Eren and D. G. Dilgin, Talanta, 2012, 89, 490 CrossRef CAS PubMed.
- Y. Dilgin, B. Kizilkaya, D. G. Dilgin, H. İ. Gökçel and L. Gorton, Colloids Surf., B, 2013, 102, 816 CrossRef CAS PubMed.
- Y. Dilgin, B. Kızılkaya, B. Ertek, F. Işık and D. G. Dilgin, Sens. Actuators, B, 2012, 171–172, 223 CrossRef CAS.
- E. Dede, Ö. Sağlam and Y. Dilgin, Electrochim. Acta, 2014, 127, 20 CrossRef CAS.
- Z. O. Uygun and Y. Dilgin, Sens. Actuators, B, 2014, 188, 78 CrossRef.
- A. N. Kawde, M. Aziz, N. Baig and Y. Temerk, J. Electroanal. Chem., 2015, 740, 68 CrossRef CAS.
- S. Tajik, M. A. Taher, H. Beitollahi and M. Torkzadeh-Mahani, Talanta, 2015, 134, 60 CrossRef CAS PubMed.
- J. Zhu, X. Y. Wu, D. Shan, P. X. Yuan and X. J. Zhang, Talanta, 2014, 130, 96 CrossRef CAS PubMed.
- A. Ozcan and S. Ilkbas, Sens. Actuators, B, 2015, 215, 518 CrossRef CAS.
- K. K. Tadi, R. V. Motghare and V. Ganesh, Electroanalysis, 2014, 26, 2328 CrossRef CAS.
- E. Alipour, M. R. Majidi and O. Hoseindokht, J. Chin. Chem. Soc., 2015, 62, 461 CrossRef CAS.
- Ö. Sağlam, B. Kızılkaya, H. Uysal and Y. Dilgin, Talanta, 2016, 147, 315 CrossRef PubMed.
- I. I. Suni, Trends Anal. Chem., 2008, 27, 604 CrossRef CAS.
- D. M. Kim, M. Y. Kim, S. S. Reddy, J. Cho, C. H. Cho, S. Jung and Y. B. Shim, Anal. Chem., 2013, 85, 11643 CrossRef CAS PubMed.
- T. T. Baby, S. S. J. Aravind, T. Arockiadoss, R. B. Rakhi and S. Ramaprabhu, Sens. Actuators, B, 2010, 145, 71 CrossRef CAS.
- F. Pariente, F. Tobalina, G. Moreno, L. Hernandez, E. Lorenzo and H. D. Abruna, Anal. Chem., 1997, 69, 4065 CrossRef CAS PubMed.
- D. G. Dilgin and H. İ. Gökçel, Anal. Methods, 2015, 7, 990 RSC.
- A. M. Vinu Mohan, K. K. Aswini, A. Maria Starvin and V. M. Biju, Anal. Methods, 2013, 5, 1764 RSC.
- L. T. Kubota and L. Gorton, Electroanalysis, 1999, 11, 719 CrossRef CAS.
- C. Lu, X.-F. Wang, J. J. Xu and H. Y. Chen, Electrochem. Commun., 2008, 10, 1530 CrossRef CAS.
- Z. Qian, H. J. Bai, G. L. Wang, J. J. Xu and H. Y. Chen, Biosens. Bioelectron., 2010, 25, 2045 CrossRef CAS PubMed.
- A. Salimi, R. Rahmatpanah, R. Hallaj and M. Roushani, Electrochim. Acta, 2013, 95, 60 CrossRef CAS.
- L. Deng, Y. Wang, L. Shang, D. Wen and F. Wang, Biosens. Bioelectron., 2008, 24, 951 CrossRef CAS PubMed.
Footnote |
† Electronic supplementary information (ESI) available. See DOI: 10.1039/c5ra25673a |
|
This journal is © The Royal Society of Chemistry 2016 |