DOI:
10.1039/C5RA21975B
(Paper)
RSC Adv., 2016,
6, 7495-7503
Cyclopentyl methyl ether as a green solvent for reversible-addition fragmentation chain transfer and nitroxide-mediated polymerizations†
Received
20th October 2015
, Accepted 25th December 2015
First published on 6th January 2016
Abstract
Cyclopentyl methyl ether (CPME) was successfully used as an environmentally friendly alternative to the regularly employed organic solvents (e.g., tetrahydrofuran (THF), dimethyl sulfoxide (DMSO), dichloromethane (DCM) and dimethylformamide (DMF)) for the reversible-addition fragmentation chain transfer (RAFT) polymerization and nitroxide-mediated polymerization (NMP) of vinyl chloride (VC) and styrene (S). Methyl acrylate (MA) and vinyl acetate (VAc) were also successfully polymerized via RAFT using CPME. The kinetic data showed a linear increase of the molecular weight with the monomer conversion for both polymerization methods. The kappp data obtained in CPME were in the range of the values reported for THF, DMSO, DCM and DMF, while the final conversions were higher. The polymer samples were comprehensively characterized by 1H nuclear magnetic resonance spectroscopy (1H-NMR), 31P-NMR, matrix-assisted laser desorption ionization time-of-flight mass spectroscopy (MALDI-TOF-MS) and size exclusion chromatography (SEC). The “livingness” of the PVC macroinitiators prepared by RAFT and NMP were confirmed by chain-end characterization and successful reinitiation experiments. The data presented here prove that CPME is an excellent green substitute to avoid the use of toxic solvents for RAFT and NMP.
Introduction
Reversible deactivation radical polymerization (RDRP) has revolutionized the field of macromolecular synthesis. On this matter, it is now possible to synthesize tailor made (co)polymers with controlled molecular weight, topology, architecture and functionalities.1–6 The most popular RDRP methods are: atom transfer radical polymerization (ATRP),5–7 nitroxide-mediated polymerization (NMP)2,8 and reversible addition-fragmentation transfer polymerization (RAFT).3,4,9–12 The intense research efforts of the scientific community during the last two decades on their mechanistic understanding2,10,13,14 enabled the expansion of the range of monomers to be controlled and the establishment of new reaction conditions that can be implemented in large scale production. Although polymerizations in bulk and in dispersed media have been widely studied, solution polymerizations have only received a very little attention despite their numerous advantages (e.g., low viscosity of the reaction medium, possibility to dilute the reaction medium and avoid the gel effect, broad variety of solvents, etc.).15–17 Even though water is the ideal solvent in terms of nontoxicity, very few examples of monomers/polymers are water-soluble. Therefore, alternative “green” organic solvents are highly desirable. The continuous search to find eco-friendly solvents for RDRP methods resulted in the use of water,18–21 water/alcohol mixtures,22–28 ionic liquids.29–36 CPME presents several important features that are particularly relevant. It is highly hydrophobic and presents a good stability in acidic and basic conditions.36 Moreover, it leads to a low formation of peroxides as by-products, results in negative skin sensitization,37 gives no genotoxicity or mutagenicity38 and is approved by the Toxic Substances Control Act (TSCA) and the European List of Notified Chemical Substances (ELINCS).36,39 CPME is therefore very appealing to circumvent the toxicological drawbacks commonly associated with the use of dimethyl sulfoxide (DMSO), dimethylformamide (DMF), dichloromethane (DCM) and tetrahydrofuran (THF), which are very effective solvents for homogeneous RDRP methods of hydrophobic monomers such as VC15,40–43 and S.39,44–46 Here, we demonstrate that CPME is a suitable (nearly universal) solvent to perform RAFT and NMP of VC and S as well as RAFT of MA and VAc.
Experimental section
Materials
VC (99.9%) was kindly supplied by CIRES Lda, Portugal. MA (Acros; 99% stabilized), S (Sigma-Aldrich; +99%) and VAc (Sigma-Aldrich; +99%) were passed over a basic alumina column before use to remove the radical inhibitors. The BlocBuilder alkoxyamine (99%) was kindly supplied by Arkema. Cyanomethyl methyl(phenyl)carbamodithioate (CMPCD) (Sigma-Aldrich, 98%), 2-(dodecylthiocarbonothioylthio)-2-methylpropionic acid (DDMAT) (Sigma-Aldrich, 98%), Trigonox 187-W40 (40% water and methanol emulsion of diisobutyryl peroxide – DIBPO), deuterated tetrahydrofuran (d8-THF) (Euriso-top; 99.5%), deuterated chloroform (CDCl3) (Euriso-top; +1% tetramethylsilane (TMS)), 2-(4-hydroxyphenylazo)benzoic acid (HABA) (Sigma-Aldrich, 99.5%), CPME (Sigma-Aldrich, inhibitor-free, anhydrous, +99.9%), methanol (Labsolve, 99.5%), hexane (Fisher Chemical, 95%), and polystyrene (PS) standards (Polymer Laboratories) were used as received. Azobisisobutyronitrile (AIBN) (Fluka, 98%) was recrystallized three times from ethanol before use. High-performance liquid chromatography (HPLC) grade THF (Panreac) was filtered (0.2 μm filter) under reduced pressure before use.
Techniques
400 MHz 1H-NMR spectra and 31P-NMR of samples were recorded on a Bruker Avance III 400 MHz spectrometer, with a 5 mm TIX triple resonance detection probe, in d8-THF and CDCl3 with tetramethylsilane (TMS) and diethyl phosphite (DEP) as an internal standard respectively.
The chromatographic parameters of the samples were determined using a size exclusion chromatography set-up from Viscotek (Viscotek TDAmax) equipped with a differential viscometer (DV) and right-angle laser-light scattering (RALLS, Viscotek), low-angle laser-light scattering (LALLS, Viscotek) and refractive index (RI) detectors. The column set consisted of a PL 10 mm guard column (50 × 7.5 mm2) followed by one Viscotek T2000 column (6 μm), one MIXED-E PLgel column (3 μm) and one MIXED-C PLgel column (5 μm). A dual piston pump was set with a flow rate of 1 mL min−1. The eluent (THF) was previously filtered through a 0.2 μm filter. The system was also equipped with an on-line degasser. The analyses were performed at 30 °C using an Elder CH-150 heater. Before injection (100 μL), the samples were filtered through a polytetrafluoroethylene (PTFE) membrane with 0.2 μm pore. The system was calibrated with narrow PS standards. The dn/dc value was determined as 0.105 for PVC and 0.185 for PS. Molecular weight (MSECn) and dispersity (Đ = Mw/Mn) of synthesized polymers were determined by triple detection calibration using the OmniSEC software version 4.6.1.354.
For the MALDI-TOF-MS analysis, the PVC samples were dissolved in THF at a concentration of 10 mg mL−1. HABA (0.05 M in THF) was used as matrix. The dried-droplet sample preparation technique was used to obtain 1/1 ratio (sample/matrix); an aliquot of 1 μL of each sample was directly spotted on the MTP AnchorChip TM 600/384 TF MALDI target, Bruker Daltonik (Bremen Germany) and, before the sample dry, 1 μL of matrix solution in THF was added and allowed to dry at room temperature, to allow matrix crystallization. External mass calibration was performed with a peptide calibration standard (PSCII) for the range 700–3000 (9 mass calibration points), 0.5 μL of the calibration solution and matrix previously mixed in an Eppendorf tube (1/2, v/v) were applied directly on the target and allowed to dry at room temperature. Mass spectra were recorded using an Autoflex III smartbeam1 MALDI-TOF-MS mass spectrometer Bruker Daltonik (Bremen, Germany) operating in the linear and reflectron positive ion mode. Ions were formed upon irradiation by a smartbeam1 laser using a frequency of 200 Hz. Each mass spectrum was produced by averaging 2500 laser shots collected across the whole sample spot surface by screening in the range m/z 400–10
000. The laser irradiance was set to 35–40% (relative scale 0–100) arbitrary units according to the corresponding threshold required for the applied matrix systems.
Procedures
The VC polymerizations were carried out in a 50 mL glass high-pressure tube equipped with a magnetic stir bar. In the kinetic studies each point represents a single experiment.
Typical procedure for the RAFT polymerization of VC in CPME at 42 °C with [VC]0/[CMPCD]0/[Trigonox]0 = 250/1/0.2. A 50 mL Ace Glass 8645#15 pressure tube, equipped with a bushing and a plunge valve, was charged with a mixture of CMPCD (66 mg, 0.29 mmol), Trigonox 187 W40 (25 mg, 0.058 mmol) and CPME (5.0 mL) (previously bubbled with nitrogen for 5 min). The precondensed VC (5 mL, 73 mmol) was added to the tube. The exact amount of VC was determined gravimetrically. The tube was sealed, submerged in liquid nitrogen and degassed through the plunger valve by applying reduced pressure and filling the tube with nitrogen about 20 times. The valve was closed and the tube reactor was placed in a water bath at 42 °C ± 0.5 °C under stirring (700 rpm). After 24 h, the reaction was stopped by plunging the tube into ice water. The tube was slowly opened, the excess VC was distilled, and the mixture was precipitated into 250 mL of methanol. The polymer was separated by filtration and dried in a vacuum oven until constant weight, yielding 3.66 g (69.8%) of PVC (MSECn = 11
500, Đ = 1.51).
Typical procedure for the RAFT polymerization of S in CPME at 60 °C with [S]0/[DDMAT]0/[AIBN]0 = 222/1/0.5. A mixture of DDMAT (59 mg, 0.16 mmol), AIBN (13 mg, 0.08 mmol) and CPME (2.0 mL) (previously bubbled with nitrogen for 5 min) was placed in a Schlenk tube reactor. S (4.0 mL, 35 mmol) was added to the reactor that was sealed, by using a glass stopper, and frozen in liquid nitrogen. The Schlenk tube reactor containing the reaction mixture was deoxygenated with four freeze–vacuum–thaw cycles and purged with nitrogen. The reactor was placed in an oil bath at 60 °C with stirring (700 rpm). During the polymerization, different reaction mixture samples were collected by using an airtight syringe and purging the side arm of the Schlenk tube reactor with nitrogen. The samples were analyzed by 1H NMR spectroscopy to determine the monomer conversion and by SEC, to determine the MSECn and Đ of the polymers (trx = 49 h, conv. = 84%, MSECn = 16
000, Đ = 1.09).
Typical procedure for the “one-pot” chain extension experiment from a CTA-terminated PVC. A 50 mL Ace Glass 8645#15 pressure tube, equipped with a bushing and a plunger valve, was charged with a mixture CMPCD (99 mg, 0.44 mmol), Trigonox 187 W40 (38 mg, 0.087 mmol) and CPME (3.0 mL) (previously bubbled with nitrogen for 5 min). The precondensed VC (3.0 mL, 44 mmol) was added to the tube. The exact amount of VC was determined gravimetrically. The tube was sealed, submerged in liquid nitrogen and degassed through the plunger valve by applying reduced pressure and filling the tube with nitrogen about 20 times. The valve was closed, and the tube reactor was placed in a water bath at 42 °C under stirring (700 rpm). After 5 h, the reaction was stopped by plunging the tube into ice water. The tube was slowly opened and the excess VC was distilled. The monomer conversion were determined gravimetrically (58.6%), and the MSECn = 4200 and Đ = 1.54 were determined by SEC. CPME (18.0 mL) (previously bubbled with nitrogen for 5 min), Trigonox 187 W40 (31 mg, 0.07 mmol) and the precondensed VC (18.0 mL, 262 mmol) were added in the medium without any purification of the previously obtained PVC-CTA macroCTA. The tube was sealed, submerged in liquid nitrogen and degassed through the plunger valve by applying reduced pressure and filling the tube with nitrogen about 20 times. The valve was closed, and the tube reactor was placed in a water bath at 42 °C under stirring (700 rpm). The reaction was stopped after 48 h by plunging the tube into ice water. The tube was slowly opened and the excess VC was distilled. The monomer conversion were determined gravimetrically (41.8%), and the MSECn = 17
300 and Đ = 1.53 of the resulting extended PVC-b-PVC were determined by SEC.
Typical procedure for the NMP of VC in CPME at 42 °C with [VC]0/[BlocBuilder]0 = 250/1. A 50 mL Ace Glass 8645#15 pressure tube, equipped with a bushing and a plunge valve, was charged with a mixture of BlocBuilder alkoxyamine (111 mg, 0.29 mmol) and CPME (5.0 mL) (previously bubbled with nitrogen for 5 min). The precondensed VC (5 mL, 73 mmol) was added to the tube. The exact amount of VC was determined gravimetrically. The tube was sealed, submerged in liquid nitrogen and degassed through the plunger valve by applying reduced pressure and filling the tube with nitrogen about 20 times. The valve was closed and the tube reactor was placed in a water bath at 42 °C ± 0.5 °C under stirring (700 rpm). After 24 h, the reaction was stopped by plunging the tube into ice water. The tube was slowly opened, the excess VC was distilled, and the mixture was precipitated into 250 mL of methanol. The polymer was separated by filtration and dried in a vacuum oven until constant weight, yielding 3.30 g (63.1%) of PVC (MSECn = 12
000, Đ = 1.54).
Typical procedure for the NMP of S in CPME at 80 °C with [S]0/[BlocBuilder]0 = 222/1. A mixture of BlocBuilder alkoxyamine (60 mg, 0.16 mmol) and CPME (2.0 mL) (previously bubbled with nitrogen for 5 min) was placed in a Schlenk tube reactor. S (4.0 mL, 35 mmol) was added to the reactor that was sealed, by using a glass stopper, and frozen in liquid nitrogen. The Schlenk tube reactor containing the reaction mixture was deoxygenated with four freeze–vacuum–thaw cycles and purged with nitrogen. The reactor was placed in an oil bath at 80 °C with stirring (700 rpm). During the polymerization, different reaction mixture samples were collected by using an airtight syringe and purging the side arm of the Schlenk tube reactor with nitrogen. The samples were analyzed by 1H NMR spectroscopy to determine the monomer conversion and by SEC, to determine the MSECn and Đ of the polymers (trx = 166 h, conv. = 71%, MSECn = 13
500, Đ = 1.09).
Typical procedure for the “one-pot” chain extension experiment from SG1-terminated PVC. A 50 mL Ace Glass 8645#15 pressure tube, equipped with a bushing and a plunger valve, was charged with a mixture of BlocBuilder alkoxyamine (167 mg; 0.44 mmol) and CPME (3.0 mL) (previously bubbled with nitrogen for 5 min). The precondensed VC (3.0 mL, 43.7 mmol) was added to the tube. The exact amount of VC was determined gravimetrically. The tube was sealed, submerged in liquid nitrogen and degassed through the plunger valve by applying reduced pressure and filling the tube with nitrogen about 20 times. The valve was closed, and the tube reactor was placed in a water bath at 42 °C under stirring (700 rpm). After 10 h, the reaction was stopped by plunging the tube into ice water. The tube was slowly opened and the excess VC was distilled. The monomer conversion were determined gravimetrically (52.2%), and the MSECn = 4300 and Đ = 1.55 were determined by SEC. CPME (18.0 mL) (previously bubbled with nitrogen for 5 min) and the precondensed VC (18.0 mL, 262 mmol) were added in the medium without any purification of the previously obtained PVC-SG1 macroinitiator. The tube was sealed, submerged in liquid nitrogen and degassed through the plunger valve by applying reduced pressure and filling the tube with nitrogen about 20 times. The valve was closed, and the tube reactor was placed in a water bath at 42 °C under stirring (700 rpm). The reaction was stopped after 48 h by plunging the tube into ice water. The tube was slowly opened and the excess VC was distilled. The monomer conversion were determined gravimetrically (47.2%), and the MSECn = 23
600 and Đ = 1.61 of the resulting extended PVC-b-PVC were determined by SEC.
Results and discussion
In a previous publication from our group, CPME was used for the first time in the SARA-ATRP39 using CuBr2/ligands (e.g., Me6TREN, TPMA, bpy, PMDETA and TREN). The poor solubility of metal complexes in CPME required the addition of co-solvents (e.g., H2O and EtOH). For RAFT and NMP, it was considered to use CMPE as the only polymerization solvent.
RAFT polymerization in CPME
Preliminary RAFT polymerization experiments were conducted using MA with DDMAT as chain transfer agent (CTA) in CPME for a DP of 222. The results presented in Fig. 1 and Table 1 (entry 1) show a first-order kinetics with respect to MA, a good agreement between theoretical and determined molecular weights and low Đ values below 1.1, indicating an excellent control of the polymerization even up to high conversions (∼95%). These observations allowed us to conclude that CPME is a solvent compatible with RAFT systems.
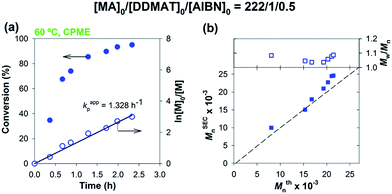 |
| Fig. 1 RAFT polymerization of MA in CPME at 60 °C mediated by DDMAT using AIBN as conventional initiator. (a) Conversion and ln[M]0/[M] vs. time. (b) Number-average molecular weight (MSECn) and dispersity (Mw/Mn) vs. theoretical number-average molecular weight (Mthn). Reaction conditions: [MA]0/[DDMAT]0/[AIBN]0 = 222/1/0.5; [MA]0/[CPME] = 2/1 (v/v). | |
Table 1 Kinetic parameters obtained for RAFT polymerizations in CPME with different monomers. Conditions: reaction temperature = 42 °C; [monomer]0/[solvent] = 2/1 (v/v)
Entry |
[M]0/[CTA]0/[I]0 |
kappp (h−1) |
Time (h) |
Conv. (%) |
Mthn × 10−3 |
MSECn × 10−3 |
Đ |
Reaction temperature: 60 °C. [Monomer]0/[solvent] = 1/1. |
1a |
[MA]0/[DDMAT]0/[AIBN]0 = 222/1/0.5 |
1.328 |
2 |
93 |
21.1 |
24.5 |
1.06 |
2a |
[S]0/[DDMAT]0/[AIBN]0 = 222/1/0.5 |
0.035 |
49 |
84 |
17.2 |
16.0 |
1.08 |
3 |
[VAc]0/[CMPCD]0/[Trig.]0 = 100/1/0.5 |
— |
2.3 |
99 |
8.7 |
9.0 |
1.18 |
4b |
[VC]0/[CMPCD]0/[Trig.]0 = 250/1/0.2 |
0.185 |
24 |
70 |
12.4 |
11.5 |
1.51 |
5b |
[VC]0/[CMPCD]0/[Trig.]0 = 100/1/0.2 |
— |
5 |
59 |
4.5 |
4.2 |
1.54 |
6b |
[VC]0/[CMPCD]0/[Trig.]0 = 1000/1/0.2 |
— |
48 |
34 |
22.7 |
17.7 |
1.72 |
The ability to polymerize S in CPME under identical experimental conditions was then evaluated. The resulting kinetic plot (see Fig. 2 and entry 2 in Table 1) reveals a linear relationship between ln[M]0/[M] values and time, and a nearly perfect match between experimentally determined Mn values and theoretical ones together with Đ values of about 1.1, indicating a perfect control. In this respect, the actual RAFT system seems to approach the ideal living polymerization conditions much better than the previously-reported SARA-ATRP counterpart.44,46,47 Although low Đ values were also obtained by SARA-ATRP, a loss of chain-end functionality at high monomer conversions was observed, leading to a deviation of MSECn from the theoretical ones,44 with an increase in Đ. This is not observed here, thus indicating that side reactions leading to a loss of functionality were minimized. S polymerizations (in bulk or in solution) usually require high temperatures (typically in the range of 70 to 110 °C) to reach reasonably high conversions and to overcome vitrification and potential catalyst solubility issues.44 With CPME and using RAFT, it is possible to reach high monomer conversions (>95%) at 60 °C. This feature is particularly relevant because high temperatures (especially above 60 °C) can induce S autopolymerization reactions (by thermal self-initiation) on the long term and result in a loss of molecular weight control (which also contributes for MSECn deviation from theoretical values).48 RAFT polymerization of S usually takes place in a DMF solution or in bulk.44 Our results demonstrate that the use of CPME is an excellent green alternative, allowing a rate of polymerization similar to that of systems in DMF and slightly better than bulk polymerizations.49,50 Therefore, CPME can replace DMF for the synthesis of well-defined PS.
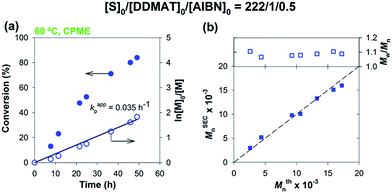 |
| Fig. 2 RAFT polymerization of S in CPME at 60 °C mediated by DDMAT using AIBN as conventional initiator. (a) Conversion vs. time and ln[M]0/[M] vs. time. (b) MSECn and Mw/Mn vs. Mthn. Reaction conditions: [S]0/[DDMAT]0/[AIBN]0 = 222/1/0.5; [S]0/[CPME] = 2/1 (v/v). | |
To demonstrate the versatility of this approach, VC polymerization in CPME was then investigated at a temperature of 42 °C (based on a previous publication from our research group).15 CMPCD was used as CTA and Trigonox was selected as a conventional initiator. Conversely to S, the plot of ln([M]0/[M]) vs. time for VC (Fig. 3) appears to have two distinct linear zones. However, this behavior has already been reported in other RAFT polymerizations of VC,15 and can be attributed to a difference in the rates of initiation by Trigonox-generated radicals, and reinitiation by the radical leaving groups ˙CH2CN formed during the pre-equilibrium reaction of the RAFT mechanism. The apparent polymerization rate constants in CPME are slightly higher than those calculated in the previous work using THF as a solvent.15 Higher conversions were obtained at the end of the reaction, while the level of control over the molecular weight appears to remain the same (with 1.8 > Đ > 1.5 and a good agreement between MSECn and Mthn).
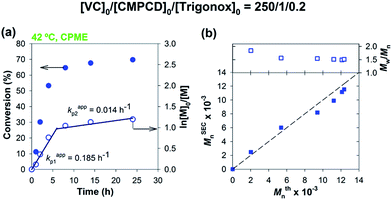 |
| Fig. 3 RAFT polymerization of VC in CPME at 42 °C mediated by CMPCD using Trigonox as conventional initiator. (a) Conversion and ln[M]0/[M] vs. time. (b) MSECn and Mw/Mn vs. Mthn. Reaction conditions: [VC]0/[CMPCD]0/[Trigonox]0 = 250/1/0.2; [VC]0/[CPME] = 1/1 (v/v). | |
The effect of the targeted number-average degree of polymerization (DPn) on the reaction kinetics and the molecular weight control was then studied. Three different DPn values were targeted: 100, 250 and 1000. As expected, the results featured in Table 1 (entries 4–6) show that the higher the targeted DPn, the slower the reaction, as fewer radicals are present in the mixture at a given time. It is remarkable to note that for all the DPn studied, better matches between the MSECn and Mthn values were obtained than those obtained in THF15 despite similar Đ values.
In addition to MA, S and VC, VAc was also tested using the CMPCD/Trigonox RAFT system (see Table 1, entry 3). An almost complete VAc conversion was achieved in less than 3 h, and the low Đ value obtained by SEC analysis confirmed the growth of well-defined PVAc chains.
In conclusion, the results presented in Table 1 confirm the robustness and the versatility of the RAFT polymerization in solution using CPME as solvent. The system allows the synthesis of different polymers in a wide range of molecular weights, while exhibiting a very high level of control that is better or at least similar to other reported solution polymerization systems.10,12,15
Structural analysis of the RAFT-derived polymers
The structure of CTA-terminated PVC chains was determined by 1H-NMR spectroscopy and MALDI-TOF-MS analysis. Both 1H-NMR spectrum (ESI, Fig. S1†) and MALDI-TOF-MS spectrum (ESI, Fig. S2†) confirmed the well-defined structure predicted for the PVC chains.15
In addition, the structure of the RAFT-derived PVAc was also studied by 1H-NMR (Fig. 4). The signals of the PVAc main chain, d and e (at 1.9–2.7 ppm and 4.2–4.8 ppm, respectively) were identified.51 Furthermore, the characteristic signals of CMPCD protons (a, b and c), corresponding to those found previously for PVC (ESI, Fig. S1†) are also present in this spectrum, thereby confirming the presence of chain-end functionalities (from the RAFT agent) in the PVAc chains. This conclusion is consistent with the very good control over the molecular weight that has been observed (Table 1, entry 3).
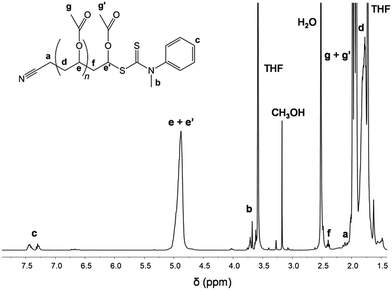 |
| Fig. 4 The 1H NMR spectrum in THF-d8 of PVAc-CTA (MSECn = 9000; Đ = 1.18) obtained in Table 1, entry 3. | |
Evaluation of the RAFT-derived polymer livingness
The living nature of the polymers was confirmed by carrying out successful chain extension experiments using PVC (Table 1, entry 5) and PVAc (Table 1, entry 3) as macro-CTA (Fig. 5 and 6). As shown in Fig. 5, a complete shift of the SEC trace during the “one-pot” chain extension experiment was achieved. Molecular weight of the starting PVC-CTA (convVC = 59%, Mthn = 4500, MSECn = 4200, Đ = 1.54) has shifted toward higher molecular weight (convVC = 42%, Mthn = 23
800, MSECn = 17
300, Đ = 1.53). Also, a PVAc-b-PVC diblock copolymer (convVC = 51%, Mthn = 41
100, MSECn = 30
200, Đ = 1.59) was synthesized from a PVAc-CTA (convVAc = 99%, Mthn = 8700, MSECn = 9000, Đ = 1.18) was synthesized (Fig. 6). The structure of this block copolymer was confirmed by 1H NMR (ESI, Fig. S3†).
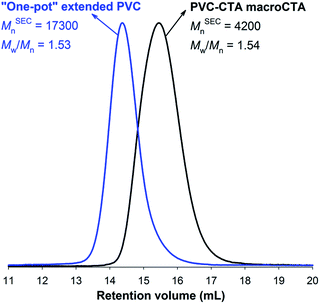 |
| Fig. 5 SEC traces of the PVC-CTA (convVC = 59%, Mthn = 4500, MSECn = 4200, Đ = 1.54) macro-CTA (right curve), and the “one-pot” extended PVC (convVC = 42%, Mthn = 23 800, MSECn = 17 300, Đ = 1.53) (left curve). | |
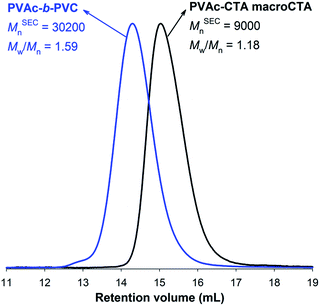 |
| Fig. 6 SEC traces of the PVAc-CTA (convVAc = 99%, Mthn = 8700, MSECn = 9000, Đ = 1.18) macro-CTA (right curve), and the PVAc-b-PVC (convVC = 51%, Mthn = 41 100, MSECn = 30 200, Đ = 1.59) block copolymer (left curve). | |
NMP in CPME
For the NMP of S and VC, the BlocBuilder alkoxyamine, based on the nitroxide SG1, was selected. It is one of the most potent alkoxyamines developed so far and its use has conducted to significant advances in the control of bulk/solution and emulsion polymerizations, and the preparation of complex and functionalized polymer architectures.2
The NMP of S using this alkoxyamine is usually performed in bulk at high temperatures; between 90 and 120 °C,2,52–54 to enable reasonable polymerization rates. However, this range of temperatures is not fully compatible with the use of CPME (boiling point is 106 °C), unless a high pressure glassware is employed. In this context, a preliminary experiment of S polymerization was carried out at 60 °C. However, as expected, the polymerization was extremely slow as no polymer was formed even after 94 h. The temperature was then increased to 80 °C but the reaction proceeded rather slowly (Fig. 7). Nevertheless, all the expected features of a RDRP system (e.g., first-order kinetic with respect to monomer conversion, linear increase of Mn with monomer conversion, good match between MSECn and Mthn values and low Đ values decreasing with monomer conversion) were obtained with a level of control comparable to that reported for NMP of S using BlocBuilder at higher temperatures.53–55 The use of a temperature lower than those reported in the literature may also contribute to a good control over the polymerization due to a lower rate of S autopolymerization, similarly to what was earlier discussed with RAFT. Therefore, despite temperature limitations resulting in slow reactions, the very good control achieved over the polymerization of S validates the use of CPME as a solvent in the field of NMP.
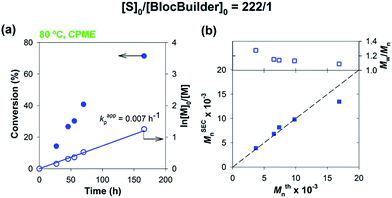 |
| Fig. 7 NMP of S in CPME at 80 °C initiated by SG1-based BlocBuilder alkoxyamine. (a) Conversion and ln[M]0/[M] vs. time. (b) MSECn and Mw/Mn vs. Mthn. Reaction conditions: [S]0/[BlocBuilder]0 = 222/1; [S]0/[CPME] = 2/1 (v/v). | |
The NMP of VC initiated by the BlocBuilder alkoxyamine in DCM or DMSO was recently proposed, and the results pointed out that a temperature of 42 °C provided the best compromise between a descent polymerization rate and a good control.40 Based on this work, the NMP of VC was investigated with CPME as the solvent. The kinetic data show a first-order kinetic (Fig. 8a) and a good agreement between MSECn and Mthn values (Fig. 8b). The Đ follow those obtained in DCM under identical experimental conditions, approaching 1.5 at the end of the reaction,40 which suggests a similar level of control. However, the rate of polymerization in CPME (kappp = 0.042) was ∼20% higher than that reported using DCM (kappp = 0.036),40 which stresses another advantage of replacing DCM and DMSO by CPME.
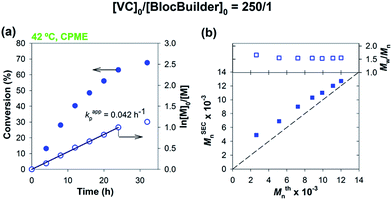 |
| Fig. 8 NMP of VC in CPME at 42 °C initiated by SG1-based BlocBuilder alkoxyamine. (a) Conversion and ln[M]0/[M] vs. time. (b) MSECn and Mw/Mn vs. Mthn. Reaction conditions: [VC]0/[BlocBuilder]0 = 250/1; [VC]0/[CPME] = 1/1 (v/v). | |
The influence of the monomer concentration in the reaction medium was then investigated for VC, by adjusting the monomer/solvent ratio and stopping the reaction after 24 h (Table 2, entries 1–3). It appears that a decrease in the [VC]0/[CPME] ratio from 1/1 (entry 1) to 1/2 (entry 3) has a deleterious effect on the final monomer conversion, which is caused by a change of the reaction medium (polarity and/or viscosity) upon dilution, as previously observed for different NMP systems.16,56 Conversely, when the [VC]0/[CPME] ratio increases from 1/1 (entry 1) to 2/1 (entry 2), dispersity increased slightly (1.54 vs. 1.69), similarly to solution NMP of oligo(ethylene glycol) methyl ether methacrylate (OEGMA) in ethanol.56 With VC, the same observations were made in DCM system.40
Table 2 NMP of VC initiated by the BlocBuilder alkoxyamine at 42 °C, using CPME as a solvent under different experimental conditions
Entry |
[VC]0/[BlocBuilder]0 |
[VC]0/[CPME] (v/v) |
Time (h) |
Conv. (%) |
Mthn × 10−3 |
MSECn × 10−3 |
Đ |
1 |
250/1 |
1/1 |
24 |
63.1 |
11.1 |
12.0 |
1.54 |
2 |
250/1 |
2/1 |
24 |
58.1 |
10.3 |
11.1 |
1.69 |
3 |
250/1 |
1/2 |
24 |
42.3 |
7.8 |
8.5 |
1.59 |
4 |
100/1 |
1/1 |
10 |
52.2 |
3.8 |
4.3 |
1.55 |
5 |
1000/1 |
1/1 |
48 |
54.0 |
36.3 |
32.3 |
1.53 |
Finally the NMP system proposed here was further tested for different target DPns. Once again DPn values of 100 and 1000 were tested and compared with a DPn of 250 (Table 2, entries 1, 4 and 5). The results not only confirm the reduction of polymerization rate as DPn increases but also the excellent control all across the DPn range tested.
Structural analysis of the NMP-derived polymers
The evidences of the well-defined structure of the PVC were obtained by 1H NMR spectroscopy (ESI, Fig. S4†). The spectrum shows the expected peaks and in particular those from the starting alkoxyamine, thus proving the chain-end functionalization, in agreement with those reported in the literature for NMP-derived PVC prepared in other organic solvents.40
The end-group fidelity of the synthesized PVC was probed by performing 31P NMR spectroscopy, which is a convenient and pretty accurate method for determination of the living chain fraction (LF) by quantifying the presence of the phosphorus-containing SG1 nitroxide end-group using diethyl phosphite (DEP) as an internal reference.57,58 The PVC-SG1 spectrum reported in Fig. 9 gave a LF of ∼87%, which is similar to LF values reported for the NMP of styrenics and acrylates. This clearly demonstrates the living nature of the PVC obtained in CPME. It also enables the design of block copolymers by NMP containing PVC segments by chain extensions.
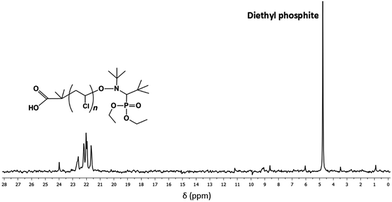 |
| Fig. 9 31P NMR spectra in d8-THF of the purified PVC (MSECn = 4300; Đ = 1.55) obtained in Table 2, entry 4. | |
Evaluation of the NMP-derived polymer livingness
The living character of the PVC-SG1 obtained by NMP was confirmed by a successful “one-pot” chain extension experiment of VC from the SG1-terminated PVC (Table 2, entry 4) in CPME at 42 °C. The SEC traces presented in Fig. 10 showed the complete shift of the low molecular weight SG1-terminated PVC macroinitiator (convVC = 52%, Mthn = 3800, MSECn = 4300, Đ = 1.55) towards a higher molecular weight polymer (convVC = 47%, Mthn = 29
800, MSECn = 23
600, Đ = 1.61), thus assessing the formation of a PVC-b-PVC diblock copolymer.
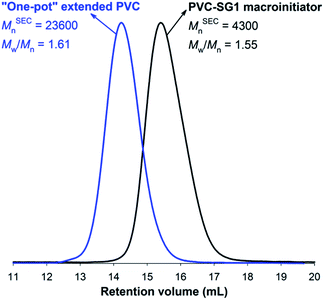 |
| Fig. 10 SEC chromatograms of the PVC-SG1 macroinitiator (convVC = 52.2%, Mthn = 3800, MSECn = 4300, Đ = 1.55) (black line) and the PVC-b-PVC diblock copolymer (convVC = 47.2%, Mthn = 29 800, MSECn = 23 600, Đ = 1.61) (blue line) after “one-pot” chain extension in CPME. | |
Conclusions
In conclusion this work reports the use of CPME for the NMP and RAFT polymerizations of VC and S as alternative to THF, DCM, DMSO and DMF. This eco-friendly solvent allowed also the RAFT polymerization of VAc and MA. The structures of the obtained polymers were confirmed by 1H-NMR, 31P-NMR, MALDI-TOF-MS and the living character was confirmed by successful chain extension experiments. The results proved that CPME is a suitable “green” solvent to be employed in two of the most popular RDRP methods.
Acknowledgements
Carlos M. R. Abreu acknowledges FTC-MCTES for his PhD scholarship (SFRH/BD/88528/2012). The 1H NMR and 31P NMR data were obtained at the Nuclear Magnetic Resonance Laboratory of the Coimbra Chemistry Centre (http://www.nmrccc.uc.pt), University of Coimbra, supported in part by Grant REEQ/481/QUI/2006 from FCT, POCI-2010, and FEDER, Portugal. The MALDI-TOF-MS data were obtained by Manuel Marcos Garcia and Paula Alvarez Chaver at Unidad de Espectrometria de Masas do Servicio de Determinación Estructural, Proteómica y Genómica, Centro de Apoyo Cientifíco y Tecnologico a la Investigación (CACTI, University of Vigo, Spain).
Notes and references
- K. Matyjaszewski and N. V. Tsarevsky, J. Am. Chem. Soc., 2014, 136, 6513–6533 CrossRef CAS.
- J. Nicolas, Y. Guillaneuf, C. Lefay, D. Bertin, D. Gigmes and B. Charleux, Prog. Polym. Sci., 2013, 38, 63–235 CrossRef CAS.
- G. Moad, E. Rizzardo and S. H. Thang, Aust. J. Chem., 2012, 65, 985–1076 CrossRef CAS.
- G. Moad, E. Rizzardo and S. H. Thang, Aust. J. Chem., 2009, 62, 1402–1472 CrossRef CAS.
- K. Matyjaszewski and J. H. Xia, Chem. Rev., 2001, 101, 2921–2990 CrossRef CAS.
- M. Kamigaito, T. Ando and M. Sawamoto, Chem. Rev., 2001, 101, 3689–3745 CrossRef CAS.
- J.-S. Wang and K. Matyjaszewski, J. Am. Chem. Soc., 1995, 117, 5614–5615 CrossRef CAS.
- M. K. Georges, R. P. N. Veregin, P. M. Kazmaier and G. K. Hamer, Macromolecules, 1993, 26, 2987–2988 CrossRef CAS.
- S. Perrier and P. Takolpuckdee, J. Polym. Sci., Part A: Polym. Chem., 2005, 43, 5347–5393 CrossRef CAS.
- G. Moad, E. Rizzardo and S. H. Thang, Aust. J. Chem., 2005, 58, 379–410 CrossRef CAS.
- J. Chiefari, Y. K. Chong, F. Ercole, J. Krstina, J. Jeffery, T. P. T. Le, R. T. A. Mayadunne, G. F. Meijs, C. L. Moad, G. Moad, E. Rizzardo and S. H. Thang, Macromolecules, 1998, 31, 5559–5562 CrossRef CAS.
- G. Moad, E. Rizzardo and S. H. Thang, Acc. Chem. Res., 2008, 41, 1133–1142 CrossRef CAS.
- D. Konkolewicz, Y. Wang, P. Krys, M. Zhong, A. A. Isse, A. Gennaro and K. Matyjaszewski, Polym. Chem., 2014, 5, 4396–4417 RSC.
- T. Guliashvili, P. V. Mendonca, A. C. Serra, A. V. Popov and J. F. J. Coelho, Chem.–Eur. J., 2012, 18, 4607–4612 CrossRef CAS.
- C. M. R. Abreu, P. V. Mendonca, A. C. Serra, J. F. J. Coelho, A. V. Popov, G. Gryn'ova, M. L. Coote and T. Guliashvili, Macromolecules, 2012, 45, 2200–2208 CrossRef CAS.
- M. Chenal, S. Mura, C. Marchal, D. Gigmes, B. Charleux, E. Fattal, P. Couvreur and J. Nicolas, Macromolecules, 2010, 43, 9291–9303 CrossRef CAS.
- K.-H. Kuo, W.-Y. Chiu and K.-C. Cheng, Polym. Int., 2008, 57, 730–737 CrossRef CAS.
- P. V. Mendonça, S. E. Averick, D. Konkolewicz, A. C. Serra, A. V. Popov, T. Guliashvili, K. Matyjaszewski and J. F. J. Coelho, Macromolecules, 2014, 47, 4615–4621 CrossRef.
- D. Konkolewicz, P. Krys, J. R. Góis, P. V. Mendonça, M. Zhong, Y. Wang, A. Gennaro, A. A. Isse, M. Fantin and K. Matyjaszewski, Macromolecules, 2014, 47, 560–570 CrossRef CAS.
- A. Simakova, S. E. Averick, D. Konkolewicz and K. Matyjaszewski, Macromolecules, 2012, 45, 6371–6379 CrossRef CAS.
- D. Konkolewicz, A. J. D. Magenau, S. E. Averick, A. Simakova, H. He and K. Matyjaszewski, Macromolecules, 2012, 45, 4461–4468 CrossRef CAS.
- P. V. Mendonca, D. Konkolewicz, S. E. Averick, A. C. Serra, A. V. Popov, T. Guliashvili, K. Matyjaszewski and J. F. J. Coelho, Polym. Chem., 2014, 5, 5829–5836 RSC.
- J. R. Gois, N. Rocha, A. V. Popov, T. Guliashvili, K. Matyjaszewski, A. C. Serra and J. F. J. Coelho, Polym. Chem., 2014, 5, 3919–3928 RSC.
- J. R. Gois, D. Konkolewic, A. V. Popov, T. Guliashvili, K. Matyjaszewski, A. C. Serra and J. F. J. Coelho, Polym. Chem., 2014, 5, 4617–4626 RSC.
- F. Huo, X. Wang, Y. Zhang, X. Zhang, J. Xu and W. Zhang, Macromol. Chem. Phys., 2013, 214, 902–911 CrossRef CAS.
- R. A. Cordeiro, N. Rocha, J. P. Mendes, K. Matyjaszewski, T. Guliashvili, A. C. Serra and J. F. J. Coelho, Polym. Chem., 2013, 4, 3088–3097 RSC.
- C. M. R. Abreu, A. C. Serra, A. V. Popov, K. Matyjaszewski, T. Guliashvili and J. F. J. Coelho, Polym. Chem., 2013, 4, 5629–5636 RSC.
- C. M. R. Abreu, P. V. Mendonça, A. C. Serra, J. F. J. Coelho, A. V. Popov and T. Guliashvili, Macromol. Chem. Phys., 2012, 213, 1677–1687 CrossRef CAS.
- J. R. C. Costa, P. V. Mendonça, P. Maximiano, A. C. Serra, T. Guliashvili and J. F. J. Coelho, Macromolecules, 2015, 48, 6810–6815 CrossRef CAS.
- A. Anastasaki, V. Nikolaou, G. Nurumbetov, N. P. Truong, G. S. Pappas, N. G. Engelis, J. F. Quinn, M. R. Whittaker, T. P. Davis and D. M. Haddleton, Macromolecules, 2015, 48, 5140–5147 CrossRef CAS.
- J. P. Mendes, F. Branco, C. M. R. Abreu, P. V. Mendonca, A. V. Popov, T. Guliashvili, A. C. Serra and J. F. J. Coelho, ACS Macro Lett., 2014, 3, 544–547 CrossRef CAS.
- T. Erdmenger, C. Guerrero-Sanchez, J. Vitz, R. Hoogenboom and U. S. Schubert, Chem. Soc. Rev., 2010, 39, 3317–3333 RSC.
- T. Biedroń and P. Kubisa, J. Polym. Sci., Part A: Polym. Chem., 2002, 40, 2799–2809 CrossRef.
- A. J. Carmichael, D. M. Haddleton, S. A. F. Bon and K. R. Seddon, Chem. Commun., 2000, 1237–1238 RSC.
- C. Magee, A. Earla, J. Petraitis, C. Higa, R. Braslau, P. B. Zetterlund and F. Aldabbagh, Polym. Chem., 2014, 5, 5725–5733 RSC.
- K. Watanabe, N. Yamagiwa and Y. Torisawa, Org. Process Res. Dev., 2007, 11, 251–258 CrossRef CAS.
- K. Watanabe, Molecules, 2013, 18, 3183 CrossRef CAS.
- V. Antonucci, J. Coleman, J. B. Ferry, N. Johnson, M. Mathe, J. P. Scott and J. Xu, Org. Process Res. Dev., 2011, 15, 939–941 CrossRef CAS.
- P. Maximiano, J. P. Mendes, P. V. Mendonça, C. M. R. Abreu, T. Guliashvili, A. C. Serra and J. F. J. Coelho, J. Polym. Sci., Part A: Polym. Chem., 2015, 53, 2722–2729 CrossRef CAS.
- C. M. R. Abreu, P. V. Mendonca, A. C. Serra, B. B. Noble, T. Guliashvili, J. Nicolas, M. L. Coote and J. F. J. Coelho, 2015, submitted to Macromolecules, DOI:10.1021/acs.macromol.5b02017.
- T. Hatano, B. M. Rosen and V. Percec, J. Polym. Sci., Part A: Polym. Chem., 2010, 48, 164–172 CrossRef CAS.
- M. J. Sienkowska, B. M. Rosen and V. Percec, J. Polym. Sci., Part A: Polym. Chem., 2009, 47, 4130–4140 CrossRef CAS.
- V. Percec, T. Guliashvili, J. S. Ladislaw, A. Wistrand, A. Stjerndahl, M. J. Sienkowska, M. J. Monteiro and S. Sahoo, J. Am. Chem. Soc., 2006, 128, 14156–14165 CrossRef CAS.
- N. Rocha, P. V. Mendonça, J. P. Mendes, P. N. Simões, A. V. Popov, T. Guliashvili, A. C. Serra and J. F. J. Coelho, Macromol. Chem. Phys., 2013, 214, 76–84 CrossRef CAS.
- C. M. R. Abreu, P. V. Mendonca, A. C. Serra, A. V. Popov, K. Matyjaszewski, T. Guliashvili and J. F. J. Coelho, ACS Macro Lett., 2012, 1, 1308–1311 CrossRef CAS.
- P. V. Mendonca, A. C. Serra, J. F. J. Coelho, A. V. Popov and T. Guliashvili, Eur. Polym. J., 2011, 47, 1460–1466 CrossRef CAS.
- J. P. Mendes, F. Branco, C. M. R. Abreu, P. V. Mendonca, A. C. Serra, A. V. Popov, T. Guliashvili and J. F. J. Coelho, ACS Macro Lett., 2014, 3, 858–861 CrossRef CAS.
- F. R. Mayo, J. Am. Chem. Soc., 1968, 90, 1289–1295 CrossRef CAS.
- Y. Wu, W. Zhang, Z. Zhang, X. Pan, Z. Cheng, J. Zhu and X. Zhu, Chem. Commun., 2014, 50, 9722–9724 RSC.
- A. Goto, K. Sato, Y. Tsujii, T. Fukuda, G. Moad, E. Rizzardo and S. H. Thang, Macromolecules, 2001, 34, 402–408 CrossRef CAS.
- Y. Piette, A. Debuigne, V. Bodart, N. Willet, A.-S. Duwez, C. Jerome and C. Detrembleur, Polym. Chem., 2013, 4, 1685–1693 RSC.
- B. Charleux and J. Nicolas, Polym. J., 2007, 48, 5813–5833 CAS.
- S. Grimaldi, J.-P. Finet, F. le Moigne, A. Zeghdaoui, P. Tordo, D. Benoit, M. Fontanille and Y. Gnanou, Macromolecules, 2000, 33, 1141–1147 CrossRef CAS.
- D. Benoit, S. Grimaldi, S. Robin, J.-P. Finet, P. Tordo and Y. Gnanou, J. Am. Chem. Soc., 2000, 122, 5929–5939 CrossRef CAS.
- J. Nicolas, B. Charleux, O. Guerret and S. Magnet, Macromolecules, 2004, 37, 4453–4463 CrossRef CAS.
- J. Nicolas, P. Couvreur and B. Charleux, Macromolecules, 2008, 41, 3758–3761 CrossRef CAS.
- V. Delplace, S. Harrisson, H. T. Ho, A. Tardy, Y. Guillaneuf, S. Pascual, L. Fontaine and J. Nicolas, Macromolecules, 2015, 48, 2087–2097 CrossRef CAS.
- J. Nicolas, C. Dire, L. Mueller, J. Belleney, B. Charleux, S. R. A. Marque, D. Bertin, S. Magnet and L. Couvreur, Macromolecules, 2006, 39, 8274–8282 CrossRef CAS.
Footnote |
† Electronic supplementary information (ESI) available: Experimental and characterization details. See DOI: 10.1039/c5ra21975b |
|
This journal is © The Royal Society of Chemistry 2016 |