DOI:
10.1039/C5RA17187C
(Paper)
RSC Adv., 2016,
6, 851-858
Excess-electron-induced C–C bond formation in transformation of carbon dioxide
Received
26th August 2015
, Accepted 8th December 2015
First published on 9th December 2015
Abstract
This study presents a new fixation method of CO2 through excess-electron-induced C–C bond formation using quantum chemical method. Because of active CO2−˙ with a distinct radical character at the carbon center, two divalent anion complexes [O2C–C6H6–CO2]2− (cis-II and trans-II) are obtained via C–C bond formation between the carbon atom of C6H6 and the carbon atom of CO2 in the process of CO2−˙ radical attacking on benzene molecule. Furthermore, the transformations of cis-II and trans-II are predicted. We found that the more favorable transformation is for cis-II. It can produce terephthalic and one H2 molecule via two H-atom elimination with the energy barrier of 35.70 kcal mol−1. Furthermore, we found that the formed hydrogen bond complex CO2–HCN did not reduce the energy barrier; however, it could reduce the energy of the transition state with respect to that of the reactant, due to it dispersing the charge of benzene ring.
1. Introduction
The study of the transformation of carbon dioxide is currently one of the most active research areas in the scientific community,1–5 because the potentially devastating effects of steadily increasing concentrations of CO2 are threatening the sustainable development of our society. Recently, a number of studies have shown that the use of CO2 can not only generate useful organic compounds from a nontoxic, abundant, and economical carbon resource,1–11 but also to a certain extent, reduce its atmospheric concentration. However, only a few reaction processes utilize CO2 as a raw material because CO2 is the most oxidized state of carbon and highly stable. Therefore, much effort needs to be made regarding the transformation of CO2.7,10 In recent years, various strategies of promoting the reactivity of organic materials toward CO2 have been proposed, especially with regard to chemical transformation. The first proposal concentrates on coordinating CO2 to transition-metal complexes10–14 wherein cleavage of the transformed CO2 moiety from the metal center hinders catalytic application. Second, CO2 reacts with unsaturated hydrocarbons, for example, the oxidative coupling of CO2 with olefins and alkynes.15–29 The third one concerns insertion of CO2 into M–X bonds (M = metal, X = C, O and H).30–41 When a CO2 molecule inserts into the M–C bond of a compound, a carboxylate species would be formed.30–35 By inserting into the M–H bond with suitable catalysts, the synthesis of formic acid from the less toxic and more abundant CO2 is feasible, and the insertion of CO2 into M–O bonds results in the formation of carbonate species.36–41 Another charming reaction strategy is the photocatalytic and electrochemical reduction of CO2.42,43 Despite many studies reporting the transformation of CO2 by various physical and chemical means, it must be noted that most of reports still go back to the initial concepts of transition metal catalysis proposed by the research groups of Inoue, Musco, Hoberg, Walther and Behr et al. For example, about three decades ago, Hoberg and Walther started examining the oxidative coupling of carbon dioxide (1 bar) and olefins catalyzed by nickel(0) complexes or iron(0) complexes.44,45 The research groups of Inoue and Musco were the first to report the reaction between carbon dioxide and dienes catalyzed by palladium(0) complexes.46,47 Behr and co-workers improved the original synthetic procedures of Inoue and Musco using palladium(II) acetate or acetylacetonate complexes and phosphines in acetonitrile as the solvent, which led to a significant increase in the yield of the lactones.48 However, no new breakthrough in the transformation of CO2 has been developed. Therefore, it is significant that new transformation methods for using CO2 as a starting material emerge in the near future.
The most prevalent obstacle of CO2 usage is that it is a weak electrophile. It is difficult to achieve C–C bond formation when reacting with organic molecules. Luckily, the assistance from transition metals may be a good approach to address this problem.10–43 Herein, we present idea that free electron assisting provides an alternative approach to achieve the transformation reactions (see Scheme 1). As shown in Scheme 1, first, CO2 captures a free electron to form active CO2−˙ with a distinct radical character at the carbon center. Then, the active CO2−˙ reacts with an organic molecule H-CR to form intermediate electron–molecule compounds via C–C bond formation. Finally, one product would be obtained through dissociating an electron. In recent years, chemical reactions via free electron assisting have been widely investigated. For example, upon electron attachment, the van der Waals complex of azabenzene and CO2 (ref. 49 and 50) would generate a covalent bond between the nitrogen atom and the carbon atom of CO2 through an extended π-orbital conjugation over all the moieties of the complex. Swiderek et al. have demonstrated a new strategy to synthesize C2H5NH2 from C2H4 and NH3 by low-energy free electrons induction,51 which relied on the electrostatic attraction caused by the soft ionization of one of the reaction partners. Another important instance was that an excess electron induced the hydrogen-bonded complex, NH3⋯HCl, to form the ionic salt,52 first forming a dipole-bound anion of NH4Cl, and then a deformed Rydberg radical NH4, polarized by a chloride anion, Cl−. For electron impact catalytic reaction, the Sajeev group reported a new elementary reaction mechanism of an electron and a molecule in a metastable compound,53 which strongly relates to bond breaking and bond formation.
 |
| Scheme 1 One potential reaction route under an excess electron action. | |
Schröder et al. stated that the geometrically bent radical anion of CO2 constitutes a new type of highly reactive ion, and therefore it can be regarded as an activated CO2 unit.54 Although in a real gas phase experiment it is difficult to use CO2−˙ as a reactant, due to its short lifetime. Sajeev and Davis proposed a good strategy wherein the formation of the weak molecular complex [CO2·HCN]−˙ helps the CO2−˙ moiety have longer lifetime and permanent reactivity.55 Beyer's group presented CO2−˙ species that are concurrently solvated and stabilized by water ligands forming CO2−˙(H2O)n56–59 and performed their reactions with CH3SSCH3 to achieve cleavage of the disulfide bond in the gas phase by Fourier transform ion cyclotron resonance mass spectrometry.
It is useful to understand the reaction of active CO2−˙ and the benzene molecule to investigate other reactions of long-lived CO2−˙ species in weak molecular complexes. CO2−˙ attacks C6H6, which leads to the formation of a new C–C chemical bond. This is the first step to fix and transform CO2 by electron impact. Furthermore, because of the attack from CO2−˙, the aromaticity of C6H6 ring is broken in the electron–molecule compound [C6H6–CO2]−˙. Then, a second CO2−˙ would further attach to the electron–molecule compound [C6H6–CO2]−˙ to form the divalent anion complexes cis-II, trans-II and the hydrogen bond complex I (see Scheme 2).
 |
| Scheme 2 Possible anion complexes formed by CO2−˙ attaching to C6H6. | |
In this study, we investigated the reaction between CO2−˙ and C6H6 to form the divalent anion complexes, cis-II, trans-II and hydrogen bond complex, I, via C–C bond formation (see Scheme 2). Furthermore, transformations of cis-II and trans-II are predicted; we found that the most likely transformation was that cis-II transforms to terephthalic and one H2 molecule via H elimination with an energy barrier of 35.70 kcal mol−1. Subsequently, investigations showed that the long-lived CO2−˙ moiety in the weak molecular complex of [CO2·HCN]−˙ also had permanent reactivity to induce C–C bond formation with the energy barrier of 36.35 kcal mol−1. However, we found that it could reduce the energy of the transition state with respect to that of the reactant, due to it dispersing the charge on benzene ring. Therefore, we hope that this study is useful for further theoretical and experimental studies regarding the transformation of CO2.
2. Computational details
All the calculations were performed with the GAUSSIAN 09 programs.60 The geometrical optimizations of all the intermediates and transition states were performed at the second-order Møller-Plesset perturbation theory (MP2) level with the augmented correlation consistent basis set aug-cc-PVDZ. All studied structures are checked to be closed-shell structures, except for [C6H6–CO2]−˙ and CO2−˙. Frequency calculations at the same level were performed to confirm each stationary point to be either a minimum or a transition structure (TS). Energy-minimum geometries had only real frequencies, whereas TS geometries had an imaginary frequency corresponding to the relevant reaction coordinate. In several cases wherein TS are not easily confirmed by animation of their vibrations, intrinsic reaction coordinate (IRC)61,62 calculations were performed to confirm the connection of each TS to its corresponding reactant and product. Moreover, zero-point energy (ZPE) is also evaluated with a scale factor of 1. Furthermore, high-level (CCSD(t)/aug-cc-PVDZ) energy calculation on the optimized structures is carried out to obtain the single point energy. The charge distributions of complexes were obtained by natural bond orbital (NBO)63–65 analyses at the MP2/aug-cc-PVDZ level.
3. Results and discussion
3.1 Formation of electron–molecule complex [C6H6–CO2]−˙
A metastable electron–molecule compound [C6H6–CO2]−˙ is obtained in the process of CO2−˙ attacking the benzene molecule. The barrier of C–C bond formation is only 7.22 kcal mol−1, due to CO2−˙ exhibiting radical character. Fig. 1 shows the optimized reactant, TS and product structures. Fig. 2 lists HOMOs of [C6H6–CO2]−˙ and TS[C6H6–CO2]−˙ to roughly illustrate the formation of [C6H6–CO2]−˙. From Fig. 2, the electron density transfers from the rich C6H6 ring to the C atom with the positive charge in CO2−˙; moreover, the electron density of O atoms in CO2−˙ gives a feedback on to the C6H6 ring (see Fig. 2a). The ensuing charge transfer therefore enhances the interaction between C6H6 and CO2−˙. In the electron–molecule [C6H6–CO2]−˙, the bond length of C1–C2 is 1.618 Å, which is longer than the usual C–C bond (∼1.55 Å). The formation of the new C1–C2 bond is a key step to fix and transform CO2. Because the conjugation of the C6H6 ring is destroyed as a result of the CO2−˙, the C6H6 ring becomes reactive. The second CO2−˙ could be further attached to electron–molecule compound [C6H6–CO2]−˙ to form the anion complexes cis-II and trans-II with no energy barriers (this issue will be discussed in the next section).
 |
| Fig. 1 PES profile for the process of CO2−˙ attaching to C6H6. | |
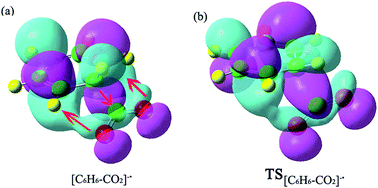 |
| Fig. 2 HOMOs of [C6H6–CO2]−˙ and TS[C6H6–CO2]−˙ showing charge transfers to and fro from the new C–C bond. | |
The geometrical parameters of the optimized reactant, TS and product structures are listed in Fig. 1. In the reactant CO2−˙, the bend angle of O–C–O is 136° and the bond lengths of C–O are 1.251 Å, whereas in the product structure, [C6H6–CO2]−˙, the bend angle of O–C–O shrinks to 131° and the bond lengths of C–O extend to 1.261 Å.49,50 This indicates that the newly formed C1–C2 bond further weakens the C–O bond and activates the C6H6 ring. From Fig. 1, we found that the C1–C2–C3 bond angle of 109.7° in TS[C6H6–CO2]−˙ is much smaller than 135.7° in [C6H6–CO2]−˙, this indicates that the process that produced [C6H6–CO2]−˙ has a tendency towards forming an extended π-conjugated structure.
Fig. 1 shows that the formation of [C6H6–CO2]−˙ may be a reversible reaction. However, the formation of the C1–C2 bond provides an activation of the C6H6 ring, and the second CO2−˙ easily attacks the C6H6 ring to form the divalent anion complexes [O2C–C6H6–CO2]2− (cis-II and trans-II) with no energy barrier. This can be considered as the combination between two radical ions (see Scheme 3 for two possible mechanisms of the formation of the radical ion, [C6H6–CO2]−˙). Thus, for the step of formation of [C6H6–CO2]−˙, the shift of the equilibrium is promoted to the product by depleting the [C6H6–CO2]−˙ (see Scheme 4).
 |
| Scheme 3 The stable radical ions. | |
 |
| Scheme 4 The shift of the equilibrium. | |
3.2 Fixation of the second CO2−˙ to form divalent anion complexes
Due to the activation of C6H6, the second CO2−˙ can easily fix the electron–molecule compound [C6H6–CO2]−˙ to form divalent anion complexes [O2C–C6H6–CO2]2− (see Scheme 2). Three possible anion complexes cis-II, trans-II and hydrogen bond complex I are shown in Fig. 3. In the divalent anions [O2C–C6H6–CO2]2−, the C1–C2 bond is reduced by 0.034–0.047 Å, which indicates that the strength of C1–C2 bonds is enhanced. As shown in Fig. 4, the second CO2−˙ easily attacks the C6H6 ring to form the divalent anion complexes cis-II and trans-II with no energy barrier. However, comparing with the reactants, the energies of cis-II and trans-II only decrease by 1.96 and 2.21 kcal mol−1, respectively. However, the formation of hydrogen bond complex I is very energetically unfavorable; this formation step is a strong endothermic reaction requiring the energy of 54.00 kcal mol−1. Therefore, we concluded that the reaction of the second CO2−˙ attacking C6H6 ring may follow a pathway producing cis-II or trans-II, with the former process being energetically more favorable.
 |
| Fig. 3 Optimized structures and their geometrical parameters. Bond lengths are in Angstroms and angles are in degrees. | |
 |
| Fig. 4 Energy profiles for the CO2 transformation reaction. | |
Predicting feasible transformation routes is important to the study of CO2 transformation. Thus, we calculated the possible transformations of cis-II and trans-II. Fig. 3 depicts the evolution of the structures, including the reactants, TS and product structures. Although the formation of hydrogen bond complex I is unfavorable in energy terms, we still considered it to compare with the transformations of cis-II and trans-II. (1) Supposing that hydrogen bond complex I may transform to OCOH− and ph-COO−. In hydrogen bond complex I, due to the formation of a hydrogen bond, the C2–H1 bond length is lengthened to 1.156 Å, which is longer by 0.042 Å than that in the electron–molecule compound [C6H6–CO2]−˙ (1.114 Å). This indicates that the C2–H1 bond has been activated. In TS-I, the C2–H1 bond length is 1.481 Å and the C2–C1 bond length is shortened to 1.561 Å. Interestingly, the C1–C2–C3 bond angle is approximately thought of as the dihedral between the two planes of C6H6 and CO2−˙. It is found that this is 163.2°, 172.6° and 180° for the reactant, TS and product structures, respectively. This indicates that π66 of C6H6 and π35 of CO2−˙ become more and more coupled to form an extended π-conjugated network from the reactant to product structure. (2) For the transformation of trans-II, the reaction starts with H-transfer from atom C2 to atom O1 to form an intermediate trans-II-a. Then, the second H-transfer process occurs to form the product [HOOC-ph-COOH]2− (terephthalic acid with two negative charges). As shown in Fig. 3, although the second CO2−˙ easily fixes onto the C6H6 ring with no energy barrier, the C2–H1 bond has not been activated, which is different from hydrogen bond complex I. With the evolution of the structures from the reactant to product, the C1–C2 and C3–C4 bond lengths become shorter and shorter. In the product [HOOC-ph-COOH]2−, their bond lengths decrease to 1.400 Å, which would exhibit double bond character. For the change of C1–C2–C3 (C2–C3–C4) bond angle, it is important to understand the formation of the product. It is found that both C1–C2–C3 and C2–C3–C4 bond angles increase with the evolution of the structures, even though C1–C2–C3 (or C2–C3–C4) has a small decrease in the transition state structure TS2-trans-II (or TS1-trans-II). These indicate that an extended π-conjugated network was formed by the coupling of one π66 of C6H6 and two π35 of CO2−˙. (3) As shown in Fig. 3, cis-II can transform to one −OOC-ph-COO− and one H2 molecule, and this plays a significant role in the transformation of CO2, because it generates not only a useful chemical, but also a clean energy source of H2. In the transformation of cis-II, the reactant cis-II with C2v symmetry strengthens the C1–C2 and C3–C4 bond; they are 1.578 Å, which is shorter than that in the electron–molecule compound [C6H6–CO2]−˙ (1.618 Å). As in trans-II, the C2–H1 bond has also not been activated, whereas in TS-cis-II, the C1–C2 and C3–C4 bonds of 1.554 Å slightly decrease by 0.024 Å. It can be noted that the H1–H2 distance is only 1.020 Å, which is much shorter than that in cis-II (3.394 Å). As in trans-II and hydrogen bond complex I, the change in C1–C2–C3 (C2–C3–C4) bond angle indicates that the formation of an extended π-conjugated network promotes the evolution of the structures and stabilizes the product complexes.
The transformation energy profile is presented in Fig. 4. As mentioned above, the formation of hydrogen bond complex I is very energetically unfavorable; therefore, we do not discuss its transformation here. For complex trans-II, in its transformation to the product it undergoes two transition states TS1-trans-II and TS2-trans-II. In TS1-trans-II (TS2-trans-II), the H-transfer process from C2 (C3) to O1 (O2) occurs via a four-membered ring C–H–O–C. However, if the H-transfer process occurs, the energy barriers of TS1-trans-II and TS2-trans-II are high (47.01 and 59.45 kcal mol−1, respectively) and too high to achieve H-transfer. For cis-II, its transformation to the product only undergoes one transition state TS-cis-II (1635i cm−1), which corresponds to the vibration of H elimination with an energy barrier of 35.70 kcal mol−1. Compared to the transformation of trans-II, we found that the process of the formation of one H2 by H elimination is easier than the H-transfer process. In a word, the transformation process of cis-II seems to be a more favorable route for the transformation of C6H6 and CO2.
A theoretical analysis of the charge transfer process has been performed. Fig. 5 depicts the charges of the benzene skeleton along with the transformation from reactant to product. The charges of the benzene skeleton increased with respect to I and cis-II and decreased with respect to trans-II. The decreased charge decreases the stabilities of intermediates and transition states for trans-II. In contrast, the increased charge enhances the stabilities of intermediates and transition states for I and cis-II. Compared with TS-I and TS-cis-II, the charges of the benzene skeleton in TS1-trans-II and TS2-trans-II are very negative, and large energy barriers have to be overcome to achieve H transfer. Thus, the H elimination/transfer is easier in I and cis-II, than in trans-II. As Ramos and Fernandes stated,66 the charge density of the transition structures is more widely distributed than that of reactants, which favors the reaction to be catalyzed in hydrophobic environments.
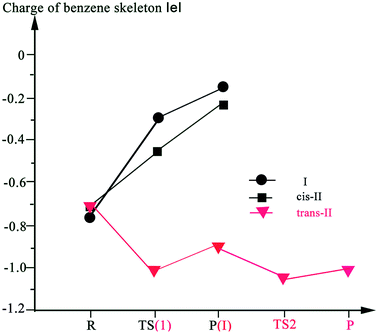 |
| Fig. 5 The charges of benzene skeleton along with the transformation from the reactant to the product. | |
3.3 HCN assistance promoting the transformation
Sajeev stated that the activated CO2 moieties in the weak molecular complexes are long-lived and reactive.55 In this study, we further investigated the reaction for cis-II transformation between the benzene molecule and the long-lived CO2−˙ moiety in the weak molecular complex of [CO2·HCN]−˙. Fig. 6 and 7 depict the process of CO2 attaching to C6H6 and the process of the formation of H2 under HCN molecule assistance, respectively. First, comparing the process without HCN molecule assistance, the activation energy for the process of CO2 attaching to C6H6 increases by 3.9 kcal mol−1 from 7.22 to 11.12 kcal mol−1, this is because the weak molecular complex of CO2·HCN slightly decreases the radical character of the C atom due to the dispersion effect via hydrogen bond interaction. For the same reason, the product [C6H6–CO2–HCN]− has a lower energy of 4.27 kcal mol−1 than the reactants. In the process of H elimination, we calculated that the energy barrier is 36.35 kcal mol−1, which is very close to the 35.70 kcal mol−1 without HCN molecule assistance. Thus, this shows that the formed hydrogen bond complex CO2–HCN did not reduce the energy barrier for the H elimination process. However, the reduction of the energy of TS[NCH–2OC–C6H6–CO2–HCN]2− (18.57 kcal mol−1) with respect to that of the reactant is much larger than TS-cis-II (33.49 kcal mol−1) without HCN molecule assistance. This is due to the dispersion effect of HCN via hydrogen bond interaction. From Fig. 8, the NBO charge of the benzene skeleton of TS[NCH–2OC–C6H6–CO2–HCN]2− (−0.484 |e|) is lower than −0.52 |e| in TS-cis-II without HCN molecule assistance. Clearly, the NBO charge of the benzene skeleton is dispersed to enhance the stability of TS[NCH–2OC–C6H6–CO2–HCN]2−. This point may be significant for investigating other relevant reactions.
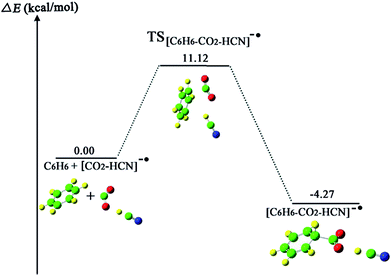 |
| Fig. 6 PES profile for the process of CO2−˙ attaching to C6H6 via HCN assistance. | |
 |
| Fig. 7 Energy profiles for the CO2 transformation reaction via HCN assistance. | |
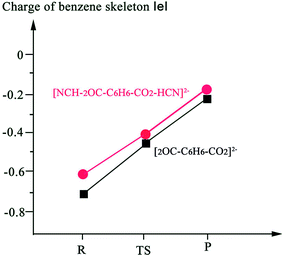 |
| Fig. 8 Comparing the charge of the benzene skeleton with and without HCN assistance. | |
4. Conclusion
In this study, we have revealed a new fixation method of CO2 through excess-electron-induced C–C bond formation using a quantum chemical method. First, by excess electron induction, two CO2 molecules are fixed on a benzene molecule to form two divalent anion complexes [O2C–C6H6–CO2]2− (cis-II and trans-II) via C–C bond formation between the carbon atom of C6H6 and the carbon atom of CO2, due to the distinct radical character at the carbon center in the excess electron species CO2−˙. Furthermore, the transformations of cis-II and trans-II have been predicted. We found that the more favorable transformation of cis-II is to produce terephthalic and one H2 molecule via two H-atom eliminations with an energy barrier of 35.70 kcal mol−1. Furthermore, we found that the long-lived CO2−˙ moiety in the weak molecular complex [CO2·HCN]−˙ also had permanent reactivity to induce C–C bond formation; however, it did not reduce the energy barrier. However, it could reduce the energy of the transition state with respect to that of the reactant, due to its ability to disperse the charge of the benzene ring. As we expect, this study may encourage further related research into CO2 transformation.
Acknowledgements
This study was supported by the National Natural Science Foundation of China (No. 21303065).
References
- C. Costentin, M. Robert and J. M. Saveant, Chem. Soc. Rev., 2013, 42, 2423–2436 RSC.
- D. J. Darensbourg, Inorg. Chem., 2010, 49, 10765–10780 CrossRef CAS PubMed.
- M. Mikkelsen, M. Jørgensen and F. C. Krebs, Energy Environ. Sci., 2010, 3, 43–81 CAS.
- C. Song, Catal. Today, 2006, 115, 2–32 CrossRef CAS.
- M. Aresta, Carbon Dioxide as Chemical Feedstock, Wiley-VCH, Weinheim, 2010 Search PubMed.
- R. Zevenhoven, S. Eloneva and S. Teir, Catal. Today, 2006, 115, 73–79 CrossRef CAS.
- T. Sakakura, J. Choi and H. Yasuda, Chem. Rev., 2007, 107, 2365–2387 CrossRef CAS PubMed.
- I. Omae, Catal. Today, 2006, 115, 33–52 CrossRef CAS.
- E. E. Benson, C. P. Kubiak, A. J. Sathrum and J. M. Smieja, Chem. Soc. Rev., 2009, 38, 89–99 RSC.
- K. Huang, C. Sun and Z. Shi, Chem. Soc. Rev., 2011, 40, 2435–2452 RSC.
- I. Castro-Rodriguez, H. Nakai, L. N. Zakharov, A. L. Rheingold and K. Meyer, Science, 2004, 305, 1757–1759 CrossRef CAS PubMed.
- S. N. Riduan and Y. Zhang, Dalton Trans., 2010, 39, 3347–3357 RSC.
- M. Aresta and A. Dibenedetto, Dalton Trans., 2007, 2975–2992 RSC.
- J. Louie, Curr. Org. Chem., 2005, 9, 605–623 CrossRef CAS.
- W. H. Bernskoetter and B. T. Tyler, Organometallics, 2011, 30, 520–527 CrossRef CAS.
- A. Graët, L. Sinault, M. B. Fusaro, A.-L. Vallet, C. Seu, J. L. Kilgore and M. M. Baum, Organometallics, 2010, 29, 1997–2000 CrossRef.
- M. Takimoto, M. Kawamura and M. Mori, Org. Lett., 2003, 5, 2599–2601 CrossRef CAS PubMed.
- M. Takimoto, M. Kawamura and M. Mori, Synthesis, 2004, 791–795 CAS.
- D. C. Graham, C. Mitchell, M. I. Bruce, G. F. Metha, J. H. Bowie and M. A. Buntine, Organometallics, 2007, 26, 6784–6792 CrossRef CAS.
- M. Aresta, C. Pastore, P. Giannoccaro, G. Kovács, A. Dibenedetto and I. Pápai, Chem.–Eur. J., 2007, 13, 9028–9034 CrossRef CAS PubMed.
- C. Bruckmeier, M. W. Lehenmeier, R. Reichhardt, S. Vagin and B. Rieger, Organometallics, 2010, 29, 2199–2202 CrossRef CAS.
- M. Kawamura and M. Mori, J. Am. Chem. Soc., 2002, 124, 10008–10009 CrossRef.
- K. Shimizu, M. Takimoto, M. Mori and Y. Sato, Synlett, 2006, 3182–3184 CAS.
- C. M. Williams, J. B. Johnson and T. Rovis, J. Am. Chem. Soc., 2008, 130, 14936–14937 CrossRef CAS PubMed.
- J. Louie, J. E. Gibby, M. V. Farnworth and T. N. Tekavec, J. Am. Chem. Soc., 2002, 124, 12188–12189 CrossRef.
- T. N. Tekavec, A. M. Arif and J. Louie, Tetrahedron, 2004, 60, 7431–7437 CrossRef CAS.
- R. Johansson, M. Jaranmark and O. F. Wendt, Organometallics, 2005, 24, 4500–4502 CrossRef CAS.
- J. Wu and N. Hazari, Chem. Commun., 2011, 47, 1069–1071 RSC.
- K. Ukai, M. Aoki, J. Takaya and N. Iwasawa, J. Am. Chem. Soc., 2006, 128, 8706–8707 CrossRef CAS PubMed.
- R. Johansson and O. F. Wendt, Dalton Trans., 2007, 488–492 RSC.
- F. Huang, G. Lu, L. L. Zhao, H. X. Li and Z. X. Wang, J. Am. Chem. Soc., 2010, 132, 12388 CrossRef CAS PubMed.
- A. Jansen and S. Pitter, J. Mol. Catal. A: Chem., 2004, 217, 41–45 CrossRef CAS.
- J. Takaya, S. Tadami, K. Ukai and N. Iwasawa, Org. Lett., 2008, 10, 2697–2700 CrossRef CAS PubMed.
- L. Dang, Z. Lin and T. Marder, Organometallics, 2010, 29, 917–927 CrossRef CAS.
- R. Tanaka, M. Yamashita and K. Nozaki, J. Am. Chem. Soc., 2009, 131, 14168–14169 CrossRef CAS PubMed.
- C. Federsel, R. Jackstell, A. Boddien, G. Laurenczy and M. Beller, ChemSusChem, 2010, 3, 1048–1050 CrossRef CAS PubMed.
- C.-X. Miao, J.-Q. Wang, Y. Wu, Y. Du and L.-N. He, ChemSusChem, 2008, 1, 236–241 CrossRef CAS PubMed.
- A. Decortes and A. W. Kleij, ChemCatChem, 2011, 3, 831–834 CrossRef CAS.
- D. J. Darensbourg, Inorg. Chem., 2010, 49, 10765–10780 CrossRef CAS PubMed.
- M. R. Kember, A. Buchard and C. K. Williams, Chem. Commun., 2011, 47, 141–163 RSC.
- G. W. Coates and D. R. Moore, Angew. Chem., Int. Ed., 2004, 116, 6784–6806 CrossRef.
- A. Parkin, J. Seravalli, K. A. Vincent, S. W. Ragsdale and F. Armstrong, J. Am. Chem. Soc., 2007, 129, 10328–10329 CrossRef CAS PubMed.
- W. Shin, S. H. Lee, J. W. Shin, S. P. Lee and Y. Kim, J. Am. Chem. Soc., 2003, 125, 14688–14689 CrossRef CAS PubMed.
- H. Hoberg and S. Schaefer, J. Organomet. Chem., 1983, 251, C51–C53 CrossRef CAS.
- H. Hoberg, K. Jenni, C. Krüger and E. Raabe, Angew. Chem., Int. Ed., 1986, 25, 810–811 CrossRef.
- Y. Sasaki, Y. Inoue and H. Hashimoto, J. Chem. Soc., Chem. Commun., 1976, 605–606 RSC.
- A. Musco, C. Perego and V. Tartiari, Inorg. Chim. Acta, 1978, 28, L147–L148 CrossRef CAS.
- A. Behr, K. D. Juszak and W. Keim, Synthesis, 1983, 574–575 CrossRef CAS.
- S. H. Lee, N. Kim, D. G. Ha and S. K. Kim, J. Am. Chem. Soc., 2008, 130, 16241–16244 CrossRef CAS PubMed.
- S. Y. Han, I. Chu, J. H. Kim, J. K. Song and S. K. Kim, J. Chem. Phys., 2000, 113, 596 CrossRef CAS.
- T. Hamann, E. Böhler and P. Swiderek, Angew. Chem., Int. Ed., 2009, 48, 1–4 CrossRef PubMed.
- S. N. Eustis, D. Radisic, K. H. Bowen, R. A. Bachorz, M. Haranczyk, G. K. Schenter and M. Gutowski, Science, 2008, 319, 936–939 CrossRef CAS PubMed.
- D. Davis, V. P. Vysotskiy, Y. Sajeev and L. S. Cederbaum, Angew. Chem., Int. Ed., 2011, 50, 4119–4122 CrossRef CAS PubMed.
- D. Schröder, C. A. Schalley, J. N. Harvey and H. Schwarz, Int. J. Mass Spectrom., 1999, 25, 185–187 Search PubMed.
- D. Davis and Y. Sajeev, Phys. Chem. Chem. Phys., 2014, 16, 17408–17411 RSC.
- R. F. Höckendorf, Q. Hao, Z. Sun, B. S. Fox-Beyer, Y. Cao, O. P. Balaj, V. E. Bondybey, C.-K. Siu and M. K. Beyer, J. Phys. Chem. A, 2012, 116, 3824–3835 CrossRef PubMed.
- O. P. Balaj, C.-K. Siu, I. Balteanu, M. K. Beyer and V. E. Bondybey, Chem.–Eur. J., 2004, 10, 4822–4830 CrossRef CAS PubMed.
- C. van der Linde, A. Akhgarnusch, C.-K. Siu and M. K. Beyer, J. Phys. Chem. A, 2011, 115, 10174–10180 CrossRef CAS PubMed.
- A. Akhgarnusch, R. F. Höckendorf, Q. Hao, K. P. Jäger, C.-K. Siu and M. K. Beyer, Angew. Chem., 2013, 125, 9497–9500 CrossRef.
- M. J. Frisch, G. W. Trucks, H. B. Schlegel, G. E. Scuseria, M. A. Robb, J. R. Cheeseman, G. Scalmani, V. Barone, B. Mennucci, G. A. Petersson, H. Nakatsuji, M. Caricato, X. Li, H. P. Hratchian, A. F. Izmaylov, J. Bloino, G. Zheng, J. L. Sonnenberg, M. Hada, M. Ehara, K. Toyota, R. Fukuda, J. Hasegawa, M. Ishida, T. Nakajima, Y. Honda, O. Kitao, H. Nakai, T. Vreven, J. A. Montgomery Jr, J. E. Peralta, F. Ogliaro, M. Bearpark, J. J. Heyd, E. Brothers, K. N. Kudin, V. N. Staroverov, R. Kobayashi, J. Normand, K. Raghavachari, A. Rendell, J. C. Burant, S. S. Iyengar, J. Tomasi, M. Cossi, N. Rega, J. M. Millam, M. Klene, J. E. Knox, J. B. Cross, V. Bakken, C. Adamo, J. Jaramillo, R. Gomperts, R. E. Stratmann, O. Yazyev, A. J. Austin, R. Cammi, C. Pomelli, J. Ochterski, R. L. Martin, K. Morokuma, V. G. Zakrzewski, G. A. Voth, P. Salvador, J. J. Dannenberg, S. Dapprich, A. D. Daniels, O. Farkas, J. B. Foresman, J. V. Ortiz, J. Cioslowski and D. J. Fox, Gaussian 09 (Revision A.2), Gaussian, Inc., Wallingford, CT, 2009 Search PubMed.
- V. Barone, M. Cossi and J. Tomasi, J. Comput. Chem., 1998, 19, 404–417 CrossRef CAS.
- Y. Takano and K. N. Houk, J. Chem. Theory Comput., 2005, 1, 70–77 CrossRef PubMed.
- A. E. Reed, R. B. Weinstock and F. Weinhold, J. Chem. Phys., 1985, 83, 735–746 CrossRef CAS.
- J. E. Carpenter and F. J. Weinhold, J. Mol. Struct.: THEOCHEM, 1988, 169, 41–62 CrossRef.
- E. D. Glendenig, J. K. Badenhoop, A. E. Reed, J. E. Carpenter, J. A. Bohmann, C. M. Morales and F. Weinhold, NBO 5.0, Theoretical Chemistry Institute, University of Wisconsin, Madison, 2001 Search PubMed.
- P. A. Fernandes and M. J. Ramos, Chem.–Eur. J., 2004, 10, 257–266 CrossRef CAS PubMed.
|
This journal is © The Royal Society of Chemistry 2016 |
Click here to see how this site uses Cookies. View our privacy policy here.