DOI:
10.1039/C5PY01634G
(Paper)
Polym. Chem., 2016,
7, 44-53
Effects of a trans- or cis-cyclohexane unit on the thermal and rheological properties of semi-aromatic polyamides†
Received
9th October 2015
, Accepted 4th November 2015
First published on 4th November 2015
Abstract
Semi-aromatic monomers containing a cyclohexane unit, prepared using a facile interfacial reaction method from 1,4-cyclohexanediamine (trans- or cis-) and 4-fluorobenzoyl chloride (4-FBC) at room temperature, were reacted with 1,1-bis(4-hydroxyphenyl)-1-phenylethane (BHPPE) to afford two kinds of semi-aromatic polyamide (BH-trans-BFCD and BH-cis-BFCD) in this work. An investigation of thermal properties confirmed high glass transition temperatures (Tg) of 224–265 °C and good thermal stability with initial degradation temperatures (Td) of 445 to 450 °C for the synthesized semi-aromatic polyamides, which were determined by differential scanning calorimetry (DSC) and thermogravimetric analysis (TGA) characterization. It was found that the properties (thermal and mechanical) of BH-trans-BFCD, containing a trans-conformation, were much better than those of BH-cis-BFCD, containing a cis-conformation. Comparing the thermal stability of the resultant semi-aromatic polyamides with a commercial product, PA6T-Dupont, the prepared polyamides treated at 220 °C for 7 h displayed a slightly reduced tensile strength as well as a smaller change in color. According to rheological testing, they were found to have better melt flowability (complex viscosities ranged from 210 to 3070 Pa s at 310 °C to 360 °C). Additionally, their thermal degradation kinetics and the thermal pyrolysis mechanism of these semi-aromatic polyamides were investigated by thermogravimetric analysis and Pyrolysis/Gas Chromatography/Mass (Py-GC/MS) analysis, which further indicated their higher thermal stability and processability.
Introduction
Nylon exhibits outstanding properties such as good mechanical performance and melting processability due to its good chain regularity and high content of strong hydrogen bonds.1,2 However, its moderate thermal properties limit its wide application especially when it is used in surface mount technology (SMT) and so on. In order to improve the thermal properties of aliphatic polyamides, incorporation of aromatic units into the polymer main chain is an efficient approach.3–7 Aromatic polyamides (aramids), such as Aramid-1414 and Aramid-1313, well-known for their excellent thermal, mechanical and corrosion resistance properties, are seen as one of the irreplaceable special engineering plastics,8,9 but have a drawback of poor processability. Therefore, semi-aromatic polyamides combining the high long-term use temperature and heat resistance of wholly aromatic polyamides with the good melting processability of nylon are desirable. However, only a few semi-aromatic polyamides, such as poly(nonamethylene terephthalamide) (PA9T),10–14 poly(decamethylene terephthalamide) (PA10T)15,16 and copolymers of PA6T/66, PA6T/6 and so on,17 are commercial and processable. Usually, semi-aromatic polyamides, e.g. PA4T, homopolymer of PA6T, and so on (as shown in Table 1) which contain a short chain (less than 7 methylenes) diamine are found to have poor solubility and possess a higher melt temperature than thermal degradation temperature. They are thought to be impossible to thermally process. Therefore, in the past decade, much attention has focused on chemical modification of semi-aromatic polyamides to improve their processability such as the incorporation of a cyclodextrin unit,18 sulfide groups,19 ester unit20,21 and long carbon chains22–27 [PA-10T, PA-12T and PA-18T (Tm = 245 °C) and so on] into the polymer backbone to decrease their melt temperature and improve their melt processability, or the introduction of noncoplanar biphenylene moieties,28 hyperbranched units,29,30 bulky pendant groups,31–33 triazole units,34 sulphone units35 and azo groups36,37 into the polymer main chain to weaken its molecular regularity in order to improve its solution processability.
Table 1 The melt temperature (Tm) and thermal degradation temperature (Td) of traditional semi-aromatic polyamides
Polymer |
T
m (°C) |
T
d (°C) |
Polymer |
T
g (°C) |
T
m (°C) |
T
d (°C) |
PA4T |
430 |
350 |
PA18T |
— |
245 |
— |
PA6T (homopolymer) |
370 |
350 |
Semi-aromatic PA (with bulky pendant groups) |
270 |
— |
500 |
PA9T |
305 |
464 |
Semi-aromatic PA (with biphenylene unit) |
300 |
— |
510 |
PA10T |
315 |
472 |
Semi-aromatic PA (with naphthalene ring) |
— |
300 |
495 |
PA12T |
295 |
429 |
Semi-aromatic PA (with ester unit) |
110–140 |
219–260 |
— |
Additionally, the durability of commercial semi-aromatic polyamides such as the copolymer of PA6T/66 at high temperature is found to be poor. The color of the product becomes deep, and its tensile strength decreases gradually with treatment time under high temperature. Therefore, the aim of this work was to synthesize well-designed semi-aromatic polyamides with pronounced processability, mechanical and thermal properties. In this case, we introduced a monomer containing a stable cyclohexane unit (cis- or trans-conformation) into the polymer chain to investigate the polymers’ thermal and rheological properties. On the other hand, the incorporation of bulky side groups is expected to reduce inter-chain interactions and reduce the polymers’ viscosity, so the bisphenol BHPPE containing a bulky side group was reacted with BFCD through a nucleophilic substitution reaction in this work. Finally, the effects of different conformations on these semi-aromatic polyamides’ thermal, rheological properties, degradation kinetics and mechanism were investigated in detail.
Results and discussion
Synthesis and chemical structure of monomers (trans-BFCD and cis-BFCD)
The semi-aromatic monomers containing a cyclohexane unit were synthesized with a facile interfacial reaction using dichloromethane and water as solvents. In order to avoid the generation of the mono-substitution byproduct of N-(4-fluorobenzoyl) trans- or cis-cyclohexanediamine, during the reaction procedure, 4-FBC was always kept in excess by adding 1,4-cyclohexanediamine into the solution of 4-FBC. This is beneficial for getting a high yield and purity of the product. Fig. 1 is the FT-IR spectra of the monomers, amide absorptions can be found near 3300 and 1635 cm−1, methylene absorptions near 2930 and 2850 cm−1, benzene ring (C
C) absorptions near 1600 and 1490 cm−1, and para-substituted benzene ring absorptions near 850 cm−1. The characteristic absorption of C–N in cis-BFCD (1233 cm−1) is found to be much stronger than that of trans-BFCD (1228 cm−1). The main reason is that trans-BFCD has much higher symmetry; the bond of C–N is affected more by the carbonyl unit, so the bond of C–N is weakened. The FT-IR results suggest that the amidation reaction of 4-FBC and 1,4-cyclohexanediamine occurred. Fig. 2 shows the 1H-NMR spectra of trans-BFCD and cis-BFCD. The proton signals of cyclohexane units range from 1.42 to 3.88 ppm. The signals in the range of 7.26–7.95 ppm are attributed to the protons of the benzene rings. The proton signal of –CONH– is a double peak at 8.084–8.100 ppm (cis-BFCD) or at 8.287–8.307 ppm (trans-BFCD). The chemical shift of trans-BFCD is larger when compared to cis-BFCD. It is ascribed to the higher content of hydrogen bonds in trans-BFCD due to its higher symmetry than cis-BFCD (as shown in Fig. 3), which causes the proton signal of N–H to shift to a lower field. The chemical structure characterizations indicate that the monomers are synthesized as shown in Scheme 1.
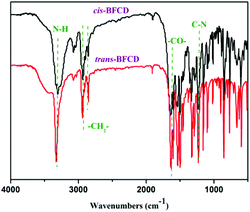 |
| Fig. 1 The FT-IR spectra of trans-BFCD and cis-BFCD. | |
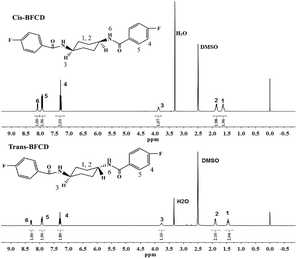 |
| Fig. 2 The 1H-NMR spectra of trans-BFCD and cis-BFCD. | |
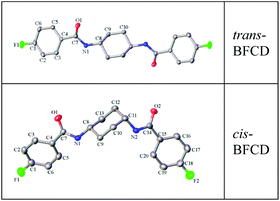 |
| Fig. 3 The molecular structure of trans-BFCD and cis-BFCD tested using four-circle single-crystal diffraction. | |
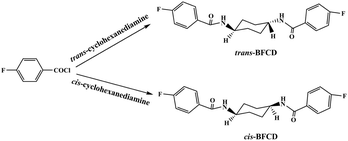 |
| Scheme 1 Synthesis route of trans-BFCD and cis-BFCD. | |
Synthesis and chemical structure of polymers (BH-trans-BFCD and BH-cis-BFCD)
Two kinds of novel semi-aromatic polyamides were synthesized through the reaction of bisphenol (BHPPE) and BFCD. At the prepolymerization stage, a low reaction temperature was applied to give water as a byproduct, then a high temperature was maintained to yield high molecular weight polyamides in a polycondensation step. The molecular weights of the resultant semi-aromatic polyamides were characterized by intrinsic viscosity measurements and GPC, which are listed in Table 2. It is found that the molecular weight of BH-trans-BFCD is larger than that of BH-cis-BFCD. The rate of the polymerization reaction was evaluated by a model reaction of trans-BFCD or cis-BFCD with phenol (see ESI†). The monomer of trans-BFCD was found to have almost the same reaction rate as cis-BFCD. The difference in viscosity of BH-trans-BFCD and BH-cis-BFCD may be based on their difference in hydrodynamic radius. FT-IR spectra of BH-trans-BFCD and BH-cis-BFCD are shown in Fig. 4. The characteristic absorptions of –CONH–, –CH2– and benzene rings were similar to those of the monomers. The absorption of C–F near 1330 cm−1 almost disappeared while two new characteristic absorptions near 1172 cm−1 and 2960 cm−1, which were attributed to the absorptions of the ether bond and methyl unit, could be observed. This suggests the nucleophilic substitution reaction of C–F and –OH has occurred. Fig. 5 is the 1H-NMR spectrum of BH-trans-BFCD. The proton signals of the aliphatic chain are in the range of 1.4 to 3.8 ppm. The single peak at 2.1 ppm was attributed to the protons of –CH3. The aromatic ring proton signals appeared in the range of 6.9–7.9 ppm. They were too close to be separated well. But the ratio of integrated proton signals was about 4
:
4
:
3
:
2
:
4
:
4
:
4
:
2
:
1
:
2
:
4
:
2, which was identical with the calculated result. The structure of BH-cis-BFCD was also confirmed by its 1H-NMR spectrum (as shown in Fig. 6). Compared with the 1H-NMR spectrum of BH-trans-BFCD, it was found that the proton signal of N–H showed a similar chemical shift trend to the monomers, again because of the stronger role of hydrogen bonding in BH-trans-BFCD than BH-cis-BFCD. The chemical structure characterizations indicated the polymers are synthesized as shown in Scheme 2.
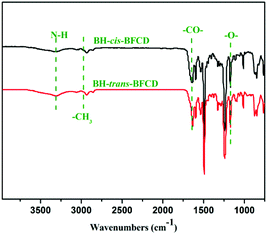 |
| Fig. 4 The FT-IR spectra of BH-trans-BFCD and BH-cis-BFCD. | |
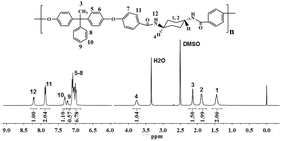 |
| Fig. 5 The 1H-NMR spectrum of BH-trans-BFCD. | |
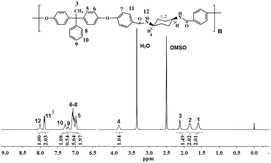 |
| Fig. 6 The 1H-NMR spectrum of BH-cis-BFCD. | |
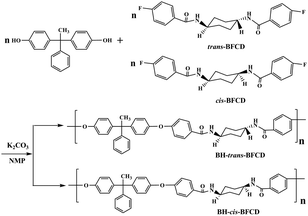 |
| Scheme 2 Synthesis route of BH-trans-BFCD and BH-cis-BFCD. | |
Table 2 Intrinsic viscosities (ηint) and molecular weights of BH-trans-BFCD and BH-cis-BFCD
Polyamide |
η
int (dL g−1) |
M
n (g mol−1) |
M
w (g mol−1) |
PDI (Mw/Mn) |
BH-trans-BFCD |
1.17 |
7.40 × 104 |
1.61 × 105 |
2.18 |
BH-cis-BFCD |
0.93 |
6.12 × 104 |
1.46 × 105 |
2.39 |
Thermal analysis of BH-trans-BFCD and BH-cis-BFCD
The thermal properties of the resultant semi-aromatic polyamides were first tested with DSC. As shown in Fig. 7, obvious glass transition temperatures (Tg) of BH-trans-BFCD and BH-cis-BFCD at around 224 and 265 °C, respectively, could be observed. The Tg of BH-trans-BFCD is about 40 °C higher than that of BH-cis-BFCD. The main reason is the low inter-chain interactions caused by loose packing of the polymer chains and less hydrogen bonding of BH-cis-BFCD. No melting peak was found for both polymers from the DSC curves indicating that they are both amorphous. Fig. 8 shows the TGA curves of BH-trans-BFCD and BH-cis-BFCD tested at different heating rates (5, 10, 20 and 40 °C min−1) under a nitrogen atmosphere. The starting thermal degradation temperature (Td5%) of BH-trans-BFCD and BH-cis-BFCD was 450 and 445 °C, respectively. The char yield of BH-trans-BFCD and BH-cis-BFCD at 800 °C in nitrogen was about 11.1 and 10.7 wt%, respectively. In order to investigate the difference of the thermal decomposition behaviour of these semi-aromatic polyamides, a differential method (Kissinger method) was applied to calculate their thermal degradation kinetics. The Kissinger method can be used to calculate the activation energy (E), degradation order (n) and pre-exponential factor ln
A,38 and does not need to know the degradation mechanism beforehand, with the following equation eqn (1): |  | (1) |
where β is the heating rate, Tmax is the maximum thermal degradation temperature, n is the reaction order, αmax is the maximum conversion and A is the pre-exponential factor. The activation energy E can be calculated according to the slope of the plot of ln(β/Tmax2) versus 1000/Tmax. The values of initial decomposition temperature (Td5%) and the maximum thermal degradation temperature (Tmax) are shown in Table 3. Fig. 9 shows the curves of the Kissinger method applied to the experimental data at different heating rates. The values (as shown in Table 4) obtained from Fig. 9 for the activation energy (E) of BH-trans-BFCD and BH-cis-BFCD were 240.9 and 235.2 kJ mol−1, respectively. BH-trans-BFCD displayed the highest thermal degradation activation energy among these semi-aromatic polyamides, suggesting it exhibits the best thermal stability. Compared with BH-cis-BFCD, the molecular chain of BH-trans-BFCD consists of much more close packing and hydrogen bonding than that of BH-cis-BFCD, so BH-trans-BFCD shows the most stable thermal stability.
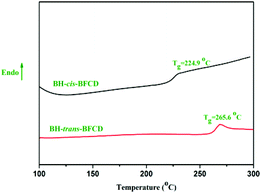 |
| Fig. 7 DSC curves of BH-trans-BFCD and BH-cis-BFCD. | |
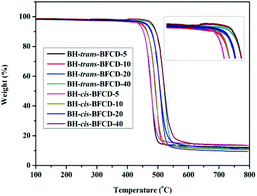 |
| Fig. 8 TGA curves of BH-trans-BFCD and BH-cis-BFCD at different heating rates. | |
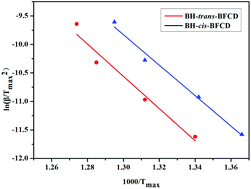 |
| Fig. 9 The Kissinger method applied to experimental data at different heating rates of BH-trans-BFCD and BH-cis-BFCD. | |
Table 3 The initial and maximum thermal degradation temperatures of BH-trans-BFCD and BH-cis-BFCD
Heating rate |
BH-trans-BFCD |
BH-cis-BFCD |
T
d5% (°C) |
T
max (°C) |
T
d5% (°C) |
T
max (°C) |
5 °C min−1 |
438 |
480 |
438 |
473 |
10 °C min−1 |
450 |
494 |
445 |
489 |
20 °C min−1 |
464 |
509 |
459 |
505 |
40 °C min−1 |
482 |
520 |
477 |
512 |
Table 4 The slope, intercept of the fitting line and calculated value of E of BH-trans-BFCD and BH-cis-BFCD
Polymer |
Fitting line slope |
Fitting line intercept |
Activation energy E (kJ mol−1) |
BH-trans-BFCD |
−28.979 |
26.816 |
240.9 |
BH-cis-BFCD |
−28.284 |
26.210 |
235.2 |
Thermal degradation mechanism
Py-GC/MS was used to characterize the thermal degradation mechanism of BH-trans-BFCD, BH-cis-BFCD and PA6T-Dupont under a nitrogen atmosphere at 550 °C and 650 °C. As shown in Fig. 10, four kinds of degradation compounds of BH-trans-BFCD and BH-cis-BFCD including benzene, toluene, ethylbenzene and phenol were found at 550 °C. This was attributed to polymer side chain decomposition and the further degradation of diphenol units. This was quite different from the decomposition routes of PA6T-Dupont. The degradation compounds of PA6T-Dupont at 550 °C consisted of cyclopentanone, benzonitrile, methylbenzonitrile, hexanedinitrile and 1,4-benzendicarbonitrile. The polymer main chain of PA6T-Dupont began to decompose from the amide unit while BH-trans-BFCD and BH-cis-BFCD began to degrade from the side chain of the BHPPE unit. So BH-trans-BFCD (or BH-cis-BFCD) showed higher thermal stability than PA6T-Dupont. This agreed with the results of the thermal degradation kinetics analysis. The degradation compounds of BH-trans-BFCD, BH-cis-BFCD and PA6T-Dupont consisted of up to nine kinds of products when the pyrolysis temperature was raised to 650 °C. Comparing with the first pyrolysis step, BH-trans-BFCD decomposed into many more products with the increase of pyrolysis temperature such as benzonitrile, diphenyl ether, diphenylmethane, 1-methyl-4-phenoxybenzene and 4-phenoxybenzonitrile. This indicates that the amide groups begin to degrade with the appearance of compounds which contain a nitrile unit. In other words, this suggests that the polymer main chain of BH-trans-BFCD begins to decompose when the pyrolysis temperature is 650 °C. As summarized in Table 5, it was found that PA6T-Dupont continued to decompose into much smaller pieces (alkyl radicals) and isomerized into 4-methylbenzonitrile, ethylbenzonitrile and 4-ethenylbenzonitrile with an increase of the pyrolysis temperature to 650 °C.
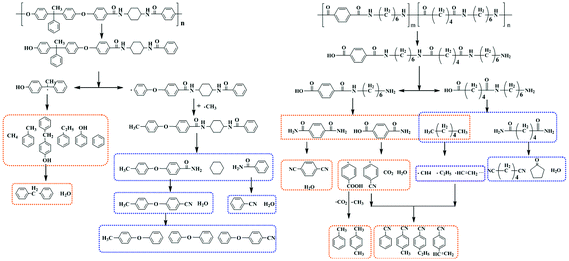 |
| Fig. 10 Thermal degradation routes of BH-trans-BFCD, BH-cis-BFCD and PA6T-Dupont. | |
Table 5 Thermal degradation compounds of BH-trans-BFCD, BH-cis-BFCD and PA6T-Dupont tested by Py-GC/MS (pyrolysis temperatures: 550 and 650 °C)
Retention time (min) |
Pyrolysis compounds |
BH-trans/cis-BFCD |
PA6T-Dupont |
550 °C |
650 °C |
550 °C |
650 °C |
+: Compound was detected; −: Compound was not detected. |
3.1 |
Benzene |
+ |
+ |
− |
− |
4.7 |
Toluene |
+ |
+ |
− |
+ |
5.2 |
Cyclopentanone |
− |
− |
+ |
+ |
6.9 |
Ethylbenzene |
+ |
+ |
− |
+ |
7.1 |
p-Xylene |
− |
− |
− |
+ |
10.6 |
Benzonitrile |
− |
+ |
+ |
+ |
11.0 |
Phenol |
+ |
+ |
− |
− |
14.2 |
4-Methylbenzonitrile |
− |
− |
+ |
+ |
17.0 |
Ethylbenzonitrile |
− |
− |
− |
+ |
17.2 |
Hexanedinitrile |
− |
− |
+ |
+ |
17.6 |
4-Ethenylbenzonitrile |
− |
− |
− |
+ |
19.1 |
1,4-Benzenedicarbonitrile |
− |
− |
+ |
+ |
22.0 |
Diphenyl ether |
− |
+ |
− |
− |
22.7 |
Diphenylmethane |
− |
+ |
− |
− |
24.3 |
1-Methyl-4-phenoxy-benzene |
− |
+ |
− |
− |
28.5 |
4-Phenoxybenzonitrile |
− |
+ |
− |
− |
Mechanical properties
As described in Table 6, the tensile strength and Young's modulus of BH-trans-BFCD and BH-cis-BFCD were 83.6–101.2 MPa and 2.4–2.8 GPa, respectively. BH-trans-BFCD showed better mechanical performance than BH-cis-BFCD. This is mainly ascribed to its high content of hydrogen bonds and molecular chain close packing. We also investigated the tensile strength and color change of BH-trans-BFCD and BH-cis-BFCD which were treated at 220 °C for about 1, 3, 5 and 7 h. Both of the two samples were found to have a decreased tensile strength after treating at 220 °C for a certain period (as shown in Fig. 11). BH-trans-BFCD displayed about a 20 MPa (20%) reduction while a decrease of about 30 MPa (36%) was displayed for BH-cis-BFCD. The reduction magnitude of the tensile strength of BH-cis-BFCD was much larger than that of BH-trans-BFCD. In addition, the tensile strength of BH-trans-BFCD treated at 220 °C for 7 h was found to be still much higher than even untreated BH-cis-BFCD. This indicates that BH-trans-BFCD has a much better tensile strength retention at high temperature than BH-cis-BFCD.
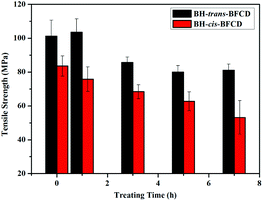 |
| Fig. 11 Tensile strength of BH-trans-BFCD and BH-cis-BFCD treated at 220 °C for 1, 3, 5 and 7 h. | |
Table 6 Tensile and thermal properties of BH-trans-BFCD and BH-cis-BFCD
Polymer |
Tensile strength (MPa) |
Elongation at yield (%) |
Young's modulus (GPa) |
Storage modulus at 220 °C (GPa) |
Glass transition temperaturea (°C) |
Detected by dynamic mechanical analysis (DMA).
|
BH-trans-BFCD |
101.2 |
17.5 |
2.8 |
1.4 |
273.7 |
BH-cis-BFCD |
83.6 |
20.2 |
2.4 |
1.2 |
238.4 |
In order to investigate this phenomenon, PLM was carried out to study the samples’ aggregation structure (as shown in Fig. 12). From the PLM images of BH-trans-BFCD (annealed at 280 °C for 1 h, 3 h and 5 h under vacuum conditions) and BH-cis-BFCD (annealed at 280 °C for 5 h under vacuum conditions), we observed a micro-fibrous structure. And this micro-fibrous structure got increasingly obvious. But this phenomenon can not be found in the sample of BH-cis-BFCD. So BH-trans-BFCD had a better tensile strength than BH-cis-BFCD. As shown in Fig. 13, the color of BH-cis-BFCD and BH-trans-BFCD became deep but when compared with BH-cis-BFCD the color change of BH-trans-BFCD was slighter. The deeper color is caused by the content changes of the chromophore contained in the compounds. With high temperature treatment, BH-cis-BFCD became unstable, and generated more chromophore units than BH-trans-BFCD. Above all, the tensile strength and color change experiments suggest that the mechanical properties and high temperature stability of BH-trans-BFCD are much better than BH-cis-BFCD. This was in agreement with the results of the thermal degradation analysis.
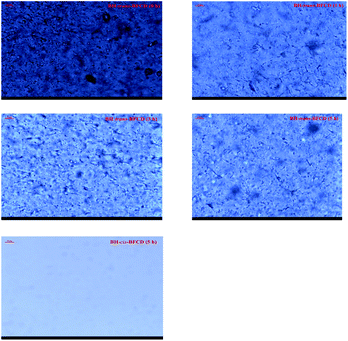 |
| Fig. 12 The PLM images of BH-trans-BFCD and BH-cis-BFCD treated at 280 °C under vacuum for 1, 3 and 5 h. | |
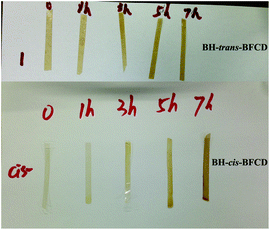 |
| Fig. 13 The color change of BH-trans-BFCD and BH-cis-BFCD treated at 220 °C for 1, 3, 5 and 7 h. | |
Dynamic mechanical analysis (DMA) was also used to characterize the thermal mechanical properties of BH-trans-BFCD and BH-cis-BFCD. As shown in Fig. 14, the glass transition temperatures of BH-trans-BFCD and BH-cis-BFCD were about 273 and 238 °C, respectively. BH-trans-BFCD displayed a much higher Tg than BH-cis-BFCD, which was consistent with the results of DSC measurement. From the storage modulus curves (Fig. 14) of the two semi-aromatic polyamides, it was found that the prepared polymers had good thermal mechanical properties. Their storage modulus only decreased a little from 50 °C to the glass transition temperature of the polymers. BH-trans-BFCD and BH-cis-BFCD showed a storage modulus of about 1.3 GPa (at 250 °C) and 1.2 GPa (at 220 °C), respectively. This suggests that the prepared semi-aromatic polyamides possess good thermal mechanical properties.
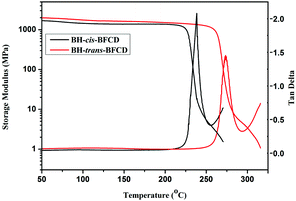 |
| Fig. 14 DMA curves (tan delta and storage modulus) of BH-trans-BFCD and BH-cis-BFCD. | |
Rheological properties of polymers
The rheological properties of the resultant semi-aromatic polyamides were characterized with a parallel plate rheometer. In order to investigate the suitable melting process window of the two semi-aromatic polyamides, a temperature sweep (as shown in Fig. 15 and 16) was conducted to analyse their complex viscosity, storage modulus (G′) and loss modulus (G′′). Fig. 15 shows that the complex viscosity of BH-cis-BFCD is much lower. The main reason is that the cis-chemical structure of BH-cis-BFCD displays a much larger molecular volume than BH-trans-BFCD. So BH-cis-BFCD shows a much lower viscosity out of these two samples. As shown in Fig. 16, the melt loss modulus (G′′) of BH-cis-BFCD was almost larger than the storage modulus (G′) at the tested temperatures. It also found that the melt loss modulus of BH-trans-BFCD was larger than the storage modulus at about 305 °C. It suggests that these two samples can be melt processed above this temperature (the intersection point of G′ and G′′).
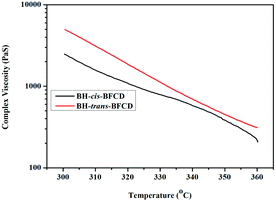 |
| Fig. 15 Plot of complex viscosities versus temperature for BH-trans-BFCD and BH-cis-BFCD. | |
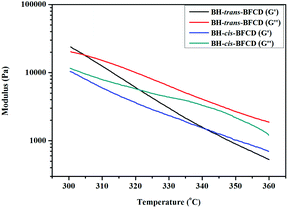 |
| Fig. 16 Storage modulus and loss modulus curves versus temperature for BH-trans-BFCD and BH-cis-BFCD. | |
As shown in Fig. 17 and 18, the time sweep was used to calculate the melt stability of these samples. The complex viscosity of BH-trans-BFCD was almost unchanged from the beginning to the end of the experiment, while it increased gradually for the BH-cis-BFCD. The main reason for the above phenomenon is that it is easy for oxygen to permeate into the molecular chain of BH-cis-BFCD, which has a loose chemical structure. Then, the probability of an oxidation reaction gets much higher. Also the melt storage modulus (G′) and loss modulus value (G′′) of BH-cis-BFCD and BH-trans-BFCD were investigated (as shown in Fig. 18). The values of G′′ of BH-cis-BFCD and BH-trans-BFCD were always larger than G′. The intersection point of the melt storage modulus (G′) and loss modulus value (G′′) did not appear during the whole testing period. This suggests that BH-cis-BFCD and BH-trans-BFCD display a similarly good melt stability.
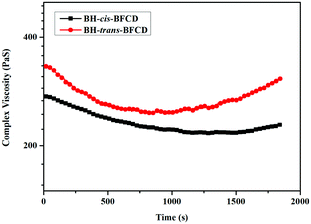 |
| Fig. 17 Plot of complex viscosity versus time (at 330 °C) for BH-trans-BFCD and BH-cis-BFCD. | |
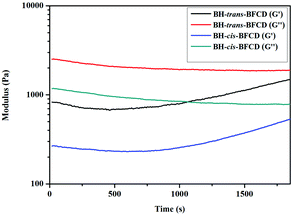 |
| Fig. 18 Storage modulus and loss modulus curves versus temperature for BH-trans-BFCD and BH-cis-BFCD. | |
Conclusions
Semi-aromatic amide monomers containing a stable trans- or cis-cyclohexane unit were synthesized with a facile interfacial reaction at ambient temperature. They were then used to react with BHPPE with a bulky group by nucleophilic polycondensation to synthesize two kinds of semi-aromatic polyamide, named BH-trans-BFCD and BH-cis-BFCD. It was found that the glass transition temperature, thermal stability and melting stability of BH-trans-BFCD were much higher than those of BH-cis-BFCD because of the close packing and large content of hydrogen bonds of the polymer chain of BH-trans-BFCD. Also the sample of BH-trans-BFCD was found to have better mechanical properties than BH-cis-BFCD because of its micro-fibrous structure. Additionally, the resultant semi-aromatic polyamides displayed high thermal stability when compared with the commercial material PA6T-Dupont. Therefore, these semi-aromatic polyamides could be potentially used as heat resistant thermoplastic materials, especially when a thin-walled work-piece and excellent durability are required.
Experimental
Materials
N-Methyl-2-pyrrolidone (NMP) (ShanDong YuNeng Chemical Industry Company), 4-FBC (99.5%, Lanning Chemical Company Limited), sodium hydroxide (NaOH) (AR, KeLong Chemical Reagent Company), 1,4-trans-cyclohexanediamine (99%, Beisite Chemical Reagent Company), 1,4-cis-cyclohexanediamine (99%, Fuersite Chemical Reagent Company), PA6T-Dupont (DuPont Company, 18502 NC010) and other reagents were obtained commercially. BHPPE was synthesized as reported earlier by our group.39
Monomer synthesis
N,N′-Bis(4-fluorobenzoyl) trans-cyclohexanediamine (trans-BFCD) (as shown in Scheme 1).
Firstly, a 1 L mixture of dichloromethane and 4-FBC (317 g, 2 mol) was added into a 5 L three-necked round bottom flask. Next, a solution of 1,4-trans-cyclohexanediamine (114 g, 1 mol), NaOH (80 g, 2 mol) and sodium dodecyl sulfate (3 g) dissolved in deionized water (1000 ml) was added dropwise over 2 h. Then the reaction was kept at ambient temperature under stirring for about 8 h to yield a white mixture. Afterwards, the mixture was evaporated at 50 °C to recover the solvent CH2Cl2. Then, the slurry mixture was filtered to collect the solid crude product. The crude product was washed with hot deionized water three times to remove the byproduct and unreacted reagent. Then, it was recrystallized from N,N-dimethylformamide (DMF) to form needle-like crystals. Finally, it was dried at 80 °C in an oven under vacuum. Yield: 311.1 g (86.9%). FT-IR (KBr, cm−1): 3328 cm−1 (N–H), 1631 cm−1 (–CO–), 3074 cm−1 (C–H of aromatic ring), 2929, 2852 cm−1 (–CH2–), 1601, 1499 cm−1 (C
C of aromatic ring), 1228 cm−1 (C–N), 855 cm−1 (para substitution of the benzene ring). 1H-NMR [400 MHz, deuterated DMSO-d6/TMS, ppm]: 1.428–1.477 (t, 4H, H1), 1.891–1.907 (d, 4H, H2), 3.764 (s, 2H, H3), 7.269–7.313 (t, 4H, H4), 7.901–7.936 (m, 4H, H5), 8.287–8.307 (d, 2H, H6).
N,N′-Bis(4-fluorobenzoyl) cis-cyclohexanediamine (cis-BFCD).
1,4-cis-BFCD was obtained by following the same general synthesis procedure as trans-BFCD after the reaction of 4-FBC with 1,4-cis-cyclohexanediamine. The crude product was recrystallized from ethanol to form square granulated crystals. Yield: 303.2 g (84.7%). FT-IR (KBr, cm−1): 3304 cm−1 (N–H), 1637 cm−1 (–CO–), 3073 cm−1 (C–H of aromatic ring), 2932, 2855 cm−1 (–CH2–), 1603, 1500 cm−1 (C
C of aromatic ring), 1233 cm−1 (C–N), 848 cm−1 (para substitution of the benzene ring). 1H-NMR [400 MHz, deuterated DMSO-d6/TMS, ppm]: 1.629–1.657 (m, 4H, H1), 1.833–1.878 (m, 4H, H2), 3.878 (s, 2H, H3), 7.266–7.310 (m, 4H, H4), 7.914–7.950 (m, 4H, H5), 8.084–8.100 (d, 2H, H6).
Polymer synthesis
Synthesis of BH-trans-BFCD.
The polymerizations (as shown in Scheme 2) were performed in a 500 mL three-necked round-bottom flask fitted with a mechanical stirrer. Equimolar amounts of trans-BFCD (35.8 g, 0.1 mol) and BHPPE (29 g, 0.1 mol), and NMP (200 ml) were added into the reactor together with toluene (10 ml) as a water carrier and potassium carbonate (16.6 g, 0.12 mol) as a base. The mixture was heated to 160–180 °C to remove the reaction byproduct, water, for about 1–2 h. This is beneficial for the conversion of oligomers. Next, the reaction temperature was kept at 202 °C for 10 h to allow for the formation of high molecular weight polymers. Then, the viscous reaction solution was poured into water to precipitate a fibrous polymer. It was crushed into a powder and washed with hot deionized water repeatedly to remove the KF formed during the polymerization procedure. Finally, it was dried at 100 °C in an oven under vacuum for 12 h.
BH-cis-BFCD was obtained following a similar synthesis procedure as BH-trans-BFCD.
Characterization
Intrinsic viscosity analysis: the intrinsic viscosity (ηint) of polymers was measured by a Cannon-Ubbelohde viscometer at 30 ± 0.1 °C. A polymer solution was prepared by dissolving 0.500 g of polymer into 100 ml of NMP. The results were obtained by a one-point method (or the Solomon–Ciuta equation) as follows:
where ηr = η/η0 and ηsp = η/η0 − 1.
Chemical structure analysis.
FT-IR spectroscopic measurement was performed using a NEXUS670 FT-IR instrument. 1H-NMR spectra were obtained using a Bruker-400 NMR spectrometer. Thermal analysis: differential scanning calorimetry (DSC) measurements were determined using a NETZSCH DSC 200 PC thermal analysis instrument. The heating rate was 10 °C min−1 under a nitrogen atmosphere. Thermogravimetric analysis (TGA) measurements were determined by a TGA Q500 V6.4 Build 193 thermal analysis instrument at different heating rates of 5, 10, 20 and 40 °C min−1 under a nitrogen atmosphere. Mechanical testing: the stress–strain behaviour of the resultant polymers was measured with an Instron Corporation 4302 instrument at room temperature. The specification of the sheet specimens was 50 mm × 3 mm × 3 mm (length × width × thickness) and five replicates were prepared for every sample. An average value of five replicates was used. A polarized light microscope (PLM, BX51) was used to determine the aggregation structure of the samples. A dynamic mechanical analysis (DMA, TA-Q800) was used to investigate the samples’ thermal mechanical properties with a tensile mode at a frequency of 1 Hz and a heating rate of 5 °C min−1. Rheological testing (a disk sample with a diameter of 3 cm and a thickness of 1 mm): a parallel plate rheometer (Bohlin Gemini 200, Britain) fitted with 2.5 cm diameter stainless steel parallel plates was used to characterize the samples’ melt flowability with a scanning model based on temperature (temperature scanning rate: 5 °C min−1, shear strain: 2%, shear frequency: 1 Hz) and time (shear strain 2%, shear frequency 1 Hz). Thermal degradation mechanism: Py/GC-MS spectrometry was carried out to study the degradation mechanism of the resultant semi-aromatic polyamides at different pyrolysis temperatures (550 °C and 650 °C).
Acknowledgements
The authors are grateful to the National Natural Science Foundation of China (Grant No. 21304060) and Outstanding Young Scholars Fund of Sichuan University (Grant No. 2015SCU04A25) for the financial support.
Notes and references
- M. G. José, C. Félix, F. S. García and L. P. de la José, High-performance aromatic polyamides, Prog. Polym. Sci., 2010, 35, 623–638 CrossRef.
- L. Crespo, G. Sanclimens, M. Pons, E. Giralt, M. Royo and F. Albericio, Peptide and Amide Bond-Containing Dendrimers, Chem. Rev., 2005, 105, 1663–1681 CrossRef CAS PubMed.
- G. Zhang, D. T. Bai, D. S. Li, S. R. Long, X. J. Wang and J. Yang, Synthesis and properties of polyamides derived from 4,6-bis(4-chloroformylphenylthio) pyrimidine and 3,6-bis(4-chloroformylphenylthio) pyridazine, Polym. Int., 2013, 62, 1358–1367 CrossRef CAS.
- S. M. Du, W. B. Wang, Y. Yan, J. Zhang, M. Tian, L. Q. Zhang and X. H. Wan, A facile synthetic route to poly(p-phenylene terephthalamide) with dual functional groups, Chem. Commun., 2014, 50, 9929–9931 RSC.
- J. C. Williams, M. A. B. Meador, L. McCorkle, C. Mueller and N. Wilmoth, Synthesis and Properties of Step-Growth Polyamide Aerogels Crosslinked with Triacid Chlorides, Chem. Mater., 2014, 26, 4163–4171 CrossRef CAS.
- S. H. Hsiao, C. W. Chen and G. S. Liou, Novel aromatic polyamides bearing pendent diphenylamino or carbazolyl groups, J. Polym. Sci., Part A: Polym. Chem., 2004, 42, 3302–3313 CrossRef CAS.
- G. S. Liou, H. Y. Lin and H. J. Yen, Synthesis and characterization of electroactive hyperbranched aromatic polyamides based on A2B-type triphenylamine moieties, J. Mater. Chem., 2009, 19, 7666–7673 RSC.
- Y. Rao, A. J. Waddon and R. J. Farris, Structure–property relation in poly(p-phenylene terephthalamide) (PPTA) fibers, Polymer, 2001, 42, 5937–5946 CrossRef CAS.
- J. J. Ferreiro, C. J. G. dela, A. E. Lozano and A. J. de, Polyisophthalamides with heteroaromatic pendent rings: synthesis, physical properties, and water uptake, J. Polym. Sci., Part A: Polym. Chem., 2005, 43, 5300–5311 CrossRef CAS.
- A. J. Uddin, Y. Ohkoshi, Y. Gotoh, M. Nagura and T. Hara, Influence of moisture on the viscoelastic relaxations in long aliphatic chain contained semiaromatic polyamide, (PA9-T) fiber, J. Polym. Sci., Part B: Polym. Phys., 2003, 41, 2878–2891 CrossRef CAS.
- A. J. Uddin, Y. Ohkoshi, Y. Gotoh, M. Nagura and T. Hara, Melt spinning and laser-heated drawing of a new semiaromatic polyamide, PA9-T fiber, J. Polym. Sci., Part B: Polym. Phys., 2004, 42, 433–444 CrossRef CAS.
- A. J. Uddin, Y. Gotoh, Y. Ohkoshi, M. Nagura, R. Endo and T. Hara, Hydration in a new semiaromatic polyamide observed by humidity-controlled dynamic viscoelastometry and X-ray diffraction, J. Polym. Sci., Part B: Polym. Phys., 2005, 43, 1640–1648 CrossRef CAS.
- A. J. Uddin, Y. Ohkoshi, Y. Gotoh, M. Nagura, R. Endo and T. Hara, Effects of take-up speed of melt spinning on the structure and mechanical properties of maximally laser drawn PA9-T fibers, Int. Polym. Process., 2006, 21, 263–271 CrossRef CAS.
- A. J. Uddin, Y. Gotoh, Y. Ohkoshi, T. Nishino and R. Endo, Crystal Modulus of a New Semiaromatic Polyamide 9-T, Polym. Eng. Sci., 2012, 52, 331–337 CAS.
- C. H. Zhang, X. B. Huang, X. B. Zeng, M. Cao, T. M. Cai, S. J. Jiang and Q. F. Yi, Fluidity Improvement of Semiaromatic Polyamides: Modification with Oligomers, J. Appl. Polym. Sci., 2014, 131(7) DOI:10.1002/APP.40058.
- W. Z. Wang and Y. H. Zhang, Synthesis of semiaromatic polyamides based on decanediamine, Chin. J. Polym. Sci., 2010, 4, 467–473 CrossRef.
- A. Ballistreri, D. Garozzo, M. Giuffrida and P. Maravigna, Thermal decomposition processes in aliphatic-aromatic polyamides investigated by mass spectrometry, Macromolecules, 1986, 19, 2693–2699 CrossRef CAS.
- M. Shabanian, N. J. Kang, J. W. Liu, U. Wagenknecht, G. Heinrichbd and D. Y. Wang, Bio-based semi-aromatic polyamide/functional clay nanocomposites: preparation and properties, RSC Adv., 2014, 4, 23420–23427 RSC.
- G. Zhang, G. S. Huang, D. S. Li, X. J. Wang, S. R. Long and J. Yang, Facile Synthesis of Processable Semiaromatic Polyamides Containing Thioether Units, Ind. Eng. Chem. Res., 2011, 50, 7056–7064 CrossRef CAS.
- C. H. R. M. Wilsens, Y. S. Deshmukh, B. A. J. Noordover and S. Rastogi, Influence of the 2,5-Furandicarboxamide Moiety on Hydrogen Bonding in Aliphatic-Aromatic Poly(ester amide)s, Macromolecules, 2014, 47, 6196–6206 CrossRef CAS.
- H. Harashina, T. Nakane and T. Itoh, Synthesis of Poly(ester amide)s by the Melt Polycondensation of Semiaromatic Polyesters with Ethanolamine and Their Characterization, J. Polym. Sci., Part A: Polym. Chem., 2007, 11, 2184–2193 CrossRef.
- S. H. Yang, P. Fu, M. Y. Liu, Y. D. Wang, Y. C. Zhang and Q. X. Zhao, Synthesis, characterization of polytridecamethylene 2,6-naphthalamide as semiaromatic polyamide containing naphthalene-ring, Express Polym. Lett., 2010, 4, 442–449 CrossRef CAS.
- W. Z. Wang, X. W. Wang, R. X. Li, B. Y. Liu, E. G. Wang and Y. H. Zhang, Environment-Friendly Synthesis of Long Chain Semiaromatic Polyamides with High Heat Resistance, J. Appl. Polym. Sci., 2009, 114, 2036–2042 CrossRef CAS.
- T. F. Novitsky, C. A. Lange, L. J. Mathias, S. Osborn, R. Ayotte and S. Manning, Eutectic melting behavior of polyamide 10,T-co-6,T and 12,T-co-6,T copolyterephthalamides, Polymer, 2010, 51, 2417–2425 CrossRef CAS.
- M. Y. Liu, K. F. Li, S. H. Yang, P. Fu, Y. D. Wang and Q. X. Zhao, Synthesis and Thermal Decomposition of Poly(dodecamethylene terephthalamide), J. Appl. Polym. Sci., 2011, 122, 3369–3376 CrossRef CAS.
- W. Z. Wang and Y. H. Zhang, Environment-friendly synthesis of long chain semiaromatic polyamides, Express Polym. Lett., 2009, 8, 470–476 CrossRef.
- D. J. Liaw, F. C. Chang, M. K. Leung, M. Y. Chou and K. Muellen, High thermal stability and rigid rod of novel organosoluble polyimides and polyamides based on bulky and noncoplanar naphthalene-biphenyldiamine, Macromolecules, 2005, 38, 4024–4029 CrossRef CAS.
- S. Moisa, G. Landsberg, D. Rittel and J. L. Halary, Hysteretic thermal behavior of amorphous semi-aromatic polyamides, Polymer, 2005, 46, 11870–11875 CrossRef CAS.
- S. Shabbir, S. Zulfiqar and M. M. I. Sarwar, Amine-terminated aromatic and semi-aromatic hyperbranched polyamides: synthesis and characterization, J. Polym. Res., 2011, 18, 1919–1929 CrossRef CAS.
- S. Shabbir, S. Zulfiqar, A. Zahoor and M. M. I. Sarwar, Pyrimidine based carboxylic acid terminated aromatic and semiaromatic hyperbranched polyamide-esters: synthesis and characterization, Tetrahedron, 2010, 66, 7204–7212 CrossRef CAS.
- D. J. Liaw, P. N. Hsu, W. H. Chen and S. L. Lin, Synthesis and Properties of New Soluble Polyamides Derived from 2,2′-Dimethyl-4,4′-bis(4-carboxyphenoxy) biphenyl, Macromolecules, 2002, 35, 4669–4676 CrossRef CAS.
- G. Zhang, Y. X. Zhou, Y. Kong, Z. M. Li, S. R. Long and J. Yang, Semiaromatic polyamides containing ether and different numbers of methylene (2–10) units: synthesis and properties, RSC Adv., 2014, 4, 63006–63015 RSC.
- G. Zhang, Y. X. Zhou, Y. Li, X. J. Wang, S. R. Long and J. Yang, Investigation of the synthesis and properties of isophorone and ether units based semi-aromatic Polyamides, RSC Adv., 2015, 5, 49958–49967 RSC.
- O. Ivanysenko, S. Strandman and X. X. Zhu, Triazole-linked
polyamides and polyesters derived from cholic acid, Polym. Chem., 2012, 3, 1962–1965 RSC.
- M. Shabanian, N. J. Kang, D. Y. Wang, U. Wagenknecht and G. Heinrich, Synthesis, characterization and properties of novel aliphatic-aromatic polyamide/functional carbon nanotube nanocomposites via in situ polymerization, RSC Adv., 2013, 3, 20738–20745 RSC.
- H. Y. Huang, Y. T. Lee, L. C. Yeh, J. W. Jian, T. C. Huang, H. T. Liang, J. M. Yeh and Y. C. Chou, Photoactively electroactive polyamide with azo group in the main chain via oxidative coupling polymerization, Polym. Chem., 2013, 4, 343–350 RSC.
- D. Matsunaga, T. Tamaki and K. Ichimura, Azo-pendant polyamides which have the potential to photoalign chromonic lyotropic liquid crystals, J. Mater. Chem., 2003, 13, 1558–1564 RSC.
- H. E. Kissinger, Reaction Kinetics in Differential Thermal Analysis, Anal. Chem., 1957, 29, 1702–1706 CrossRef CAS.
- G. Zhang, X. J. Xing, D. S. Li, X. J. Wang and J. Yang, Effects of thioether content on the solubility and thermal properties of aromatic polyesters, Ind. Eng. Chem. Res., 2013, 52, 16577–16584 CrossRef CAS.
Footnote |
† Electronic supplementary information (ESI) available. See DOI: 10.1039/c5py01634g |
|
This journal is © The Royal Society of Chemistry 2016 |