DOI:
10.1039/C5NJ02794B
(Paper)
New J. Chem., 2016,
40, 832-837
Room temperature synthesis of organic surfactant-free PbS and PbSe nanoparticles exhibiting NIR absorption†
Received
(in Montpellier, France)
10th October 2015
, Accepted 13th November 2015
First published on 20th November 2015
Abstract
Chemically synthesized nanoparticles have organic molecules surrounding the particles as capping, which creates an inorganic–organic interface. These intimate interfaces influence the properties of the applied materials and subsequently the applications. Therefore, a method to produce surfactant-free PbS and PbSe nanoparticles at room temperature within 15 min of reaction time was developed. The materials, their morphologies, and optical properties were characterized by means of X-ray diffraction, electron microscopy, and UV-Vis-NIR spectroscopy. These particles were monodispersed with almost uniform size and shape with an average diameter of 2.07 ± 0.4 nm for PbS and 2.98 ± 0.6 nm for PbSe. UV-Vis-NIR spectra of these materials showed absorption spanning the NIR region.
1. Introduction
In the last few years, the synthesis of semiconducting nanomaterials has attracted great research interest due to their extensive application in emerging devices.1–8 Semiconducting nanomaterials such as CdS/Se, ZnS/Se, and PbS/Se are useful materials in technology because of their tuneable optical properties.9–11 Materials with a low band gap and a large Bohr radius show a strong confinement of the electron–hole pairs and larger optical nonlinearity.12–14 Among many applied materials, PbS and PbSe are important IV–VI group semiconductors that have a narrow band gap (PbS = 0.41 eV, PbSe = 0.28 eV) with an effective Bohr radius of 18 nm and 46 nm respectively.15,16 The band gaps can be tuned for absorption across the visible to near infrared (NIR) regions (700–1600 nm).17
Materials exhibiting NIR absorption and emission are very useful in various applications like NIR communications, in vivo biomedical applications (imaging and labelling), infrared detectors and electroluminescent devices.18 For example, Mn doped PbS nanoparticles are used as ferromagnetic materials, PbS/Se nanoparticles are useful in high-performance field-effect transistors (FETs), thermoelectric (TE) devices, etc.17a,19,20 Furthermore, both PbS and PbSe show multiple exciton generation for a single photon, which increases the photo conversion efficiency in a quantum dot based solar cell by up to 66%.21,22
The optical, electronic, catalytic, biological, mechanical and magnetic properties of nanoparticles are different from their bulk.2,5,19,23–29 Colloidal synthesis of nanoparticles offers variety in sizes, shapes, and dimensions of the particles of applied materials.2,5,19,23–29 Various methods are available for the synthesis of PbS and PbSe including sono electrochemical, gas phase, solution phase, template-assisted syntheses, hot injection methods, pyrolysis of single source precursors and polymer film routes.30 Most of the methods are complex, require high temperatures and need templates. Templates and surfactants in reactions introduce complexity and impurities in the products, which affect the properties of the materials.
Generally, chemically synthesized nanoparticles have organic molecules surrounding the particles as capping, which creates an inorganic–organic interface. These intimate interfaces influence the properties of the materials and subsequently the applications. For example, the organic molecules around the nanoparticles can be a hindrance to the electron mobility in electronic and optical devices.31 Therefore, to ensure compatibility, it is crucial to design a method of producing applied materials without organic capping molecules surrounding. Herein, we report a simple room temperature, short duration (less than 15 min) synthetic method for PbS and PbSe nanoparticles without any capping agent.
Intimate organic–inorganic interfaces between the nanoparticles and capping molecules, and the size and shape of particles collectively impart the properties of nanoparticles. The availability of organic surfactant molecules in the reaction medium, reaction length and temperature, would control the above parameters. Therefore, we have been developing methods and advocating for organic capping agent-free nanoparticles.32 Consequently, we have developed a simple typical room temperature synthetic method for the production of both PbS and PbSe.
2. Experimental section
2.1. Materials
All the chemicals used in the synthesis were purchased from Sigma Aldrich. Lead nitrate {Pb(NO3)2·3H2O}, lithium borohydride (LiBH4), sulphur (S) powder and selenium (Se) powder are used as reagents without any further purification. Standard air-free conditions were maintained throughout the reaction.
2.2. Synthesis of PbS nanoparticles
In a typical procedure, 39 mg of sulphur (1.21 mmol) was dispersed into 10 ml of dry tetrahydrofuran (THF) in a 50 ml two neck RB flask and then LiBH4 (53 mg, 2.42 mmol) and lead nitrate (400 mg, 1.21 mmol) were added. The reaction mixture was stirred at room temperature under a nitrogen atmosphere for 15 min. Initially the colour of the solution was yellow, but after completion of the reaction it turned black. Volatile side products and solvents were removed by high vacuum. Then the crude product was washed by methanol and THF to remove excess of Pb(NO3)2·3H2O, sulphur, lithium salt and other side products. The washing was carried out as follows. Methanol was added to the crude reaction mixture, sonicated for 3 min and then centrifuged. The centrifugate was discarded and fresh methanol was added to the residue. This process was repeated three times with methanol and two times with THF, finally the residue was dried for 3 h under vacuum to obtain pure black PbS nanoparticles.
2.3. Synthesis of PbSe nanoparticles
In a 50 ml two neck RB flask, 95 mg selenium powder (1.21 mmol) was added to 10 ml of dry THF and then LiBH4 (53 mg, 2.42 mmol) and lead nitrate (400 mg, 1.21 mmol) were added. The reaction mixture was stirred under a nitrogen atmosphere for 15 min at room temperature. After completion of the reaction, it turned black. Volatile side products and solvents were removed under a high vacuum. Then the crude product was purified similar to the above procedure to obtain black PbSe nanoparticles.
2.4. Instruments and sample preparation
Powder X-ray diffraction (PXRD) patterns were obtained using a Bruker D8 X-ray diffractometer [λ (Cu-Kα) = 1.54 Å] at a scan rate of 1° min−1. Field Emission Scanning Electron Microscopy (FESEM) images were recorded using an Ultra 55 Carl Zeiss instrument with an operating voltage of 10 kV. Samples for the FESEM analysis were mounted on a stub using conductive carbon tape. For this purpose the samples were dispersed in methanol and kept on a glass plate. Transmission electron microscopy (TEM) images were recorded using a FEI Technai G2 20 STEM instrument operated at an acceleration voltage of 200 kV. The TEM samples were prepared by dispersing in methanol followed by sonication for 3 min. Then the sonicated solution was dispersed on carbon coated copper grids (200 meshes). The UV-Visible-NIR spectra of samples dispersed in toluene were recorded using a Shimadzu UV-3600/Vis spectrometer. The Fourier transform infrared (FT-IR) spectra (KBr pellet) were recorded using a Jasco 5300 spectrophotometer.
3. Results and discussion
3.1. Synthesis and characterization
In the present procedure, Pb(NO3)2·3H2O was reduced by LiBH4 in the presence of sulphur (S), or selenium (Se) powder at room temperature in THF to yield PbS or PbSe respectively. It is worth mentioning here that Xianming Liu33 reported PbSe nanowire synthesis, in a sealed tube, which was possible at 10 °C in 4 h. However, we obtained the materials under the best possible ambient conditions in short duration reactions. Along with the formation of a product, we also observed the evolution of BH3 gas,34 which is a useful reagent in the organic synthesis. BH3 was characterized by trapping with triphenyl phosphine followed by the analysis of multinuclear spectral data of the borane-phosphine adduct.
The PXRD (Fig. 1a and b) pattern of as prepared PbS and PbSe showed that both the products were well crystallised. All peaks could be indexed as the FCC phase of PbS (JCPDS # 65-0692, a = 5.94) and the FCC phase of PbSe (JCPDS # 65-2941, a = 6.134) respectively. There were no other significant peaks in spectra corresponding to S, Se or Pb(NO3)2, confirming the purity of the products. EDS analyses also showed only Pb, S, Se and there were no other impurities (Fig. 2a and b). FESEM images showed aggregates of PbS spherical nanoparticles (Fig. 3a and b) and PbSe spherical nanoparticles (Fig. 3c and d). Both the particle sizes varied between 10 and 40 nm.
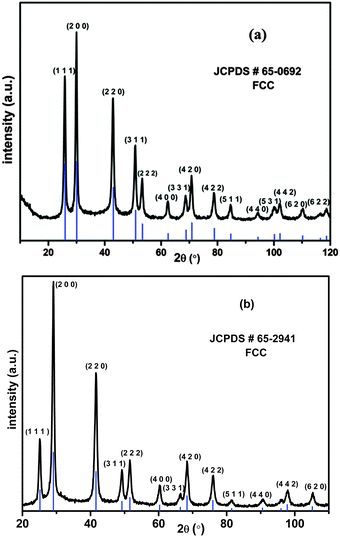 |
| Fig. 1 PXRD spectrum of (a) PbS and (b) PbSe both showing a face centred cubic lattice structure. | |
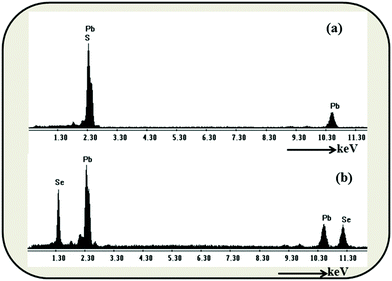 |
| Fig. 2 EDS spectra of (a) PbS and (b) PbSe. | |
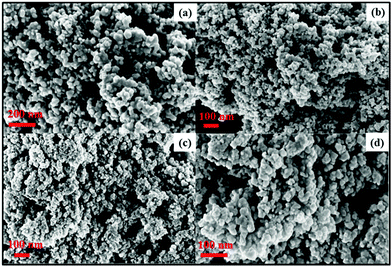 |
| Fig. 3 FESEM images of (a and b) PbS, (c and d) PbSe showing aggregation of spherical nanoparticles. | |
Transmission electron microscopy (TEM) analyses of PbS and PbSe nanoparticles were carried out to observe the shape and size of the as-prepared nanoparticles. The shape of the PbS nanoparticles was near spherical in all cases (Fig. 4a and 5a) showing monodispersed particles with an average diameter of 2.07 ± 0.4 nm for PbS and 2.98 ± 0.6 nm for PbSe. Selected area electron diffraction (SAED) of PbS nanoparticles (Fig. 4c) showed diffraction rings identified as planes of (1 1 1), (2 0 0), (2 2 0), (2 2 2), (4 0 0), (3 1 1), (4 2 2), (5 1 1), (4 4 0), (6 2 0), (4 4 4). These planes matched well with the PXRD pattern of the cubic phase of PbS (JCPDS # 65-0692). Similarly, SAED of PbSe nanoparticles (Fig. 5c) diffraction rings were identified as planes of (1 1 1), (2 0 0), (2 2 0), (2 2 2), and (4 2 0). These planes matched well with the PXRD pattern of the cubic phase of PbSe (JCPDS #65-2941). HRTEM images of PbS nanoparticles showed, d = 2.97 Å conforming the plane (2 0 0), (JCPDS #65-0692), while that of PbSe nanoparticles showed, d = 3.54 Å conforming the plane (1 1 1), (JCPDS #65-2941).
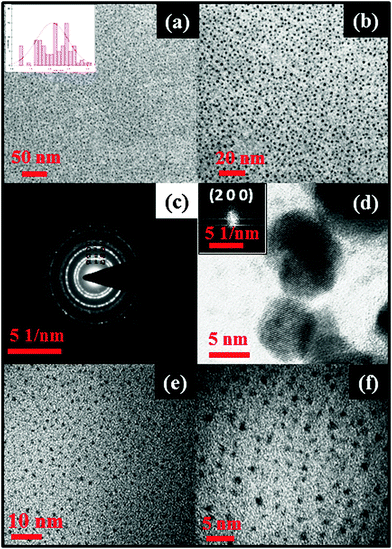 |
| Fig. 4 TEM images of PbS nanoparticles, (a and b) particles of spherical shape (inset: particle size distribution). (c) SAED pattern. (d) HRTEM images of PbS nanoparticles (inset: FFT pattern). (e and f) After eight months of synthesis. | |
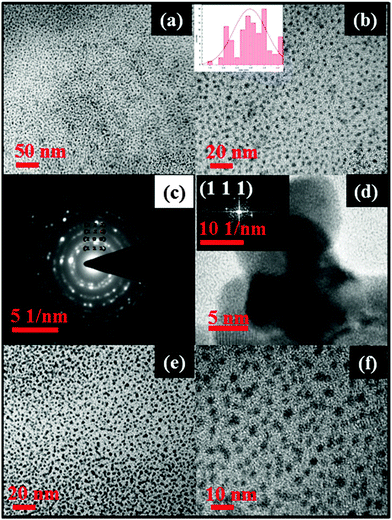 |
| Fig. 5 TEM images of PbSe nanoparticles, (a and b) particles of spherical shape (inset: particle size distribution). (c) SAED pattern. (d) HRTEM images of PbS nanoparticles (inset: FFT pattern), (e and f) after eight months of synthesis. | |
3.2. Organic surfactant-free lead chalcogenides
The purity of the products was primarily confirmed by clean PXRD patterns. EDS analyses of PbS and PbSe showed only Pb, S, and Se and there were no peak(s) corresponding to any carbonaceous impurities. FT-IR spectra of the as prepared PbS (Fig. 6) and PbSe (Fig. S8, ESI†) nanoparticles showed no signal for any organic moieties confirming the absence of any organic molecules covering the particles. Thus, our reactions yielded nanoparticles without any surfactants around. Generally, capping agents are needed to ensure the colloidal stability during the synthesis of nanoparticles. We have been observing that metal sulphide nanoparticles do not readily agglomerate32 whereas it is a common phenomenon in the case of metal nanoparticles. Nevertheless, the formation of nanoparticles without any surfactant molecules in the reaction medium definitely needs an explanation.
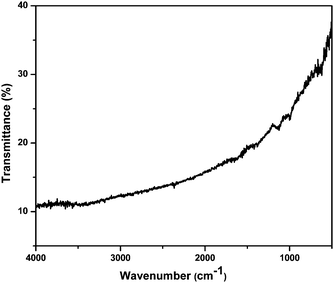 |
| Fig. 6 FT-IR spectrum of PbS nanoparticles showing the absence of organic species. | |
Metal nanoparticles normally agglomerate because of the possibility of metallic bonding. However, metal sulphides, sulphates and other salts need few driving forces to grow into big crystals.35 Hence, the digestion process is practiced well enough to encourage the growth of crystals. Xie and co-workers explained the formation of nanoparticles without a capping agent invoking the right growing conditions as a favouring factor for the formation of thermodynamically stable structure.36 As explained by the theory of relative super saturation,37 particles grow only under favourable conditions. Since, our reaction time was very short, it was a room temperature reaction and the products were not soluble, the conditions were not supporting the growth of the particles. This means, the reaction parameters were not encouraging the growth of the nuclei.
The reaction conditions reported here are the optimized conditions. We have conducted the reactions in toluene and ether as well. Interestingly, these reactions did not yield any clean product indicating the importance of the solvent selection. It is likely that the solubility of sulphur (selenium was not soluble in THF) and LiBH4 in THF played a major role. It is to be noted that the metal source, PbNO3, was partially soluble in THF. In our procedure, elemental sulphur or selenium was added first, and then LiBH4 was added. LIBH4 reduced the chalcogens and immediate formation of a black suspension in THF was observed, which indicated the formation of S2− or Se2−. Subsequently after addition of Pb(NO3)2·3H2O, black PbS or PbSe was obtained. Thus, the partial solubility of the metal source created a supersaturated condition, which drove the reaction to yield the nanoparticles. However, the prolonged reaction time (3 h) yielded products with particles of bigger size (Fig. S5, ESI†).
To understand further the stability, we have recorded the PXRD pattern (Fig. S1 and S2, ESI†) and TEM images (Fig. 4e, f and 5e, f) of eight month old samples of PbS and PbSe stored in a screw capped vial. The spectrum showed no change in the diffraction patterns indicating the stability of the compounds. The TEM images of the organic surfactant-free particles showed only a subtle change in the sizes (average diameter of 2.51 ± 0.6 nm for PbS and 3.26 ± 0.4). Therefore, from this study and our earlier work33 it is very clear that the surfactants are needed only during the synthesis of metal sulphide nanoparticles. Since there was no other post synthesis factor (such as Ostwald ripening) supporting the growth of the particles, these PbS and PbSe nanoparticles remained in nano sizes.
3.3. NIR absorption of lead chalcogenides
Materials with NIR absorption have potential use in many areas, case in point, solar cells and electronic communications.38 Absorption spectra of PbS with an excitonic absorption peak spanning from 800 to 1800 nm were reported.39 Similarly, absorption spectra of PbSe with a peak spanning from 900 to 2500 nm were reported.40 These lead chalcogenides are known to exhibit a blue shift in NIR absorption with decreasing size.41 Therefore, we have studied the absorption properties of the PbS and PbSe nanoparticles obtained in our reactions. These nanoparticles were dispersed in toluene and UV-Vis-NIR spectra were recorded. Interestingly, both the nanoparticles had absorption bands spanning into the IR region. PbS showed a strong absorption at 802 nm (Fig. 7a), while PbSe showed a strong absorption at 1424 nm (Fig. 7b). Significantly, these organic-free chalcogenide (PbS and PbSe) nanoparticles did not show emission spectra. Scanning the literature42 showed that the emission was diminishing when there was no surfactants. It is to be noted that our observation is in concurrence with the literature. However, since these materials were free from organic substances, they will have potential use in electronic devices. It is worth mentioning here that it was possible to produce these applied materials using a standardized synthetic methodology within a short reaction time.
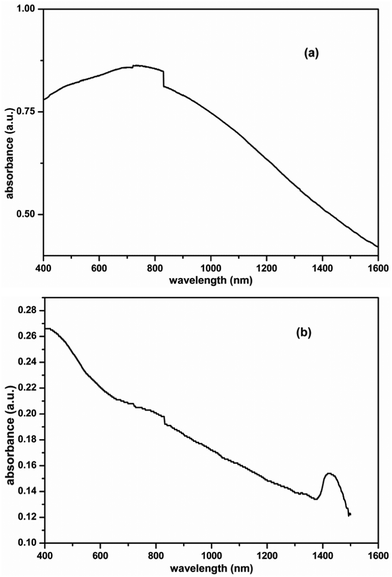 |
| Fig. 7 UV-Vis-NIR spectrum of as prepared (a) PbS and (b) PbSe nanoparticles showing absorption peak in the NIR region. | |
4. Conclusions
In summary, we have developed a simple, short duration room temperature synthetic methodology for the production of organic capping-free PbS and PbSe nanoparticles. This method requires no complex apparatus, reagents, or techniques, and the reactions afforded PbS/Se nanoparticles in high yields. The synthesized particles were thoroughly characterized by different techniques; PXRD, FESEM and TEM while their purity was revealed from FT-IR spectra. These particles were monodispersed with almost uniform size and shape with an average diameter of 2.07 ± 0.4 nm for PbS and 2.98 ± 0.6 nm for PbSe. UV-Vis-NIR spectra of these materials showed absorption spanning the NIR region.
Acknowledgements
The authors thank the Centre for Nanotechnology at the University of Hyderabad for the TEM facility. SNG acknowledges UGC-India for a doctoral fellowship. The work is supported by DST-SERB of India under project No: SB/S1/IC-47/2013.
Notes and references
- A. K. Dutta, T. Ho, L. Zhang and P. Stroeve, Chem. Mater., 2000, 12, 1042 CrossRef CAS.
- L. Brus, Appl. Phys. A: Mater. Sci. Process., 1991, 53, 465 CrossRef.
- A. P. Alivisatos, Science, 1996, 271, 933 CAS.
- W. C. W. Chan and S. Nie, Science, 1998, 281, 2016 CrossRef CAS PubMed.
- J. Hu, T. W. Odom and C. M. Lieber, Acc. Chem. Res., 1999, 32, 435 CrossRef CAS.
- X. Peng, L. Manna, W. Yang, J. Wickham, E. Scher, A. Kadavanich and A. P. Alivisatos, Nature, 2000, 404, 59 CrossRef CAS PubMed.
- X. Duan, Y. Huang, Y. Cui, J. Wang and C. M. Lieber, Nature, 2001, 409, 66 CrossRef CAS PubMed.
- K. Singh, A. A. McLachlan and D. G. Marangoni, Colloids Surf., A, 2009, 345, 82 CrossRef CAS.
-
(a) B. Z. Liang, K. L. Dzienis, J. Xu and Q. Wang, Adv. Funct. Mater., 2006, 16, 542 CrossRef;
(b) T. Rath and G. Trimmel, Hybrid Mater., 2013, 1, 15–36 Search PubMed.
-
(a) C.-H. Lai, M.-Y. Lu and L.-J. Chen, J. Mater. Chem., 2012, 22, 19 RSC;
(b) J. Jiea, W. Zhang, I. Bello, C.-S. Lee and S.-T. Lee, Nano Today, 2010, 5, 313 CrossRef.
-
(a) W. Ma, S. L. Swisher, T. Ewers, J. Engel, V. E. Ferry, H. Atwater and A. P. Alivisatos, ACS Nano, 2011, 10, 8140 CrossRef PubMed;
(b) H. Fu and S.-W. Tsang, Nanoscale, 2012, 4, 2187 RSC.
- M. Chen, Y. Xie, J. C. Lu, Y. J. Zhu and Y. T Qian, J. Mater. Chem., 2001, 11, 518–520 RSC.
- Y. Wang and N. Herron, J. Phys. Chem., 1991, 95, 525 CrossRef CAS.
- Y. Wang, Acc. Chem. Res., 1991, 24, 133 CrossRef CAS.
- F. W. Wise, Acc. Chem. Res., 2000, 33, 773 CrossRef CAS PubMed.
- E. Lifshitz, M. Bashouti, V. Kloper, A. Kigel, M. S. Eisen and S. Berger, Nano Lett., 2003, 3, 857 CrossRef CAS.
-
(a) B. L. Bakueva, I. Gorelikov, S. Musikhin, X. S. Zhao, E. H. Sargent and E. Kumacheva, Adv. Mater., 2000, 16, 926 CrossRef;
(b) R. D. Schaller, M. A. Petruska and V. I. Klimov, J. Phys. Chem. B, 2003, 107, 13765 CrossRef CAS;
(c) I. Moreels, B. Fritzinger, J. C. Martins and Z. Hens, J. Am. Chem. Soc., 2008, 130, 15081 CrossRef CAS PubMed.
-
(a) Y. T. Lim, S. Kim, A. Nakayama, N. E. Stott, M. G. Bawendi and J. V Frangioni, Mol. Imaging, 2003, 2, 50 CrossRef CAS PubMed;
(b)
R. Richards-Kortum, R. Drezek, K. Sokolov, I. Pavlova and M. Follen, in Handbook of Biomedical Fluorescence, ed. M.-A Mycek and B. W. Pogue, Marcel Dekker, Inc., New York, 2003, 237 Search PubMed;
(c) R. Weissleder, Nat. Biotechnol., 2001, 19, 316 CrossRef CAS PubMed.
- S. K. Mandal, A. R. Mandal and S. Banerjee, ACS Appl. Mater. Interfaces, 2012, 4, 205 CAS.
- D. V. Talapin and C. B. Murray, Science, 2005, 310, 86 CrossRef CAS PubMed.
- M. A. Olshavasky, A. N. Goldstein and A. P. Alivisatos, J. Am. Chem. Soc., 1990, 112, 9438 CrossRef.
-
(a) K. Anusorn, T. Kevin, T. Kensuke, K. Masaru and V. K. Prashant, J. Am. Chem. Soc., 2008, 130, 4007 CrossRef PubMed;
(b) G. Hodes, Isr. J. Chem., 1993, 33, 95 CrossRef CAS;
(c) G. Sasha and H. Gary, J. Phys. Chem., 1994, 98, 5338 CrossRef;
(d) A. J. Nozik, Phys. E, 2002, 14, 115 CrossRef CAS;
(e) Z. L. Wang, J. Phys. Chem., 2000, 104, 1153 CrossRef CAS;
(f) K. Sandeep and D. S. Gregory, Microchim. Acta, 2008, 160, 315 CrossRef.
-
(a) C. Dekker, Phys. Today, 1999, 52, 22 CrossRef CAS;
(b) T. Dietl, H. Ohno, F. Matsukura, J. Cibert and D. Ferrand, Science, 2000, 287, 1019 CrossRef CAS PubMed;
(c) J. König, H. H. Lin and A. H. MacDonald, Phys. Rev. Lett., 2000, 84, 5628–5631 CrossRef PubMed;
(d) J. M. D. Coey, M. Venkatesan and C. B. Fitzgerald, Nat. Mater., 2005, 4, 173 CrossRef CAS PubMed;
(e) S. Banerjee, M. Mandal, N. Gayathri and M. Sardar, Appl. Phys. Lett., 2007, 91, 182501 CrossRef.
- S. Y. Chang, L. Liu and S. A. Asher, J. Am. Chem. Soc., 1994, 116, 6745 CrossRef CAS.
- F. Caruso, R. A. Caruso and H. Mohwald, Science, 1998, 282, 1111 CrossRef CAS PubMed.
- D. E. Bergbreiter, Angew. Chem., Int. Ed., 1999, 38, 2870 CrossRef CAS.
- H. Huang, E. E. Remsen, T. Kowalewski and K. L. Wooley, J. Am. Chem. Soc., 1999, 121, 3805 CrossRef CAS.
- Y. Sun and Y. N. Xia, Science, 2002, 298, 2176 CrossRef CAS PubMed.
- Y. D. Yin, R. M. Rioux, C. K. Erdonmez, S. Hughes, G. A. Somorjai and A. P. Alivisatos, Science, 2004, 304, 711 CrossRef CAS PubMed.
-
(a) T. He, D. R. Chen, X. L. Jiao and Y. L. Wang, Adv. Mater., 2006, 18, 1078 CrossRef CAS;
(b) X. Zhao, V. Sukhovatkin, I. Gorelikov, S. Musikhin, E. H. Sargent, S. Cauchi and E. Kumacheva, Langmuir, 2005, 21, 1086 CrossRef CAS PubMed;
(c) Y. D. Yin, C. Erdonmez, S. Aloni and A. P. Alivisatos, J. Am. Chem. Soc., 2006, 128, 12671 CrossRef CAS PubMed;
(d) A. Dong, F. Wang, T. L. Daulton and W. E. Buhro, Nano Lett., 2007, 7, 1308 CrossRef CAS PubMed;
(e) S. Gorer, A. Albu-Yaron and G. Hodes, Chem. Mater., 1995, 7, 1243 CrossRef CAS;
(f) S. F. Wang, F. Gu and M. K. Lü, Langmuir, 2006, 22, 398 CrossRef CAS PubMed;
(g) M. Gao, Y. Yang, B. Yang, F. Bian and J. Shen, J. Chem. Soc., Chem. Commun., 1994, 2779 RSC;
(h) C. B. Murray, S. Sun, W. Gaschler, H. Doyle, T. A. Betley and C. R. Kagan, IBM J. Res. Dev., 2001, 45, 47 CrossRef CAS.
- M. V. Kovalenko, M. Scheele and D. V. Talapin, Science, 2009, 324, 1417 CrossRef CAS PubMed.
-
(a) B. G. Kumar and K. Muralidharan, J. Mater. Chem., 2011, 30, 11271 RSC;
(b) B. G. Kumar and K. Muralidharan, Eur. J. Inorg. Chem., 2013, 2102 CrossRef CAS;
(c) B. G. Kumar and K. Muralidharan, RSC Adv., 2014, 4, 28219 RSC.
- B. W. Wang, Y. Geng, Y. Qian, M. Ji and X. Liu, Adv. Mater., 1998, 10, 1479 CrossRef.
- S. R. Ghanta, M. H. Rao and K. Muralidharan, Dalton Trans., 2013, 42, 8420 RSC.
-
(a) W. Z. Ostwald, Phys. Chem., 1900, 34, 495 Search PubMed;
(b) B. Li, G. Rong, Y. Xie, L. Huang and C. Feng, Inorg. Chem., 2006, 45, 6404 CrossRef CAS PubMed;
(c) J. Li and H. C. Zeng, J. Am. Chem. Soc., 2007, 129, 15839 CrossRef CAS PubMed.
- S. Zhang, C. Wu, Z. Wu, K. Yu, J. Wei and Y. Xie, Cryst. Growth Des., 2008, 8, 2933 CAS.
-
(a) P. P. von Weimarn, Chem. Rev., 1925, 2, 217 CrossRef;
(b) A. E. Nielsen, Acta Chem. Scand., 1960, 14, 1654 CrossRef CAS;
(c) R. A. Johnson and J. D. O’Rourke, J. Am. Chem. Soc., 1954, 76, 2124 CrossRef CAS;
(d)
R. A. D. Jr and A. L. Underwood, Quantitative Analysis, PHI, 6th edn, 2013, p. 74 Search PubMed;
(e)
D. A. Skoog, D. M. West, F. J. Holler and S. R. Crouch, Fundamentals of Analytical Chemistry, CENGAGE Learning, 8th edn, 2004, p. 316 Search PubMed.
-
(a) A. G. Pattantyus-Abraham, I. J. Kramer, A. R. Barkhouse, X. Wang, G. Konstantatos, R. Debnath, L. Levina, I. Raabe, M. K. Nazeeruddin, M. Grätzel and E. H. Sargent, ACS Nano, 2010, 4, 3374 CrossRef CAS PubMed;
(b) S. A. McDonald, G. Konstantatos, S. Zhang, P. W. Cyr, E. J. D. Klem, L. Levina and E. H. Sargent, Nat. Mater., 2005, 4, 13 CrossRef PubMed.
-
(a) L. Cademartiri, J. Bertolotti, R. Sapienza, D. Wiersma, G. von Freymann and G. A. Ozin, J. Phys. Chem. B, 2006, 110, 671 CrossRef CAS PubMed;
(b) J. Joo, H. B. Na, T. Yu, J. H. Yu, Y. W. Kim, F. X. Wu, J. Z. Zhang and T. Hyeon, J. Am. Chem. Soc., 2003, 125, 11100 CrossRef CAS PubMed.
-
(a) C. M. Evans, L. Guo, J. J. Peterson, S. Maccagnano-Zacher and T. D. Krauss, Nano Lett., 2008, 8, 2896 CrossRef CAS PubMed;
(b) W. W. Yu, J. C. Falkner, B. S. Shih and V. L. Colvin, Chem. Mater., 2004, 16, 3318 CrossRef CAS.
- M. A. Hines and G. D. Scholes, Adv. Mater., 2003, 15, 1844 CrossRef CAS.
-
(a) A. Swarnkar, G. S. Shanker and A. Nag, Chem. Commun., 2014, 50, 4743–4746 RSC;
(b) K. P. Kadlag, M. J. Rao and A. Nag, J. Phys. Chem. Lett., 2013, 4, 1676–1681 CrossRef CAS PubMed;
(c) X. Chen, Y. Lou, A. C. Samia and C. Burda, Nano Lett., 2003, 3, 799–803 CrossRef CAS;
(d) A. MK. Smith and S. Nie, Acc. Chem. Res., 2010, 43, 190–200 CrossRef PubMed.
Footnote |
† Electronic supplementary information (ESI) available. See DOI: 10.1039/c5nj02794b |
|
This journal is © The Royal Society of Chemistry and the Centre National de la Recherche Scientifique 2016 |