DOI:
10.1039/C5NJ02250A
(Letter)
New J. Chem., 2016,
40, 54-57
An unprecedented gas-phase condensation reaction mediated by a bissilylated chloronium ion intermediate†
Received
(in Montpellier, France)
24th August 2015
, Accepted 16th October 2015
First published on 23rd October 2015
Abstract
Gas-phase Cl2Si(OH)+ and Si2OCl4−n(OH)n+1+ (n = 0–4) ions are shown to undergo a novel condensation reaction with SiCl4 that provides a potentially useful pathway for a variety of networks containing the –(Si–O–Si)– backbone. Theoretical calculations reveal that these reactions proceed via a bissilylated chloronium ion intermediate.
Silicon alkoxides, Si(OR)4, and related alkoxysilanes have been extensively explored as the molecular precursors of a large variety of silicon-containing glasses, gels, ceramics and nanomaterials via sol–gel chemistry.1,2 For these substrates, sol–gel processes are well known to be initiated by the hydrolysis of the alkoxy groups followed by successive condensation reactions leading to the formation of
Si–O–Si
networks. While the kinetics and thermodynamics of the hydrolysis processes have been characterized,3,4 a full understanding of the initial processes is essential for the purpose of designing materials with well-defined properties.5 This has provided the impetus for (1) theoretical studies addressing the mechanisms of the initial hydrolysis–condensation processes,6–10 and (2) attempts to recreate some of these reactions in the gas-phase by mass spectrometric techniques in order to probe the intrinsic reactivity pathways of alkoxysilanes.11–20
Because chemical modifications of the alkoxide precursors allow for the incorporation of organic functions and thus alter the functionality and nature of the end material,21–24 there is considerable interest in unravelling new approaches to hydrolysis–condensation reactions. In this communication, we present an unusual set of gas-phase condensation reactions between Cl3−nSi(OH)n+ (n = 1–3) ions and SiCl4 that provide a useful approach for a variety of
Si–O–Si
networks.
Cl3−nSi(OH)n+ (n = 1–3) ions were generated in an in-house built Fourier transform ion cyclotron resonance (FT-ICR) spectrometer by the progressive hydrolysis of gas-phase SiCl3+ ions as described in earlier studies.25,26 The initial hydrolysed ions, the Cl2Si(OH)+ isotopologues, were selectively isolated in the ICR cell using multiple ejection techniques25 and allowed to react with SiCl4 at typical pressures of (3.5 ± 0.5) × 10−8 mbar. Under these conditions, reaction (1) was observed to proceed readily.
| Cl2Si(OH)+ + SiCl4 → +Cl2SiOSiCl3 + HCl | (1) |
The Cl
3SiOSiCl
2+ ions formed in
(1) were then found to undergo rapid hydrolysis with background water (used to generate Cl
2Si(OH)
+ and related species) to yield a series of Si
2OCl
5−n(OH)
n+ (
n = 1–5) ions in an analogous fashion to that described in our previous work.
25 | Si2OCl5−n(OH)n+ + H2O → Si2OCl4−n(OH)n+1+ + HCl (n = 0–4) | (2) |
The rate constant for
reaction (1) was estimated from the successive half-lives taking into account the subsequent
reaction (2). Correction for the ion gauge readings to obtain the absolute pressure of SiCl
4 leads to a rate constant of (2.3 ± 0.7) × 10
−10 cm
3 molecule
−1 s
−1 at a cell temperature of 333 ± 5 K. This value is of the order of 10% of the calculated ion-neutral collision rate constant.
The fact that no equivalent reaction to (1) has been previously observed in the neutral chemistry of SiCl4 and Si2Cl627–31 prompted us to investigate more thoroughly the mechanism of reaction (1). This is of considerable interest because of the important role that chlorosiloxane species play in the functionalization of silica particles.32–34 We therefore decided to explore the mechanistic details for this reaction through computational chemistry. Theoretical calculations were carried out with the Gaussian 03 suite of programs35 to address the possible pathways, to characterize the intermediates and the transition states, and to map out the energy profile of the reaction. Initial calculations for the structure and energy of reagents, products, intermediates and transition states were carried out at the B3LYP/6-311+G(d,p) level of theory with vibrational frequencies corrected by a factor of 0.9679 to estimate zero-point energies (ZPE).36 For comparison purposes, calculations were also performed at the MP2/6-311+G(d,p) level with vibrational frequencies corrected by a factor of 0.9523,37 and with the M05-2X functional with the 6-311+G(d,p) basis set. These different methods were useful for assessing the robustness of the calculated energies and structures in view of possible isomeric structures.38 Transition states were identified by a single imaginary frequency, and intrinsic reaction coordinate (IRC) calculations were carried out to characterize the connection between reaction intermediates and transition states.
The results of these calculations indicate that reaction (1) proceeds by the initial formation of a chloronium ion intermediate followed by an exchange of the bridging chlorine with an OH group as outlined in Scheme 1. The calculated energy profile for this multi-step reaction (Fig. 1) reveals that this pathway is very favourable with the two transition states lying well below the energy of the reagents. Furthermore, reaction (1) is predicted to be exothermic and thus consistent with the ease of the observed ion/molecule reaction. Fig. 2 illustrates the calculated structures of the intermediates and the transition states of the energy profile shown in Fig. 1. Although the calculated values with the B3LYP method are considerably different from those obtained with the MP2 method and the M05-2X functional (see Table 1), the general features of the energy profile are the same.
 |
| Scheme 1 General view of the intermediates involved in the mechanism of reaction (1). | |
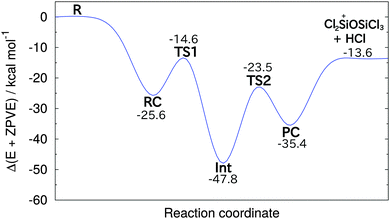 |
| Fig. 1 Calculated energy profile (including zero point energies) for reaction (1) at the MP2/6-311+G(d,p) level of theory. | |
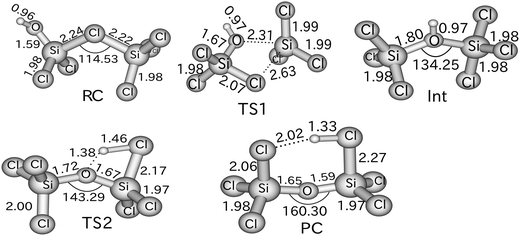 |
| Fig. 2 Optimized geometries calculated at the MP2/6-311+G(d,p) level of theory for the intermediates and transition states outlined in Scheme 1 and Fig. 1. Distances are in Å and angles in degrees. | |
Table 1 Calculated values for (E + ZPE) in kcal mol−1 for the stationary points and transition states of Fig. 1 using different computational chemistry models. The values are referenced to the energy of the reactants
|
B3LYP |
MP2 |
M05-2X |
RC |
−18.3 |
−25.6 |
−25.4 |
TS1 |
−6.9 |
−14.6 |
−12.9 |
Int |
−35.3 |
−47.8 |
−44.6 |
TS2 |
−12.1 |
−23.5 |
−19.9 |
PC |
−26.3 |
−35.4 |
−34.1 |
Products |
−9.0 |
−13.6 |
−10.5 |
The mechanism outlined for reaction (1) is particularly significant because it is found to proceed through a bissilylated halonium ion intermediate. These species have been recognized in recent years as important intermediates for carbon–halogen activation and as precursors of highly reactive Lewis acids.39–43 Similar types of species had been predicted to be stable intermediates in some early theoretical modeling of the reaction between Si+ and SiX4 (X = F, Cl).44
Several other aspects of reactions similar to (1) deserve further consideration. For example, the progressively hydrolyzed ions, Si2OCl4−n(OH)n+1+ (n = 0–4), can undergo further condensation reactions with SiCl4 in an analogous fashion to reaction (1). These secondary reactions were not extensively investigated because they are considerably slower than (1) and the ionic products fall in a mass range above the useful range of our spectrometer.25 Nevertheless, they do provide a convenient pathway for the formation of larger
Si–O–(SiCl2–O–Si(OH)Cl)n–O–Si
networks and variations thereof as shown below.
Different ligands can also be introduced in the networks by making use of the ease with which ions such as Cl3SiOSiCl2+ and Si2OCl4(OH)+ can undergo gas-phase solvolysis type reactions by analogy to our previous work.25 For example, reaction (3) is readily observed in experiments carried out in the presence of methanol at ∼1.5 × 10−8 mbar,
| Si2OCl4(OH)+ + MeOH → Si2OCl3(OH)(OMe)+ + HCl | (3) |
These reactions are predicted to be considerably exothermic by our theoretical calculations. It should be noticed that further condensation reactions of the ionic product of
reaction (3) with SiCl
4 pave the way for a variety of possible
![[triple bond, length as m-dash]](https://www.rsc.org/images/entities/char_e002.gif)
Si–O–(SiCl
2–O–Si(OH)R)
n–O–Si
![[triple bond, length as m-dash]](https://www.rsc.org/images/entities/char_e002.gif)
motifs containing different organic groups R. On the other hand, a similar approach to these reactions using SiF
4 and the corresponding fluorinated ions is predicted to be unfavourable. In fact, the reaction of gaseous F
2Si(OH)
+ with SiF
4, analogous to
reaction (1), is estimated to be endothermic by 7.7 (B3LYP), 5.1 (MP2) and 6.9 (M05-2X) kcal mol
−1 and has not been observed in the earlier study of Speranza and coworkers.
45
In summary, we have shown that a new type of gas-phase reaction represents a novel approach for building up a variety of modified
Si–O–Si
networks. This type of reaction can be further pursued with ions obtained from siloxanol, siloxanediols46 or oligomer diols containing the siloxane structure.47 Furthermore, the mechanism of these reactions illustrates the important role that bissilylated chloronium ions can play in promoting condensation type reactions.
Experimental
The experimental techniques and the FT-ICR spectrometer have been previously described.25 Cl2Si(OH)+ ions were generated from the reaction of SiCl3+ ions (formed inside the ICR cell by the electron ionization of SiCl4) with H2O maintained at a nominal pressure of 1.4 ± 10−8 mbar. The reaction and kinetics of Cl2Si(OH)+ ions (isolated by the removal of all other ions) with SiCl4 maintained at a nominal pressure of Cl2Si(OH)+ of (3.5 ± 0.5) × 10−8 mbar were followed through several half-life periods up to 6 s. The nominal pressures measured by an ionization gauge were corrected to absolute pressures by a procedure similar to that previously used in this laboratory.48 Nevertheless, we estimate that this procedure leads to an uncertainty of 30% in the actual values of the absolute pressures for the present case. The temperature of the cell heated by the filament used for electron ionization in these experiments has been previously measured with a thermocouple and is typically 333 ± 5 K.
Acknowledgements
The authors thank the Brazilian Research Council (CNPq) for continuous support of this work and Jair J. Menegon for technical assistance.
Notes and references
- J. Livage and C. Sanchez, J. Non-Cryst. Solids, 1992, 145, 11 CrossRef CAS.
- L. K. Hench and J. K. West, Chem. Rev., 1990, 90, 33 CrossRef CAS.
- R. Aelion, A. Loebel and F. Eirich, J. Am. Chem. Soc., 1950, 72, 5705 CrossRef CAS.
- J. Sanchez and A. McCormick, J. Phys. Chem., 1992, 96, 8973 CrossRef CAS.
- R. Corriu, J. Organomet. Chem., 2003, 686, 32 CrossRef CAS.
- S. Okumoto, N. Fujita and S. Yamabe, J. Phys. Chem. A, 1998, 102, 3991 CrossRef CAS.
- M. Eleanany, P. Selvam, T. Yokosuka, S. Takami, M. Kubo, A. Imamura and A. Miyamoto, J. Phys. Chem. B, 2003, 107, 1518 CrossRef.
- I. S. Ignatyev, M. Montejo and J. J. J. Lopez Gonzalez, Dalton Trans., 2010, 39, 6967 RSC.
- L. Fernandez, I. Tuñon, J. Latorre, C. Guillem, A. Beltrán and P. Amorós, J. Mol. Model., 2012, 18, 3301 CrossRef CAS PubMed.
- X. Cheng, D. Chen and Y. Liu, ChemPhysChem, 2012, 13, 2392 CrossRef CAS PubMed.
- M. L. P. da Silva and J. M. Riveros, J. Mass Spectrom., 1995, 30, 733 CrossRef.
- M. L. P. da Silva and J. M. Riveros, Int. J. Mass Spectrom. Ion Processes, 1997, 165/166, 83 CrossRef CAS.
- R. Rainone, T. Malaspina, L. A. Xavier and J. M. Riveros, Eur. J. Mass Spectrom., 2010, 16, 379 CrossRef CAS PubMed.
- T. Correra, L. A. Xavier and J. M. Riveros, J. Braz. Chem. Soc., 2013, 24, 256 CrossRef CAS.
- R. Campostrini, G. Carturan, B. Pelli and P. Traldi, J. Non-Cryst. Solids, 1989, 108, 143 CrossRef CAS.
- R. Campostrini, G. Carturan, G. Soraru and P. Traldi, J. Non-Cryst. Solids, 1989, 108, 315 CrossRef CAS.
- S. Cristoni, L. Armelao, E. Tondello and P. Traldi, J. Mass Spectrom., 1999, 34, 1380 CrossRef.
- R. E. Bossio, S. D. Callahan, A. E. Stiegman and A. G. Marshall, Chem. Mater., 2001, 13, 2097 CrossRef CAS.
- N. Söger and M. Binnewies, Z. Anorg. Allg. Chem., 2003, 629, 232 CrossRef.
- A. J. Ward, R. A. Lesic, K. Fisher, N. Proschogo, F. Fábos, A. F. Masters and T. Maschmeyer, Chem. – Eur. J., 2014, 20, 15169 CrossRef CAS PubMed.
- C. Sanchez, J. Livage, M. Henry and F. Babonneau, J. Non-Cryst. Solids, 1988, 100, 65 CrossRef CAS.
- B. Tan and S. E. Rankin, J. Non-Cryst. Solids, 2006, 352, 5453 CrossRef CAS.
- V. G. Kessler, G. I. Spijksma, G. A. Seisenbaeva, S. Håkansson, D. H. A. Blank and H. J. M. Bouwmeester, J. Sol-Gel Sci. Technol., 2006, 40, 163 CrossRef CAS.
- F. Hoffmann and M. Fröba, Chem. Soc. Rev., 2011, 40, 608 RSC.
- T. D. R. Firmino, J. J. Menegon and J. M. Riveros, Quim. Nova, 2010, 33, 2098 CrossRef CAS.
- S. Murthy and J. L. Beauchamp, J. Phys. Chem., 1992, 96, 1247 CrossRef CAS.
- H. K. Park and K. Y. Park, Mater. Res. Bull., 2008, 43, 2833 CrossRef CAS.
- N. Goldberg, M. J. Almond, J. S. Ogden, J. P. Cassidy, R. Walsh and R. Becerra, Phys. Chem. Chem. Phys., 2004, 6, 3264 RSC.
- W. C. Schumb and A. J. Stevens, J. Am. Chem. Soc., 1947, 69, 726 CrossRef CAS.
- W. C. Schumb and A. J. Stevens, J. Am. Chem. Soc., 1950, 72, 3178 CrossRef CAS.
- D. F. Peppard, W. G. Brown and W. C. Johnson, J. Am. Chem. Soc., 1946, 68, 70 CrossRef CAS.
- T. Giesenberg, S. Hein, M. Binnewies and G. Kickelbick, Angew. Chem., Int. Ed., 2004, 43, 5697 CrossRef CAS PubMed.
- M. Binnewies and K. Jug, Eur. J. Inorg. Chem., 2000, 1127 CAS.
-
M. Binnewies and N. Söger, in Silicon Chemistry, ed. P. Jutzi and U. Schubert, Wiley-VCH, Weinheim, 2003, pp. 419–430 Search PubMed.
-
M. J. Frisch, G. W. Trucks, H. B. Schlegel, G. E. Scuseria, M. A. Robb, J. R. Cheeseman, J. A. Montgomery, Jr., T. Vreven, K. N. Kudin, J. C. Burant, J. M. Millam, S. S. Iyengar, J. Tomasi, V. Barone, B. Mennucci, M. Cossi, G. Scalmani, N. Rega, G. A. Petersson, H. Nakatsuji, M. Hada, M. Ehara, K. Toyota, R. Fukuda, J. Hasegawa, M. Ishida, T. Nakajima, Y. Honda, O. Kitao, H. Nakai, M. Klene, X. Li, J. E. Knox, H. P. Hratchian, J. B. Cross, V. Bakken, C. Adamo, J. Jaramillo, R. Gomperts, R. E. Stratmann, O. Yazyev, A. J. Austin, R. Cammi, C. Pomelli, J. W. Ochterski, P. Y. Ayala, K. Morokuma, G. A. Voth, P. Salvador, J. J. Dannenberg, V. G. Zakrzewski, S. Dapprich, A. D. Daniels, M. C. Strain, O. Farkas, D. K. Malick, A. D. Rabuck, K. Raghavachari, J. B. Foresman, J. V. Ortiz, Q. Cui, A. G. Baboul, S. Clifford, J. Cioslowski, B. B. Stefanov, G. Liu, A. Liashenko, P. Piskorz, I. Komaromi, R. L. Martin, D. J. Fox, T. Keith, M. A. Al-Laham, C. Y. Peng, A. Nanayakkara, M. Challacombe, P. M. W. Gill, B. Johnson, W. Chen, M. W. Wong, C. Gonzalez and J. A. Pople, Gaussian 03, Revision C.02, Gaussian, Inc, Wallingford CT, 2004 Search PubMed.
- M. P. Andersson and P. Uvdal, J. Phys. Chem. A, 2005, 109, 2937 CrossRef CAS PubMed.
- J. P. Merrick, D. Moran and L. Radom, J. Phys. Chem. A, 2007, 111, 11683 CrossRef CAS PubMed.
- E. M. L. Fink, A. Schießer, R. Berger and M. C. Holthausen, Int. J. Mass Spectrom., 2013, 354/355, 378 CrossRef.
- P. Ducos, V. Liautard, F. Robert and T. Landais, Chem. – Eur. J., 2015, 21, 11573 CrossRef CAS PubMed.
- A. Budanow, T. Sinke, J. Tillmann, M. Bolte and M. Wagner, Organometallics, 2012, 31, 7298 CrossRef CAS.
- N. Lühmann, H. Hirao, S. Shaik and T. Müller, Organometallics, 2011, 30, 4087 CrossRef.
- M. Lehmann, A. Schulz and A. Villinger, Angew. Chem., Int. Ed., 2009, 48, 7444 CrossRef CAS PubMed.
- R. Panish, M. Bolte and T. Müller, J. Am. Chem. Soc., 2006, 128, 9676 CrossRef PubMed.
- C. L. Darling and H. B. Schlegel, J. Phys. Chem., 1993, 97, 1368 CrossRef CAS.
- F. Grandinetti, G. Occhiucci, O. Ursini, G. de Petris and M. Speranza, Int. J. Mass Spectrom., 1993, 124, 21 CrossRef CAS.
- M. Liu, N. T. Tran, A. K. Franz and J. K. Lee, J. Org. Chem., 2011, 76, 7186 CrossRef CAS PubMed.
- C. Rücker and K. Kümmerer, Chem. Rev., 2015, 115, 466 CrossRef PubMed.
- T. Giroldo, L. A. Xavier and J. M. Riveros, Angew. Chem., Int. Ed., 2004, 43, 3588 CrossRef CAS PubMed.
Footnotes |
† Electronic supplementary information (ESI) available: Cartesian coordinates and calculated energies for all the species in Fig. S1. See DOI: 10.1039/c5nj02250a |
‡ Present address: Laboratoire de Chimie Physique, CNRS UMR 8000, Université Paris-Sud, 15, rue Jean Perrin, 91405 Orsay Cedex, France. E-mail: E-mail: thiago.firmino@u-psud.fr |
|
This journal is © The Royal Society of Chemistry and the Centre National de la Recherche Scientifique 2016 |