DOI:
10.1039/C5NJ02179K
(Paper)
New J. Chem., 2016,
40, 651-661
NMR, surface tension and conductivity studies to determine the inclusion mechanism: thermodynamics of host–guest inclusion complexes of natural amino acids in aqueous cyclodextrins†
Received
(in Montpellier, France)
21st August 2015
, Accepted 12th November 2015
First published on 16th November 2015
Abstract
The assembly of two natural amino acids (viz., L-arginine and L-histidine) as guests with α and β-cyclodextrins as hosts to form inclusion complexes in aqueous medium has been demonstrated which are highly suitable for diverse applications in modern bio-medical sciences. The 1H NMR study establishes the formation of inclusion complexes, while surface tension and conductivity studies confirm that the inclusion complexes have been formed with 1
:
1 stoichiometry. The nature of the complexes has been established using thermodynamic parameters, based on density, viscosity, and refractive index measurements. The contributions of different groups of the guest molecules towards the limiting apparent molar volume and the viscosity-B coefficient are determined and solvation numbers are calculated. All the parameters support the formation of the inclusion complexes, which are explained based upon hydrophobic effects, H-bonds, electrostatic forces and structural effects.
1. Introduction
In modern science cyclodextrins are extensively used for the controlled release of compounds due to their exceptional capability to form inclusion complexes with a variety of guest molecules, thus it is an important task to follow whether a molecule forms an inclusion complex with cyclodextrins.1,2 Cyclodextrins are cyclic oligosaccharides containing six (α-CD), seven (β-CD) and eight (γ-CD) glucopyranose units, which are bound by α-(1–4) linkages forming a truncated conical structure having a hydrophobic interior and hydrophilic rims containing primary and secondary –OH groups (Scheme 1).
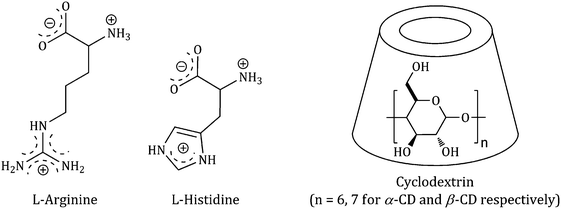 |
| Scheme 1 Molecular structure of selected α-amino acids in aqueous solution and the structure of the cyclodextrin host molecule. | |
Hence, because of having exceptional structures, they can build up stable host–guest inclusion complexes by accommodating the non-polar component of the guest molecule in their hydrophobic cavity and stabilizing the polar part of the guest molecule by the polar rims.3,4 This explains the modern-day interest in cyclodextrins in the controlled release of bio-active molecules (e.g., drugs, vitamins, amino acids etc.), food flavours, deodorisers, paint ingredients etc. as well as the removal of toxic materials, waste products and pollutants without any chemical modification.5
In the present work two natural amino acids (viz., L-arginine and L-histidine) have been studied with α and β-cyclodextrins to observe whether they form a host–guest inclusion complex by the study of 1H NMR, surface tension and conductivity. The nature of the inclusion complexes is established by density, viscosity and refractive index measurements by calculating the contributions towards the limiting apparent molar volume and the viscosity-B coefficient of different groups of the guest molecules, solvation number and limiting molar refraction by taking 0.001, 0.003, 0.005 mass fractions of α and β-cyclodextrins in aqueous medium.
2. Result and discussion
2.1.
1H NMR study confirms the inclusion phenomenon
The insertion of a guest molecule into the hydrophobic cavity of α and β-CD results in the chemical shift of the protons of the cyclodextrin molecule in the 1H NMR spectra, which is due to the interaction of the host cyclodextrin with the guest molecule.6 In the structure of cyclodextrin the H3 and H5 hydrogens are located inside the conical cavity, particularly, H3 is placed near the wider rim while H5 is placed near the narrower rim of the cyclodextrin molecule. The other H1, H2 and H4 hydrogens are situated at the exterior of the cyclodextrin molecule (Scheme 2).7
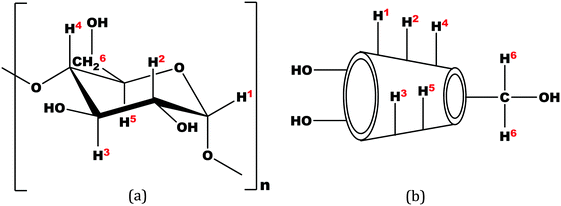 |
| Scheme 2 (a) Stereo-chemical configuration of α and β-cyclodextrin, (b) truncated conical structure of α and β-cyclodextrins. | |
Thus when a guest molecule enters into the cavity of cyclodextrin it interacts with the H3 and H5 protons, resulting in the upfield chemical shift of these protons. As H3 is located near the wider rim of cyclodextrin, through which usually the guest enters, the shift is higher for it than that for the H5 proton which is situated near the narrower rim at the interior of cyclodextrin. The other H1, H2 and H4 hydrogens also show an upfield chemical shift, but it is lower compared to that of the interior protons.8
In the present work the molecular interactions of L-Arg and L-His with α and β-cyclodextrins have been studied using the 1H NMR spectra by taking a 1
:
1 molar ratio of the amino acid and α or β-cyclodextrin in D2O at 298.15 K. It has been found that there are considerable upfield shifts (Δδ) of the interior H3 and H5 protons, but little shifts of the exterior H1, H2 and H4 protons of cyclodextrin, as well as those of the interacting protons of the amino acids (Fig. 1–4).
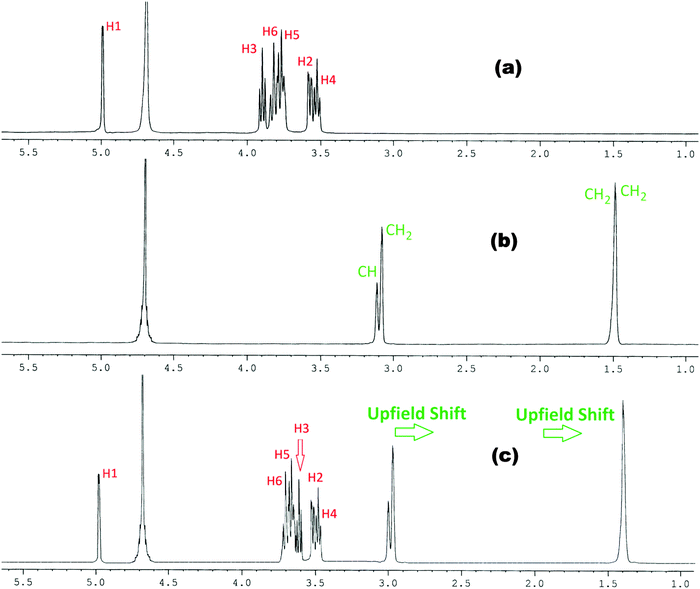 |
| Fig. 1
1H NMR spectra of (a) α-CD, (b) arginine and (c) 1 : 1 molar ratio of α-CD & arginine in D2O at 298.15 K. | |
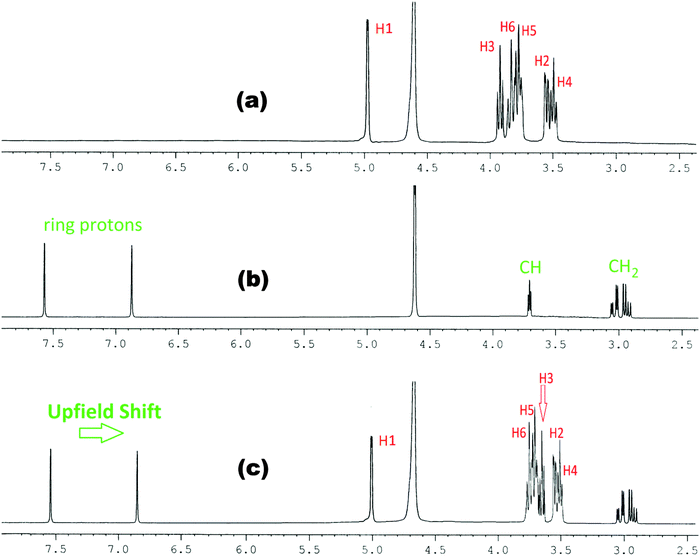 |
| Fig. 2
1H NMR spectra of (a) α-CD, (b) histidine and (c) 1 : 1 molar ratio of α-CD & histidine in D2O at 298.15 K. | |
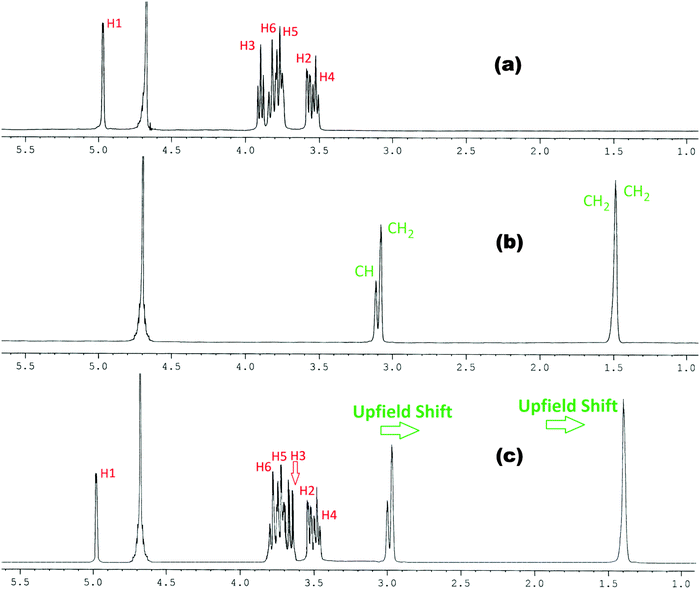 |
| Fig. 3
1H NMR spectra of (a) β-CD, (b) arginine and (c) 1 : 1 molar ratio of β-CD & arginine in D2O at 298.15 K. | |
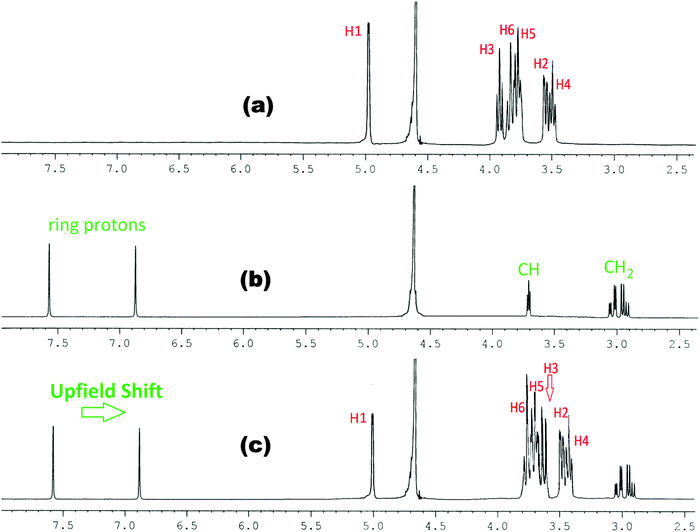 |
| Fig. 4
1H NMR spectra of (a) β-CD, (b) histidine and (c) 1 : 1 molar ratio of β-CD & histidine in D2O at 298.15 K. | |
This establishes that the inclusion phenomenon has occurred between the chosen host and guest molecules. Upon inclusion the upfield chemical shift values (Δδ) of the H3 and H5 protons of α and β-cyclodextrins have been listed in Table 1, which show that the interaction of the guest amino acids with H3 is greater than that with H5, suggesting that the inclusion has taken place through the wider rim of the α and β-cyclodextrins.
Table 1 Change in chemical shifts (ppm) of the H3 and H5 protons of cyclodextrin host molecules when complexed with amino acid guest molecules in D2O at 298.15 Ka
|
Δδ |
L-Arginine |
L-Histidine |
H3 |
H5 |
H3 |
H5 |
Standard uncertainties in temperature u are: u(T) = 0.01 K.
|
α-Cyclodextrin |
0.278 |
0.088 |
0.208 |
0.052 |
β-Cyclodextrin |
0.283 |
0.099 |
0.252 |
0.062 |
2.2. Surface tension study explains the inclusion as well as the stoichiometric ratio of the inclusion complexes
Amino acid molecules being present in zwitterionic structures show a considerable increase in the surface tension of their aqueous solution, while aqueous cyclodextrin solution does not show any remarkable change in surface tension compared to the pure aqueous solvent.9 Thus while amino acids make inclusion complexes with cyclodextrins a remarkable change in surface tension should be observed, also getting single, double, etc. breaks in the surface tension curve indicating 1
:
1, 1
:
2, etc. stoichiometries of the host and the guest in the formed inclusion complex (Scheme 3).10,11
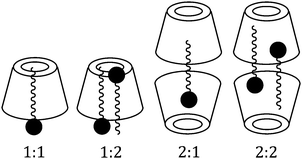 |
| Scheme 3 Different possibilities of the host–guest ratio for the inclusion complex. | |
In the present study the guest amino acid molecules exist in zwitterionic forms and also contain basic side groups, thereby having charge in their molecules, thus there might be some ionic interactions between the charged groups resulting in an increase in surface tension of the aqueous solution, which would be distinctly affected in the presence of α or β-CD. Here a set of solutions has been prepared having 10 mmol L−1 concentration of L-Arg or L-His with increasing concentration of α or β-CD and the surface tension is measured at 298.15 K. The trend of the surface tension curve is found to be progressively falling with increased concentration of α and β-CD, which may be attributed to the formation of the inclusion complex (Fig. 5a and b).
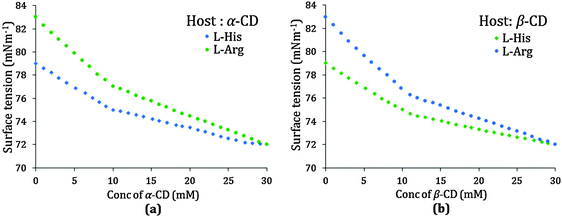 |
| Fig. 5 Variation of surface tension of L-arginine solution (10.0 mmol L−1) and L-histidine solution (10.0 mmol L−1) with increasing concentration of (a) α-cyclodextrin and (b) β-cyclodextrin respectively at 298.15 K. | |
The curves for both the amino acids are similar, but the slope of L-Arg is higher than that of L-His, which may be due to a greater number of L-Arg molecules present in the charged structure than that of L-His, both of which are encapsulated in the cyclodextrin cavity as the inclusion occurs. Single discernible breaks at about 10 mmol L−1 concentration of both α and β-CD are found for all the possible four cases indicating the 1
:
1 stoichiometric ratio for each of the inclusion complexes formed (Table 2).
Table 2 Values of surface tension (γ) and conductivity (κ) at the break point with corresponding concentration of aqueous α and β-cyclodextrins at 298.15 Ka
|
Surface tension |
L-Arginine |
L-Histidine |
Conc./mM |
γ/mN m−1 |
Conc./mM |
γ/mN m−1 |
Standard uncertainties in temperature u are: u(T) = 0.01 K.
|
α-Cyclodextrin |
9.9 |
76.5 |
9.8 |
74.5 |
β-Cyclodextrin |
9.9 |
76.0 |
9.8 |
74.0 |
|
Conductivity |
L-Arginine |
L-Histidine |
Conc./mM |
κ/μS m−1 |
Conc./mM |
κ/μS m−1 |
α-Cyclodextrin |
10.1 |
176 |
10.5 |
65 |
β-Cyclodextrin |
10.0 |
170 |
11.0 |
64 |
2.3. Conductivity study illustrates the inclusion process and their stoichiometric ratio
The measurement of the conductivity of a solution is an important tool to elucidate the inclusion phenomenon. The study of conductivity is not only a very common method to illuminate the host–guest inclusion event but also to identify the stoichiometry of the inclusion complex formed.12–14 As the amino acid molecule enters into the hydrophobic cavity of α or β-CD the conductivity of the solution decreases gradually, i.e., the conductivity of the solution is markedly affected by the inclusion phenomenon. In this study the conductivities of a series of solutions having 10 mmol L−1 concentration of aqueous L-Arg or L-His with increasing concentration of either of the two cyclodextrins have been measured, and the trend of the conductivity is found to be regularly declining which is obvious due to the formation of the inclusion complex between cyclodextrin and amino acid (Fig. 6a and b).
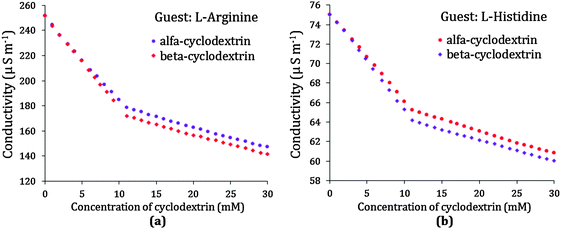 |
| Fig. 6 Variation of conductivity of aqueous (a) L-arginine solution (10.0 mmol L−1) and (b) L-histidine solution (10.0 mmol L−1) respectively with increasing concentration of α and β-cyclodextrin at 298.15 K. | |
A noticeable break is found in the conductivity curve at around a 10 mmol L−1 concentration for both α and β-CD, suggesting that the stoichiometry of the amino acid-cyclodextrin inclusion complex is equimolar, i.e., a 1
:
1 host–guest inclusion complex is formed (Scheme 3). A complex or greater number of breaks in the conductivity curve suggests different stoichiometry e.g., 1
:
2, 2
:
1, 2
:
2 etc. for the inclusion complex. In this study of all four cases of L-Arg and L-His with α and β-CD similar results are found, but the conductivity near the break is found to be a little lower for β-CD than α-CD, which might be due to the fact that the former is a better host for the two studied guests than the latter (Table 2).
2.4. pH study confirms the ionic states of the amino acids
pH measurement is a handy tool for understanding the structures of the zwitterionic forms of amino acids in aqueous solution.15 The values of pH for L-Arg and L-His in both the aqueous α and β-CD increase with increasing concentration of the respective amino acids (Table S3 in the ESI†). The ranges of the pH values at 298.15 K for the two selected amino acids in the aqueous α and β-CD solutions under experimental consideration are 7.38–7.56 and 9.80–10.73 for L-His and L-Arg, respectively. These values clearly show the variation in their zwitterionic forms, i.e., besides existing as –NH3+ and –COO− of the amine and carboxylic acid groups, the side chains of the amino acids are present as cationic groups by acquiring a proton from the aqueous solution, thus by increasing the pH value of the solution.
2.5. Density study: group contributions and interactions between amino acids and cyclodextrins
The apparent molar volume (ϕv) and limiting apparent molar volume (ϕ0v) are considered as sensitive tools for understanding the interactions taking place in solutions. The apparent molar volume is the measure of the sum of the geometric volume of the central solute molecule and changes in the solvent volume due to its interaction with the solute around the co-sphere. Here ϕv has been determined from the measured density of the solutions at 298.15 K (Table S3, ESI†) and by using the suitable equation, the magnitude of which is found to be positive for all the systems under study, indicating strong solute–solvent interactions.9,16ϕv varies linearly with the square root of molal concentration (Table S5, ESI†) and is fitted to the Masson equation, from where the limiting apparent molar volume (ϕ0v) has been determined (Table S6, ESI†).17 The values of ϕ0v increase with the increase of mass fractions of α and β-CD for both L-Arg and L-His indicating that the ion-hydrophilic group interactions are stronger than the ion-hydrophobic group interactions. In the present ternary system interactions are taking place between the zwitterionic groups and the side chain ionic groups of the amino acids with the localized hydroxyl groups of cyclodextrins, with the electrostriction of water resulting in an increase in volume. The ϕ0v values for both the amino acids and cyclodextrins at different mass fractions are represented in Fig. 7, which suggests that ϕ0v of L-Arg is greater than that of L-His due to greater electrostriction which is further due to the presence of additional methylene groups (that lengthens the chain increasing the hydrophobic interaction) and the guanidine group (that interacts better than the imidazole group with the –OH groups of cyclodextrin) that provide an enforcing tendency in L-Arg resulting in a net increase in volume.
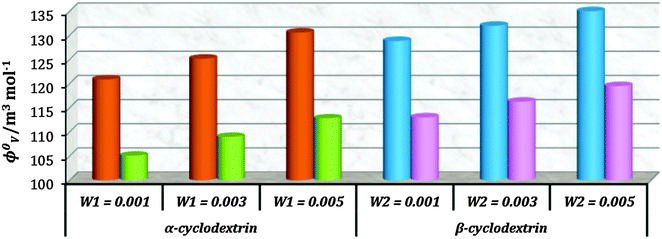 |
| Fig. 7 Plot of limiting molar volume (ϕ0v) against mass fraction (w) of aq. α-CD and aq. β-CD for L-arginine (orange & blue) and L-histidine (green & pink) respectively at 298.15 K. | |
The consequences for the selected amino acids can be recognized on the basis of the limiting partial molar volume which is found to be higher for L-Arg than L-His. In this study the values of ϕ0v are measured for glycine, L-Arg and L-His at 298.15 K for different mass fractions of α and β-CD. If one H from the side chain of glycine is replaced by
and
side groups L-Arg and L-His are found respectively, so there should be a correlation among the structures of the amino acids as well as in the values of ϕ0v, which greatly affects the inclusion complexes taking place in the solution systems.9,14,16 The variations of ϕ0v for different groups present in L-Arg and L-His with different mass fractions of α and β-CD have been estimated (Table 3). It is observed that the contribution of the zwitterionic group (NH3+,COO−) is in the range of 23.24–25.76 × 10−6 m3 mol−1 and 25.32–27.48 × 10−6 m3 mol−1 for α and β-CD respectively, which indicates that the interactions between the –OH groups of cyclodextrins and the polar head groups (NH3+,COO−) of amino acids are strong, but they are stronger for β-CD than α-CD. The contributions of hydrophobic (CH) and (CH2) groups decrease and those of the hydrophilic
and
groups increase with the increase of the mass fractions of both the cyclodextrins, which suggest that the ion–dipolar interactions increase over the hydrophobic interactions for the two selected amino acids when the mass fractions of both the cyclodextrins are increased in the solution. It is observed that the contribution to ϕ0v for
is more than that for
; but as the contributions of the hydrophobic alkyl groups are summed the overall ϕ0v for L-Arg is found to be greater than that for L-His with both the aqueous cyclodextrin solutions.
Table 3 Contributions of the zwitterionic group (NH3+), (COO−); (CH), (CH2) groups and the end group to the limiting apparent molar volume (ϕ0v) and the viscosity B-coefficient for amino acids in different mass fractions of aqueous α and β-cyclodextrins respectively at 298.15 Ka
Groups |
ϕ
0v × 10−6/ m3 mol−1 |
B/kg1/2 mol−1/2 |
w
1 = 0.001b |
w
1 = 0.003b |
w
1 = 0.005b |
w
1 = 0.001b |
w
1 = 0.003b |
w
1 = 0.005b |
Standard uncertainties u are: u(T) = 0.01 K.
w
1 and w2 are mass fractions of α and β-cyclodextrins in an aqueous mixture respectively.
|
(NH3+), (COO−) |
23.24 |
24.88 |
25.76 |
0.096 |
0.098 |
0.100 |
(CH) |
8.99 |
8.38 |
8.16 |
0.028 |
0.029 |
0.030 |
(CH2) |
17.98 |
16.76 |
16.32 |
0.056 |
0.058 |
0.060 |
(CH2)3 |
53.94 |
50.28 |
48.96 |
0.168 |
0.174 |
0.180 |
|
34.69 |
41.64 |
47.70 |
0.230 |
0.237 |
0.276 |
|
54.87 |
58.96 |
62.56 |
0.322 |
0.343 |
0.358 |
|
w
2 = 0.001b |
w
2 = 0.003b |
w
2 = 0.005b |
w
2 = 0.001b |
w
2 = 0.003b |
w
2 = 0.005b |
(NH3+), (COO−) |
25.32 |
26.82 |
27.48 |
0.100 |
0.104 |
0.108 |
(CH) |
8.28 |
7.65 |
7.44 |
0.026 |
0.027 |
0.028 |
(CH2) |
16.56 |
15.30 |
14.88 |
0.052 |
0.054 |
0.056 |
(CH2)3 |
49.68 |
45.90 |
44.64 |
0.156 |
0.162 |
0.168 |
|
45.56 |
51.61 |
55.62 |
0.264 |
0.291 |
0.298 |
|
62.86 |
66.51 |
69.70 |
0.350 |
0.361 |
0.392 |
2.6. Viscosity: group contribution and solvation number: degree of solvation by cyclodextrin molecules
The viscosity of the aqueous cyclodextrin solution increases with increasing mass fraction of α or β-CD (Table S1, ESI†) due to the structure making contribution of cyclodextrins with water molecules. In the studied ternary systems the viscosity is found to be increasing with increasing molality of amino acids (Table S3, ESI†). The viscosity B-coefficients (Table S6, ESI†), which are an indication of solute–solvent interactions, are found to be all positive (Fig. 8), and increase with the increasing concentration of α and β-CD, which is considered to arise because of increasing amino acid–cyclodextrin interaction as well as increase in the solvation.18,19
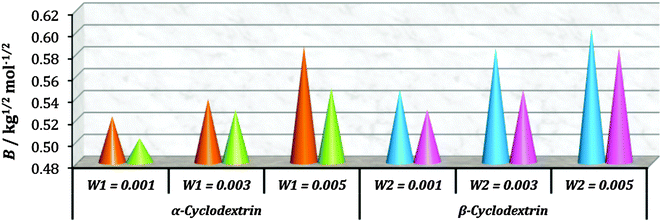 |
| Fig. 8 Plot of the viscosity B-coefficient against mass fraction (w) of aq. α-CD and aq. β-CD for L-arginine (orange & blue) and L-histidine (green & pink) respectively at 298.15 K. | |
The contributions of different groups of amino acids to the viscosity B-coefficient have been derived (Table 3).9,14,16 The contributions of the zwitterionic group (NH3+,COO−) and the polar groups
and
increase with increasing mass fractions of α and β-CD, suggesting the greater solvation of the ionic groups with the –OH groups of cyclodextrin molecules, while those of the hydrophobic (CH), (CH2) groups are found to increase demonstrating the increased solvation of the hydrophobic part of the amino acids inside the hydrophobic cavity of α and β-CD.
In the present study solvation number is a measure of the interaction taking place between the primary or secondary hydroxyl groups of cyclodextrins and the zwitterionic or the polar side groups of amino acids, this is because as the mass fraction of the cyclodextrins increases in the ternary solution system the electrostriction of water diminishes resulting in an increase in the solvation number (Table 4), which is found to be higher for L-Arg than L-His, this may be explained by L-Arg containing longer alkyl chains and the more basic guanidine group than L-His, the encapsulation of the hydrophobic part inside the cavity of cyclodextrin is greater and the guanidine group interacts better with the –OH groups of cyclodextrin replacing the surrounded water molecules.9,20
Table 4 Solvation number (Sn) of the amino acids at different mass fractions of aqueous α and β-cyclodextrins respectively at 298.15 Ka
|
S
n
|
w
1 = 0.001b |
w
1 = 0.003b |
w
1 = 0.005b |
Standard uncertainties u are: u(T) = 0.01 K.
w
1 and w2 are mass fractions of α and β-cyclodextrins in the aqueous mixture respectively.
|
L-Arginine |
4.59 |
4.62 |
4.66 |
L-Histidine |
4.50 |
4.53 |
4.58 |
|
w
2 = 0.001b |
w
2 = 0.003b |
w
2 = 0.005b |
L-Arginine |
4.65 |
4.68 |
4.72 |
L-Histidine |
4.56 |
4.60 |
4.65 |
The solvation number in the case of β-CD is observed to be higher than that of α-CD, this is probably due to the greater number of –OH groups (primary and secondary) coordinating with the appropriate phase of interaction with the zwitterionic and polar side groups replacing the water molecules around the amino acid molecules.
2.7. Refractive index demonstrates the compactness of the inclusion complexes
The refractive index (nD) and molar refraction (RM) are considered as valuable tools for investigating the molecular interactions taking place in solution systems (Tables S3 and S5, ESI†). As the interaction between the solute and the solvent (here formation of the inclusion complex between amino acids and cyclodextrins) increases the medium becomes more compact, resulting in a higher value of the limiting molar refraction (R0M) (Table S6, ESI†).9,21 Therefore, it is evident from Fig. 9 that the inclusion complexes of L-Arg with both the α and β-CD are more dense or closely packed than those of L-His, which may be explained as due to greater hydrophilic as well as hydrophobic interactions between L-Arg and both the cyclodextrins. These findings are in good agreement with the results found from density and viscosity measurements.
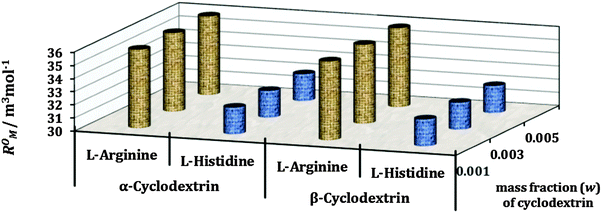 |
| Fig. 9 Plot of limiting molar refraction (R0M) for L-arginine and L-histidine in different mass fractions (w) of aq. α-CD and aq. β-CD respectively at 298.15 K. | |
2.8. Structural influence of cyclodextrins
The formation of a host–guest inclusion complex depends on the size of the guest molecule as well as the cavity diameter of the host molecule, thus it is a dimensional suitability between these two species.9 The uniqueness of the cyclodextrin molecule is the hydrophobic cavity and hydrophilic rims, which provide an appropriate environment for the apolar part of a molecule to reside inside the cavity, while the polar part makes association with the polar rims, thereby stabilizing the whole inclusion complex.1,22 Another driving force for the formation of the inclusion complex is the release of the water molecules from the hydrophobic cavity into the bulk, which is an entropy driven process.14 The stoichiometry of the inclusion complex is found to be 1
:
1 (Scheme 3) from conductivity and surface tension measurements, which may be explained on the basis of the fact that after inclusion of one amino acid molecule it would be difficult for a second molecule to be inserted into the cavity because the zwitterionic part and the ionic side group make some kind of blockage at the wider rim of the host molecule.9 The insertion of the guest amino acid molecule is expected from the wider rim of the cyclodextrin molecule, so as to make maximum contact of the alkyl groups with the cyclodextrin cavity (Scheme 4), which is also supported by NMR data.
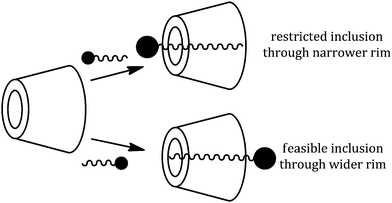 |
| Scheme 4 Feasible and restricted inclusion of the guest into the host molecule. | |
The charged terminal groups are projected toward the aqueous environment and can make H-bonds with the –OH groups at both rims of the cyclodextrin molecule (Scheme 5). Thus the stabilizing factors for the formation of the inclusion complexes are firstly, the displacement of polar water molecules from the apolar cavity of cyclodextrin and return to the bulk of water, making a large number of H-bonds; secondly, a decrease of the repulsive forces between the hydrophobic alkyl groups of amino acids in the aqueous environment and an increase in the hydrophobic interactions as the inclusion takes place in the apolar cavity of cyclodextrin; and finally, the inclusion complex is stabilized by the formation of H-bonds between the polar groups of amino acids and the primary as well as the secondary –OH groups at both the rims of α and β-CD (Scheme 5).
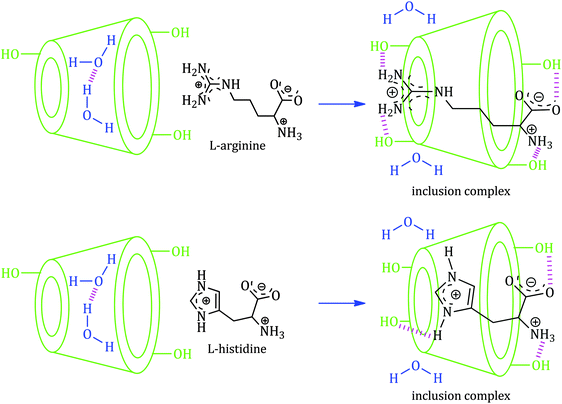 |
| Scheme 5 Schematic representation of the mechanism for the formation of the 1 : 1 inclusion complex of selected α-amino acids with both α and β-cyclodextrin molecules. | |
3. Conclusion
The present study concludes that the two essential amino acids, viz., L-Arg and L-His form host–guest inclusion complexes with α and β-CD. The NMR study confirms the inclusion phenomenon while surface tension and conductivity studies reveal that 1
:
1 inclusion complexes have been formed. Density, viscosity and refractive index measurements are used to characterize the formed inclusion complexes by determining the group contributions of the limiting apparent molar volume and the viscosity-B coefficient, as well as the solvation number and limiting molar refraction. All the findings support the formation of the inclusion complexes and thus the current work describes its appropriateness towards miscellaneous applications as a controlled delivery system in the field of modern bio-medical sciences.
4. Experimental section
4.1. Source and purity of samples
The selected amino acids and cyclodextrins of puriss grade were bought from Sigma-Aldrich, Germany and used as purchased. The mass fraction purities of L-Arg, L-His, α-cyclodextrin and β-cyclodextrin were ≥0.98, 0.99, 0.98 and 0.98 respectively.
4.2. Apparatus and procedure
The solubility of the selected cyclodextrins and that of the selected amino acids in aq. cyclodextrins has been precisely checked in triply distilled, deionized and degassed water and it was observed that these were freely soluble in all proportions of aq. cyclodextrins. All the stock solutions of the amino acids were prepared by mass (measured using Mettler Toledo AG-285 with uncertainty 0.0001 g), and the working solutions were obtained by mass dilution at 298.15 K. The conversions of molarity to molality have been done using density values.23 Adequate precautions were taken to reduce evaporation losses during mixing.
NMR spectra were recorded in D2O unless otherwise stated. 1H NMR spectra were recorded at 400 MHz and 500 MHz using Bruker ADVANCE 400 MHz and Bruker ADVANCE 500 MHz instruments respectively at 298.15 K. Signals are quoted as δ values in ppm using residual protonated solvent signals as the internal standard (D2O: δ 4.79 ppm). Data are reported as chemical shifts.
The surface tension experiments were done by the platinum ring detachment method using a Tensiometer (K9, KRŰSS; Germany) at the experimental temperature. The accuracy of the measurement was within ±0.1 mN m−1. The temperature of the system has been maintained by circulating auto-thermostated water through a double-wall glass vessel containing the solution.
Specific conductance values of the experimental solutions were measured by a Mettler Toledo Seven Multi conductivity meter with uncertainty 1.0 μS m−1. The measurements were made in a thermostated water bath maintaining the temperature at 298.15 K and using the HPLC grade water with a specific conductance of 6.0 μS m−1. The cell was calibrated using a 0.01 M aqueous KCl solution. The uncertainty in temperature was 0.01 K.
pH values of the experimental solutions were measured by a Mettler Toledo Seven Multi pH meter with an uncertainty of 0.009. The measurements were made in a thermostated water bath maintaining the temperature at 298.15 K. The uncertainty in temperature was 0.01 K.
The densities (ρ) of the solvents were measured by means of a vibrating U-tube Anton Paar digital density meter (DMA 4500M) with a precision of ±0.00005 g cm−3 maintained at ±0.01 K of the desired temperature. It was calibrated by passing triply distilled, degassed water and dry air.
The viscosities (η) were measured using a Brookfield DV-III Ultra Programmable Rheometer with fitted spindle size-42. The detailed description has already been described earlier.10
Refractive indexes were measured using a Digital Refractometer Mettler Toledo. The light source was LED, λ = 589.3 nm. The refractometer has been calibrated twice using distilled water and calibration has been checked after every few measurements. The uncertainty of refractive index measurements was ±0.0002 units.
4.3.
1H NMR data
α-Cyclodextrin.
1H NMR (500 MHz, D2O): δ = 3.48–3.51 (6H, t, J = 9.00 Hz), 3.53–3.56 (6H, dd, J = 10.00, 3.00 Hz), 3.74–3.83 (18H, m), 3.87–3.91 (6H, t, J = 9 Hz), 4.97–4.96 (6H, d, J = 3 Hz).
β-Cyclodextrin.
1H NMR (400 MHz, D2O): δ = 3.49–3.54 (6H, t, J = 9.2 Hz), 3.57–3.60 (6H, dd, J = 9.6, 3.2 Hz), 3.79–3.84 (18H, m), 3.87–3.92 (6H, t, J = 9.2 Hz), 5.00–5.01 (6H, d, J = 3.6 Hz).
L-Arginine.
1H NMR (500 MHz, D2O): δ = 1.48–1.53 (4H, m), 3.07–3.08 (2H, m), 3.13–3.14 (1H, m).
L-Histidine.
1H NMR (500 MHz, D2O): δ = 3.01–3.16 (2H, m), 3.85–3.86 (1H, m), 6.97 (1H, s), 7.67 (1H, s).
α-CD + L-Arg.
1H NMR (500 MHz, D2O): δ = 1.39–1.43 (4H, m), 2.97–2.98 (2H, t, J = 6.5 Hz), 3.03–3.04 (1H, t, J = 5.5 Hz), 3.43–3.46 (6H, t, J = 9.00 Hz), 3.49–3.52 (6H, dd, J = 10.00, 3.00 Hz), 3.59–3.63 (6H, t, J = 9 Hz), 3.66–3.75 (18H, m), 4.97–4.96 (6H, d, J = 3 Hz).
α-CD + L-His.
1H NMR (400 MHz, D2O): δ = 3.00–3.16 (2H, m), 3.84–3.85 (1H, m), 6.89 (1H, s), 7.59 (1H, s), 3.46–3.49 (6H, t, J = 9.00 Hz), 3.51–3.54 (6H, dd, J = 10.00, 3.00 Hz), 3.66–3.70 (6H, t, J = 9 Hz), 3.69–3.78 (18H, m), 4.97–4.96 (6H, d, J = 3 Hz).
β-CD + L-Arg.
1H NMR (400 MHz, D2O): δ = 1.38–1.42 (4H, m), 2.96–2.97 (2H, t, J = 6.5 Hz), 3.02–3.03 (1H, t, J = 5.5 Hz), 3.44–3.49 (6H, t, J = 9.2 Hz), 3.53–3.56 (6H, dd, J = 9.6, 3.2 Hz), 3.59–3.64 (6H, t, J = 9.2 Hz), 3.69–3.74 (18H, m), 5.00–5.01 (6H, d, J = 3.6 Hz).
β-CD + L-His.
1H NMR (400 MHz, D2O): δ = 2.97–3.13 (2H, m), 3.84–3.85 (1H, m), 6.88 (1H, s), 7.58 (1H, s), 3.45–3.50 (6H, t, J = 9.2 Hz), 3.54–3.57 (6H, dd, J = 9.6, 3.2 Hz), 3.62–3.67 (6H, t, J = 9.2 Hz), 3.73–3.78 (18H, m), 5.00–5.01 (6H, d, J = 3.6 Hz).
Acknowledgements
The authors are grateful to the Special Assistance Scheme, Department of Chemistry, NBU under the University Grants Commission, New Delhi for financial sustenance and instrumental facilities in order to carry out this research work. Prof. M. N. Roy is also highly obliged to the University Grants Commission, New Delhi, Government of India for being awarded a one time Grant under Basic Scientific Research concerning his dynamic service for augmenting of research facilities to expedite the advanced research work.
References
- J. Szejtli, Chem. Rev., 1998, 98, 1743–1753 CrossRef CAS PubMed.
- W. Saenger, Angew. Chem., Int. Ed. Engl., 1980, 19, 344–362 CrossRef.
-
M. L. Bender and M. Komiyama, Cyclodextrin Chemistry, Springer-Verlag, New York, 1978 Search PubMed.
-
J. Szejtli, in Inclusion of guest molecules, selectivity and molecular recognition by cyclodextrins, incomprehensive supramolecular chemistry, ed. J. Szejtli and T. Osa, Elsevier, Oxford, 1996, vol. 3, pp. 189–203 Search PubMed.
- Y. Gao, X. Zhao, B. Dong, L. Zheng, N. Li and S. Zhang, J. Phys. Chem. B, 2006, 110, 8576–8581 CrossRef CAS PubMed.
- K. P. Sambasevam, S. Mohamad, N. M. Sarih and N. A. Ismail, Int. J. Mol. Sci., 2013, 14, 3671–3682 CrossRef CAS PubMed.
- J. V. Caso, L. Russo, M. Palmieri, G. Malgieri, S. Galdiero, A. Falanga, C. Isernia and R. Iacovino, Amino Acids, 2015, 47, 2215–2227 CrossRef CAS PubMed.
- T. Wang, M. D. Wang, C. D. Ding and J. Fu, Chem. Commun., 2014, 50, 12469–12472 RSC.
- M. N. Roy, D. Ekka, S. Saha and M. C. Roy, RSC Adv., 2014, 4, 42383–42390 RSC.
- D. Ekka and M. N. Roy, RSC Adv., 2014, 4, 19831–19845 RSC.
- A. Pineiro, X. Banquy, S. P. Casas, E. Tovar, A. Garcia, A. Villa, A. Amigo, A. E. Mark and M. Costas, J. Phys. Chem. B, 2007, 111, 4383–4392 CrossRef CAS PubMed.
- Y. Gao, Z. Li, J. Du, B. Han, G. Li, W. Hou, D. Shen, L. Zheng and G. Zhang, Chem. – Eur. J., 2005, 11, 5875–5880 CrossRef CAS PubMed.
- A. Apelblat, E. Manzurola and Z. Orekhova, J. Solution Chem., 2007, 36, 891–900 CrossRef CAS.
- H. Shekaari and F. Jebali, Phys. Chem. Liq., 2011, 49, 572–587 CrossRef CAS.
- K. A. Connors and J. M. Lipari, J. Pharm. Sci., 1976, 65, 379–383 CrossRef CAS PubMed.
- D. Ekka and M. N. Roy, Amino Acids, 2013, 45, 755–777 CrossRef CAS PubMed.
- D. O. Masson, Philos. Mag., 1929, 8, 218–226 CrossRef CAS.
-
R. H. Stokes and R. Mills, Viscosity of Electrolytes and Related Properties. International Encyclopedia of Physical Chemistry and Chemical Physics, Pergamon, London, 1965 Search PubMed.
- H. J. V. Tyrrell and M. Kennerley, J. Chem. Soc. A, 1968, 2724 RSC.
- T. Ogawa, M. Yasuda and K. Mizutani, Bull. Chem. Soc. Jpn., 1984, 57, 662–666 CrossRef CAS.
-
M. Born and E. Wolf, Principles of Optics: Electromagnetic Theory of Propagation, Interference and Diffraction of Light, Cambridge University Press, London, 7th edn, 1999 Search PubMed.
- J. Szetjli, Chem. Rev., 1998, 98, 1743–1753 CrossRef.
-
D. P. Shoemaker and C. W. Garland, Experiments in Physical Chemistry, McGraw-Hill, New York, 1967, pp. 131–138 Search PubMed.
Footnote |
† Electronic supplementary information (ESI) available. See DOI: 10.1039/c5nj02179k |
|
This journal is © The Royal Society of Chemistry and the Centre National de la Recherche Scientifique 2016 |
Click here to see how this site uses Cookies. View our privacy policy here.