DOI:
10.1039/C5NJ02118A
(Paper)
New J. Chem., 2016,
40, 641-650
Highly selective colorimetric and reversible fluorometric turn-off sensors based on the pyrimidine derivative: mimicking logic gate operation and potential applications†
Received
(in Montpellier, France)
9th August 2015
, Accepted 12th November 2015
First published on 16th November 2015
Abstract
Two novel pyrimidine based 5-(2-hydroxybenzylideneamino)-6-amino-2-mercaptopyrimidin-4-ol (S1) and 5-(3-nitrobenzylideneamino)-6-amino-2-mercaptopyrimidin-4-ol (S2) receptors have been synthesized and characterized by various techniques. Both receptors showed 2
:
1 complexation stoichiometry with Ni(II) having binding constants 2.9 × 106 (S1) and 2.1 × 106 (S2) calculated by the Job's plot based on the UV-Vis absorption studies. The binding stoichiometry was also supported by the ESI mass spectra and NMR titration. The addition of Ni(II) to both chemosensors, S1 and S2 leads to the fluorescence quenching of S–Ni(II), forming an off sensing type system with the limits of detection 33 μM and 48 μM respectively. It was observed that S1 and S2 achieved electrochemical changes in reduction and oxidation potentials after the addition of the nickel metal ion. DFT calculations have revealed that the energy gap between the HOMO and LUMO of S1 and S2 has significantly decreased upon coordination with Ni(II) in the gas phase.
Introduction
In recent years a great deal of research has been attracted towards the detection of transition metal ions due to their environmental and biological importance. Nickel is an essential trace element for supporting life, such as respiration, metabolism and biosynthesis.1 Nickel compounds have many industrial and commercial uses, and the progress of industrialization has led to increased emission of pollutants into ecosystems.2 It has been used in industrial applications such as stainless steel, jewellery, electroplating, Ni–Cd batteries, machinery, tools and as a precursor for a catalyst.3 However a higher uptake of nickel can cause a variety of pathological effects on the human body as in a form of lung cancer, prostate cancer, larynx cancer, lung embolism, asthma and chronic bronchitis, pneumonitis and dermatitis.4 In the various literature, several review studies have been reported on the recognition of the fluorogenic unit which is responsible for the selectivity and binding efficiency of the chemosensor. Several N-heterocycles such as triazole, thiazole, pyridine, pyrrole, quinoline, or imidazole and their derivatives are utilized as recognition units. For example, pyrimidine derivatives are appropriate objects for using as excellent sensing materials and also have a variety of biological and medicinal applications. The efficiency of pyrimidine derivatives to form both the coordination and hydrogen bond makes them assuring to the use as sensing probes. The simplicity and cost-effectiveness are always a matter of concern for an optical chemosensor. Thus, here we report the facile and inexpensive synthesis of two novel pyrimidine based colorimetric and fluorogenic receptors which have been obtained in a pure powdered form without any column chromatographic purification.
In order to quantify nickel ions, several analytical techniques of high cost such as inductively coupled plasma optical emission spectrometry,5 coated wire ion selective electrode,6 microwave induced plasma,7 electrothermal atomic absorption spectrometry,8 and spectrophotometry9 have been used. Many of these pretreatment techniques are, however complicated, time consuming and not suitable for quick response. In recent time the most frequently utilized method is optical sensing, because of low cost and simple handling. There are several mechanisms which support the fluorescence behavior for cations and anions as internal charge transfer (ICT), chelation enhanced fluorescence (CHEF), photoinduced electron transfer (PET) and deprotonation mechanisms.
To design an efficient colorimetric sensor with open coordination sites which has high selectivity and sensitivity for the Ni(II) ion, two receptors have been synthesized by the condensation method. A significant color change of receptors solution occurred after addition of the Ni(II) ion from light yellow to dark yellow with a red shift. These two receptors S1 and S2 have shown fluorescence quenching after the recognition with the Ni(II) ion, caused by the paramagnetic nature of the Ni(II) ion. Further, we have been investigating the electrochemical behavior of the two receptors by the cyclic voltammetry experiment. In the recognition, 2
:
1 stoichiometry was corroborated by the Job’s plot as well as theoretical aspects. The reversible behavior of chemical receptors is presented using the logic gate application.
Results and discussion
Two Schiff bases were synthesized in good yield by employing a simple synthetic route of condensation in dry methanol. 4,5-Diamino-6-hydroxy-2-mercaptopyrimidine was used as a common amine with a different aldehyde (Scheme 1).
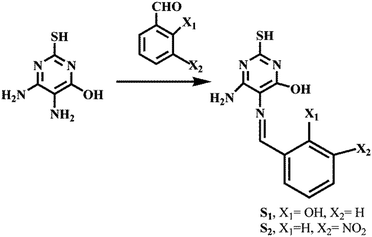 |
| Scheme 1 Synthesis of receptors S1 and S2. | |
In order to investigate the recognition ability of both receptors with different metal ions, the preliminary studies have been executed. UV-Vis studies demonstrated the affinity of S1 and S2 towards the Ni(II) ion over various metal ions, and upon the addition of nickel acetate, a sudden color change from light yellow to dark yellow was observed (Fig. S1, ESI†). Thus we have conducted colorimetric studies by using equimolar solutions (1.0 × 10−4 M) of S1, S2 and metal ions in DMF. The sudden color change of the receptors was most probably due to the recognition or deprotonation of –OH groups upon the addition of the Ni(II) ion.10
UV-Vis analysis
S1 and S2 display a more intense color change in the presence of Ni(II) on the behalf of strong metal–receptor interaction, whereas no significant changes were observed with Mg(II), Ca(II), Pb(II), Hg(II), Fe(II), Sr(II), Mn(II), Cu(II), Mo(II), Ag(I), Co(II), Zn(II) and Cd(II) ions. Upon the addition of Ni(II), the UV-Vis spectra of S1 and S2 showed a red-shift that indicates a charge transfer interaction between the receptor moiety and the nickel metal ion (Fig. 1). Binding stability was examined by monitoring over the time, the colorimetric changes were observed to measure the strength of interaction between the receptor and the metal ion.11 Usually, it has been observed that chemosensors have long response time, but in case of these receptors the binding process with the Ni(II) ion is found to very fast i.e. less than 13 s and remained quite stable.
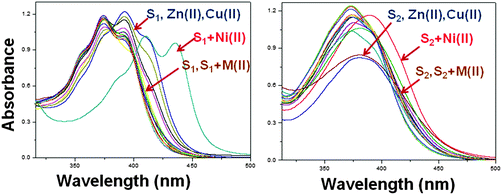 |
| Fig. 1 UV-Vis studies of S1 and S2 (4 × 10−5 M) with all metal ions. | |
It can be observed that the colorimetric properties of S–Ni(II) are also stable with time (Fig. S2, ESI†).
Stoichiometry and binding sites
To ensure the binding sites of both receptors response, the binding stoichiometry of S1 and S2 was calculated from the Job's plot on the basis of UV-Vis absorption studies.12 Equimolar solutions (4 × 10−5 M) of receptors S1, S2 and the nickel metal ion were prepared in DMF. To determine the binding stoichiometry of S + Ni(II), we carried out absorption titration experiments in the presence of varying mole-fractions of Ni(II) in DMF. The stoichiometry was established by the Job's plot between the mole fraction and absorption maximum changes at 435 nm for S1 and 409 nm for S2 (Fig. 2). Upon the addition of 0–6 mL of Ni(II) (with the equal span of 0.5 mL), the absorption maxima of S1 and S2 were quenched at 392 nm (S1) and 374 nm (S2) and a new band appeared at 435 and 409 nm, respectively. Maximum absorbance was obtained at a mole fraction of 0.66, indicating the stoichiometry of S + Ni(II) was 2
:
1. S1 has more binding ability in comparison to S2, the binding constants calculated by the Job's plot were 2.9 × 106 for S1 and 2.1 × 106 for S2.
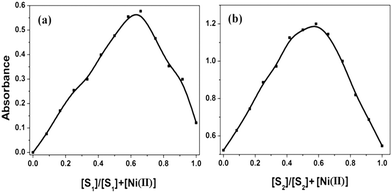 |
| Fig. 2 Job's plot of equimolar concentration (4.0 × 10−5 M) of receptors S1 (a) and S2 (b) with the Ni(II) ion. | |
The stability constants were calculated by the following expression:13
|  | (2) |
| 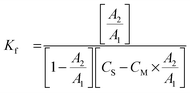 | (3) |
where,
CS is the receptor concentration,
CM is the metal concentration,
A2 is the actual absorbance, and
A1 is the absorbance at the break point.
The Job's curve for both receptors is exhibited in Fig. 2. The isosbestic points at 402 nm (S1) and 312 nm and 381 nm (S2) clearly indicate that there was a new complex formation with a certain stoichiometry ratio between the host and the guest via internal charge transfer14 (Fig. 3a and b).
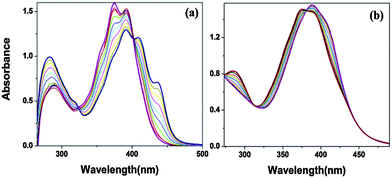 |
| Fig. 3 UV-Vis titration spectra (a and b) of receptor S1 and S2 (5 × 10−5 M) upon addition of the Ni(II) ion in the DMF solvent. (0, 0.3, 0.6, 0.9, 1.2, 1.5, 1.8, 2.1, 2.4, 2.7, 3) equivalents of the Ni(II) ion. | |
Similar to the Job's plot, the binding stoichiometry was further supported by the ESI mass spectrum and 1H NMR titration along with binding site confirmation as shown in Fig. 4. The ESI mass spectrum of a mixture of receptors and Ni(CH3COO)2·4H2O revealed the formation of the S–Ni(II) complex through the coordination bond, which showed a sharp peak at m/zcalcd = 581.92 calculated, found 581.25 for [2S1–Ni(II) + H] and m/zcalcd = 639.50 calculated, found 639.24 for [2S2–Ni(II) + H] (Fig. S3, ESI†). From the NMR studies, we have investigated the interactions between the receptors and the nickel ion upon the addition of different equivalents of the nickel ion (0, 0.3, 0.5, 0.8) to receptors S1 and S2 in DMSO-d6. Upon the addition of 0.5 equiv., the hydroxyl group of the amine moiety is completely disappeared along with the downfield shift of the imine proton from 9.75 ppm to 9.83 ppm (S1) and 9.76 ppm to 9.92 ppm (S2) through diamagnetic deshielding indicating that there is a new complex formation (2
:
1) between the –OH group, the imine CH
N moiety of pyrimidine and the nickel ion. There was no significant change on the SH proton and the rest of the aromatic region protons. It was observed that further addition (more than 0.5 equiv.) of the nickel ion did not change the 1H NMR spectra15 (Fig. S4, ESI†). The both S–Ni(II) complex optimized as square planar geometry in the gas phase, which shows the diamagnetic nature of the nickel metal ion, cause of deshielding of protons. The NMR titration evaluated that Ni(II) is coordinating through the nitrogen and oxygen atoms of the pyrimidine moiety in the S–Ni(II) complex.
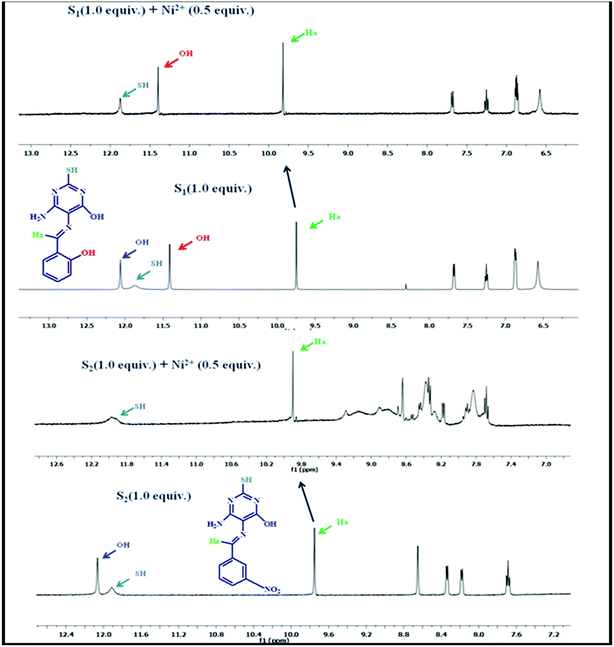 |
| Fig. 4
1H NMR titration of S1 and S2 with the Ni(II) ion. | |
Fluorescence studies
Similar to the absorption study of these two receptors, we have also investigated the photophysical properties of receptors in DMF. To scrutinize the practical ability of both S1 and S2, the competitive binding studies with other metal ions have been introduced (Fig. 5 and 6). It has been observed that other metal ions show minimum disruption in the fluorescence spectra of both compounds. Fluorescence emission of S1 and S2 (5 × 10−6 M) did not show any appreciable interference after the addition of different metal ions. S1 showed the maximum excitation at 392 nm and emission at 520 nm while S2 showed the maximum excitation at 374 nm and emission at 441 nm which shows a large stoke shift.
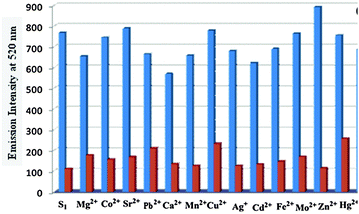 |
| Fig. 5 Interference study with different metal ion at 520 nm, S1 + metal ions (blue bar) and S1 + metal ions + Ni(II) (red bar). | |
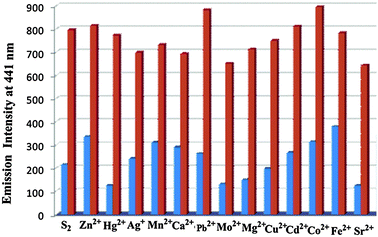 |
| Fig. 6 Interference study with different metal ions at 441 nm, S2 + metal ions (red bar) and S2 + metal ions + Ni(II) (blue bar). | |
After the gradual addition of nickel ion concentration, the fluorescence quenching phenomenon has occurred16 (Fig. 7 and 8). The electron or energy transfer could be a possible mechanism for remarkable quenching with the Ni(II) ion. The Stern–Volmer plot clearly indicates the static nature of quenching. The energy transfer mechanism provides a pathway for the contribution to the nonradiative deactivation of the excited state to a significant extent.17
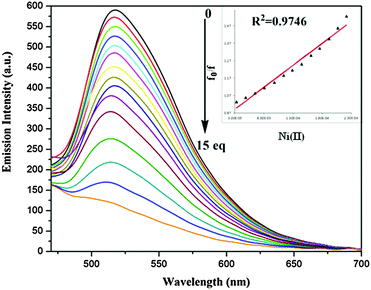 |
| Fig. 7 Emission spectra of S1 (5.0 × 10−6 M) in the presence of varying concentration of Ni(II) at λex 392 nm (inset – change in emission intensity with number of equivalents of Ni(II)). | |
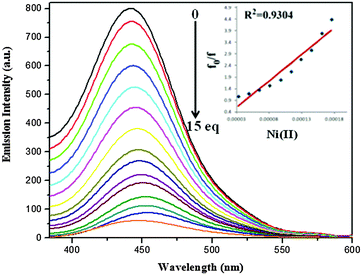 |
| Fig. 8 Emission spectra of S2 (5.0 × 10−6 M) in the presence of varying concentration of Ni(II) at λex 374 nm (inset – change in emission intensity with number of equivalents of Ni(II)). | |
For the calculation of the limit of detection, fluorescence intensity of S1 and S2 at each concentration of Ni(II) added, normalized between the maximum fluorescence intensity, found at zero equiv. of Ni(II), and the minimum fluorescence intensity, found at 5 × 10−6 M of Ni(II). The limit of detection has been calculated by using the intercept of a plot between (Imax − I)/(Imax − Imin) and log[Ni(II)]18 and found to be 33 and 48 μM for S1 and S2, respectively (Fig. S5 (ESI†)).
Optimization of analytical conditions
The potential selectivity of both receptors was determined as a function of pH over a wide pH range (1–14). A 25 mL stock solution (4 × 10−5 M) of both receptors was prepared in DMF as the receptors are sparingly soluble in water. The pH was adjusted with the addition of aqueous 0.5 M HCl and 1 M NaOH. The optical studies of both S1–Ni(II) and S2–Ni(II) with respect to different pH affirmed that both receptors predominantly work between the pH ranges of 5–11 and 6–12, respectively (Fig. S6, ESI†).
At low pH (1–4) receptors did not show any changes with the Ni metal ion in absorption studies. On increasing pH 12–14 the selectivity of sensors was decreased. The effect of pH on the emission spectra of S1 and S2 (5 μM) was examined in the absence and presence (5 μM) of Ni(II) (Fig. 9). At pH < 5, the fluorescence intensity of both receptors was relatively low owing to the protonation, at the high pH > 12, OH− will compete with receptors to bind Ni(II), which influenced the detection of Ni(II). S1 and S2 chemosensors work well in wide range of 5–11 and 6–11, respectively for the detection of the Ni(II) ion. That proves the utility of both receptors to work as a sensor under physiological pH conditions. The effect of the water content on absorption studies as well as fluorescence intensity of both receptors (5 μM) has been studied. It is observed that the selectivity and sensitivity of both receptors have been diminished on increasing the content of water. Receptor S1 has shown selectivity with the nickel ion up to 40% water while S2 has shown upto 20% water (Fig. S7, ESI†). The sensitivity of the receptors has gradually decreased after their respective percentage. Thus the receptor S1 has more real-purpose applicability than S2.
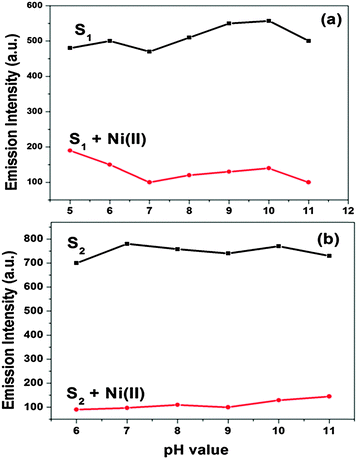 |
| Fig. 9 Effect of pH on the emission spectra of S1 (a) and S2 (b) in DMF without and with Ni(II). | |
Electrochemical behavior of sensors
Cyclic voltammetry experiment.
To investigate the redox properties of both receptors and their complexation with Ni(II), the cyclic voltammograms of receptors S1, S2 and S–Ni(II) were recorded in DMF solution containing 0.1 M TBAP. The cyclic voltammogram of S1 displayed two reversible oxidation peaks at 0.91 V and 1.33 V and a non reversible reduction peak at −0.58 V whereas upon the addition of the Ni(II) ion there was not any change in the oxidation peak, but two irreversible reduction peaks at −1.33 V and −1.73 V appeared which correspond to Ni(II) ↔ Ni(I) (Fig. 10). On the other hand S2 showed remarkable change after the complexation with the nickel ion. S2 showed two oxidation peaks at 1.17 V and 0.96 V and two irreversible reduction peaks at −0.44 V and −0.97 V. Upon the addition of the Ni(II) ion one oxidation peak shifted positively and the two irreversible peaks shifted to −0.92 and −1.5 which showed the complexation with the nickel ion (Fig. 11 and Table 1). It is well observed that the redox potential of S2 shift positively due to the electron-withdrawing substituents. It may due to the electron withdrawing influence of the additional nitro group.19
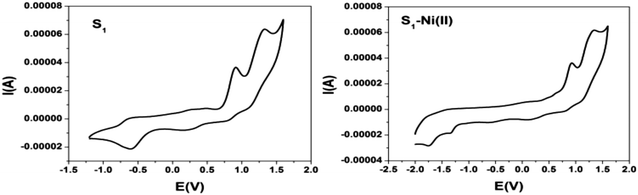 |
| Fig. 10 Cyclic voltammograms of S1 and the S1–Ni(II) complex in DMF solution containing 0.1 M TBAP at a scan rate of 100 mV s−1. | |
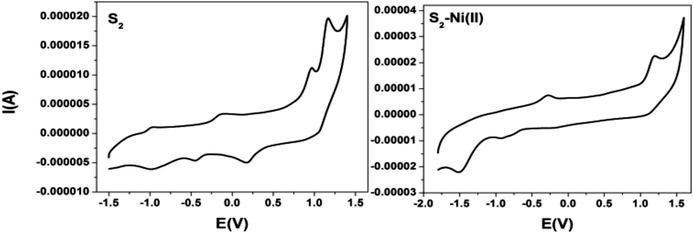 |
| Fig. 11 Cyclic voltammograms of S2 and the S2–Ni(II) complex in DMF solution containing 0.1 M TBAP at a scan rate of 100 mV s−1. | |
Table 1 The electrochemical behaviour of the receptor S1, S1–Ni(II) and S2, S2–Ni(II) complexes
Sample |
I oxid. (V) |
II oxid. (V) |
I red. (V) |
II red. (V) |
Ligand S1 |
0.91 |
1.33 |
−0.58 |
— |
S1–Ni(II) |
0.92 |
1.33 |
−1.33 |
−1.73 |
Ligand S2 |
0.96 |
1.17 |
−0.44 |
−0.97 |
S2–Ni(II) |
1.19 |
— |
−0.92 |
−1.5 |
Theoretical calculation
In order to understand the mechanism of all obtained experimental results, a DFT study20 was performed for molecular geometry and frequency calculation (Fig. S8, ESI†). The energy band gap between the HOMO–LUMO of S1 (3.79 eV), S1-Ni(II) (2.74 eV) and S2 (3.39 eV), S2-Ni(II) (2.50 eV) has been decreased, i.e. 1.05 eV and 0.89 eV, respectively (Fig. 13).
This represented a supportable mechanism for metal ion complexation according to the proposed coordination.21 The HOMO → LUMO excitation was observed to favour the ligand to metal charge transfer (LMCT) which contributes to lowest energy excitation. It was notified that the energy decrease in the LUMO level was remarkable than that in the HOMO level, indicating that the LUMO was more stabilized than the HOMO. Attributed to the importance of DFT studies, it was evaluated that apart from the band gap the electron density in S1 and S2 was localized on the atoms of the whole molecule.
Upon the complexation with the Ni(II) ion, the density was more located on the S–Ni(II) complex. This result suggested that the coordination of Ni(II) with the pyrimidine moiety promoted internal charge transfer through increasing the electron donating ability of the coordinating nitrogen and oxygen of the pyrimidine moiety. Moreover the data of IR frequency of S1 and S2 had the nearest spectrum to the experimental results (Fig. 12).
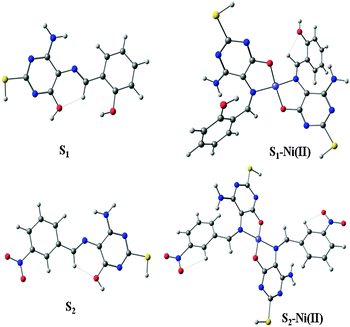 |
| Fig. 12 Optimized geometries of S1, S1–Ni(II) and S2, S2–Ni(II). | |
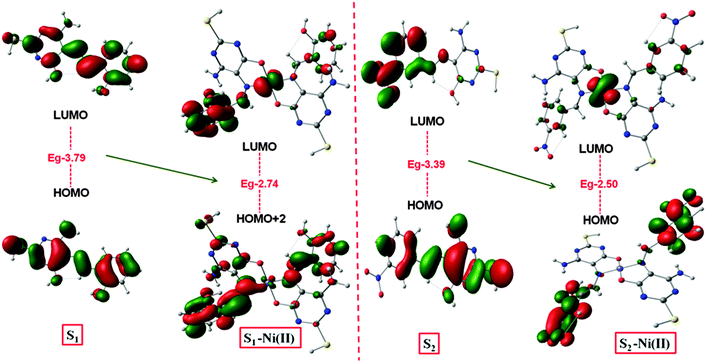 |
| Fig. 13 Pictorial view of frontier molecular orbitals of S1, S1–Ni(II) and of S2, S2–Ni(II). | |
The reversibility behavior of S1 and S2
To explain the coordination mechanism of Ni(II) with receptors S1 and S2, a fluorescence analysis has been done using EDTA. The reversibility test was performed using the S–Ni(II) solution (5 μM) with the disodium salt of ethylene diamine tetra-acetic acid (EDTA) (20 μM) in H2O. The introduction of EDTA to S–Ni(II) solution could restore the yellowish color of receptors itself due to the strong binding ability of EDTA toward Ni(II). Both sensors were found to be reversible to their original state during the addition of EDTA and can be reused upto 3 cycles as demonstrated in Fig. 14. Both receptors have shown the quenching behavior with the addition of the nickel metal ion but as the EDTA is added to the complex, the fluorescence intensity has been increased which clearly indicates that EDTA inhibits the interaction between the receptor and the metal ion.22 Further addition of Ni(II) to the solution again the quenching phenomena has been occurred. It has been illustrated that Ni(II) displayed a significant fluorescence change by showing the OFF behavior through the complex formation. The addition of an excess amount of EDTA to the mixture of receptors S1–Ni(II) and S2–Ni(II) results in the enhancement of the fluorescence intensity and hence acts as an ON switch (Fig. 14 and 15). This “ON–OFF–ON” switching process could be repeated several times with the meager loss of fluorescence efficiency.
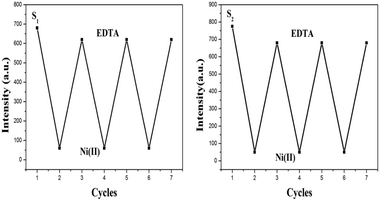 |
| Fig. 14 Reversible changes in fluorescence intensity of S1 and S2 (5.0 × 10−6 M) at 520 nm and 441 nm respectively, after the sequential addition of Ni(II) and EDTA. | |
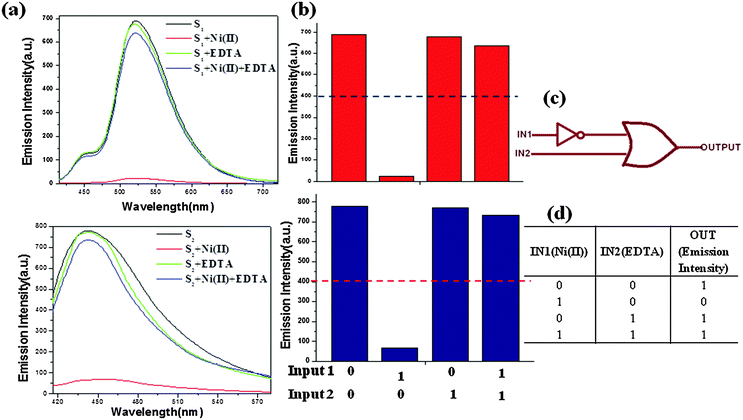 |
| Fig. 15 (a) Fluorescence spectra of S1 and S2 (5 × 10−6 M) for an emission wavelength of 520 nm and 441 nm respectively under four different input conditions; (b) changes in the fluorescence intensity at 520 nm (S1) and 441 nm (S2) under four different input conditions; (c) IMPLICATION logic scheme; (d) IMP truth table. IN1 and IN2 represent input Ni(II) ions and input EDTA, respectively; OUT is represented by their respective fluorescence intensities. | |
The repeated behavior of S1 and S2 by fluorescence change using EDTA well explained the reversible behavior of both sensors which supports the electron transport mechanism for quenching.
Logic gate application
In recent days, researchers have shown considerable interest towards molecular computing due to its broad applications in molecular switches, keypad devices, biosensing, diagnostics, functional materials, biochemical systems23etc. As discussed above the reversibility behavior of both receptors, it can be represented by the combinational logic circuit. In this concrete system (Fig. 15), the ON state (output = 1) is defined as the strong fluorescence at 520 nm (S1) and 441 nm (S2), whereas the OFF state (output = 0) corresponds to the weak fluorescence. The presence and absence of two chemical inputs IN1 Ni(II) and IN2 EDTA can be specified as ‘1’ and‘0’ states. The threshold value of fluorescence intensity is stated to be 400 nm at output in case of both receptors. However, the enhanced fluorescence of both receptors above the threshold level was ascertained in the absence (0, 0) and presence of both the inputs (1, 1) and also EDTA alone (1, 0). Therefore, by observing the fluorescence of both receptors with the two-inputs (IN1 Ni(II) and IN2 EDTA), an IMPLICATION logic gate can be established at the molecular level (Fig. 15).
Analytical application
Analysis of nickel in waste and river water samples.
The receptor S1 was successfully applied for the determination of the nickel metal ion in waste water samples.24 The water samples were collected from tap water of Roorkee and River Ganga from Roorkee and Haridwar. Absorption experiments were performed using 3 mL of S1 (4 × 10−5 M in DMF) and 40 μL of the nickel metal ion (4 × 10−5 M) in corresponding water samples.
All water samples were used without pretreatment, and a known amount of nickel was added. The recovery of the receptor S1 was obtained for different spiked water samples, calculated as 93.75–97.75% which was indicating that the application of the receptor S1 for the detection of Ni(II) in real water samples was quite doable. Table S1 (ESI†) shows that the results analyzed by the direct UV-Vis studies are found to be close enough with those calculated by the atomic absorption spectroscopy (AAS) method with an appropriate amount of Ni(II) added and justify the potential of the optical sensor for routine measurements.
Conclusion
Here we have synthesized two novel efficient colorimetric receptors via the facile condensation process and characterized by various techniques. Upon addition of different metal ions, both receptors show a remarkable change in color (yellow to dark yellow) using the Ni(II) metal ion which makes it suitable for easy naked eye detection. The 2
:
1 stoichiometry of S1 and S2 was confirmed by the Job's plot, ESI mass and 1H NMR titration. The association constant values of S1 + Ni(II) and S2 + Ni(II) were calculated by the Job's plot as 2.9 × 106 and 2.1 × 106 respectively. More interestingly, the fluorescence titration revealed that S1 and S2 behave as good fluorescence quenchers, which support the electron transfer and nonradiative decay of the excited state mechanism. The limit of detection has been calculated to be 33 μM for S1 + Ni(II) and 48 μM for S2 + Ni(II) that indicates S1 is superior than S2. Electroanalytical studies have been investigated by the cyclic voltammetric experiment. Theoretical calculations suggest the complexation of both receptors with the Ni(II) ion by the energy gap difference between the HOMO and the LUMO. Also, both receptors can work as reversible sensors with EDTA on the basis of fluorescence titration. We are confident that both receptors are useful for practical applications in other sensing fields such as biological and environmental research in the future.
Experimental details
Reagent
4,5-Diamino-6-hydroxy-2-mercaptopyrimidine, 3-nitro benzaldehyde and salicylaldehyde were purchased from Sigma-Aldrich. All the salts of metals used were of analytical grade and used directly (without any further purification).
Instrumentation and methods
The UV-Vis spectra were recorded on a Shimadzu, UV-3600 double beam spectrophotometer using a 10 mm path length silica cell. The FT-IR spectra were obtained using a Perkin Elmer FT-IR 1000 spectrophotometer as films between KBr. Elemental analysis was carried out on an Elementar model Vario EL-III. The 1H-NMR and 13C-NMR spectra of S1 and S2 in DMSO-d6 were recorded using Bruker AVANCE, 500.13 MHz spectrometer and Jeol ECX 400 MHz. The emission spectra were obtained from RF-5301PC using a 3 cm standard quartz cell. The LC-HRMS spectra were recorded on an Agilent spectrometer micrOTOF-Q II 10330 in a positive mode using HPLC methanol as the solvent. Cyclic voltammetric studies were performed under the nitrogen atmosphere at 298 K on a CHI760E electroanalyser in DMF using 0.1 M tetrabutylammonium perchlorate (TBAP) as a supporting electrolyte. The working, reference and auxiliary electrodes were glassy carbon, Ag/AgCl and Pt wire, respectively, within the potential range from +1.5000 V to −1.5000 V at a scan rate of 0.1 V s−1. All solutions should be nitrogen purged before the experiment. Geometric optimization was performed using the B3LYP functional with 6-31+g(d,p) basis sets as for receptors and with the metal ion organized in the Gaussian 09 W programme in the gas phase.
Synthesis and characterization of S1 and S2
Synthesis of S1.
The proposed Schiff base S1 was synthesized by stirring with a reflux of 4,5-diamino-6-hydroxy-2-mercaptopyrimidine (0.001 M) with salicylaldehyde (0.001 M) for 24 hours and after that a yellow color precipitate was observed.
Yield: (81%). IR data (KBr, νmax/cm−1): O–H: 3434, N–H 3302, Ar–H: 3161, C
N: 1625, C
C: 1562, C–N: 1413, C–O: 1193. Anal. calc. for C11H10N4O2S: C, 50.37; H, 3.84; N, 21.36; O, 12.20; S, 12.23, found: C, 49.98; H, 3.29; N, 20.89; O, 12.45; S, 12.14. UV-Visible (DMF, λmax/nm) 392 nm (n–π*), 290 nm (π–π*), 1H-NMR (DMSO-d6, 500 MHz, δ/ppm): 12.6 (s, 1H), 11.87 (s, 1H), 11.41 (s, 1H), 9.75 (s, 1H), 7.68 (d, 1H), 7.25 (d, 1H), 6.88 (m, 2H), 6.57 (s, 2H). 13C-NMR (S1, DMSO-d6, 100 MHz, δ/ppm) 171, 158, 157, 155, 151, 136, 131, 130, 122, 119, 116. (Fig. S9 to S12, ESI†).
Synthesis of S2.
To synthesize S2, we took 0.01 M of 4,5-diamino-6-hydroxy-2-mercaptopyrimidine and 0.01 M of 3-nitro benzaldehyde in methanol solution and after that refluxed this solution with stirring for 10 hours till whole amount of amine was consumed, a yellow color precipitate was formed.
Yield: (86%). IR data (KBr, νmax/cm−1): O–H: 3452, N–H 3361, Ar–H: 3327, C
N: 1614, C
C: 1562, C–N: 1416, C–O: 1195. Anal. calc. for C11H9N5O3S: C, 45.36; H, 3.11; N, 24.04; O, 16.48; S, 11.01, found: C, 44.83; H, 3.05; N, 24.89; O, 15.95; S, 11.14. UV-Visible (DMF, λmax/nm) 374 nm (n–π*) and 286 nm (π–π*), 1H-NMR spectra: NMR (DMSO-d6, 500 MHz, δ/ppm): 12.06 (s, 1H), 11.92 (s, 1H), 9.76 (s, 1H), 8.65 (s, 1H), 8.33 (d, 1H), 8.18 (d, 1H), 7.68 (t, 1H), 6.98 (s, 2H). 13C-NMR (S2, DMSO-d6, 100 MHz, δ/ppm) 172, 157, 153, 149, 148, 140, 134, 130, 124, 121, 102 (Fig. S9 to S12, ESI†).
Acknowledgements
NG is thankful to the University of Grant Commission (UGC), New Delhi, India, for financial support to undertake this work. NG is also grateful to Miss Pinky Yadav for her valuable discussion.
References
- S. B. Mulrooney and R. P. Hausinger, Microbiol. Rev., 2003, 27, 239–261 CAS.
- M. Bansal, D. Singh, V. K. Garg and P. Rose, World Acad. Sci. Eng. Technol., 2009, 3, 3–25 Search PubMed.
- G. Fu, Z. Hu, L. Xie, X. Jin, Y. Xie, Y. Wang, Z. Zhang, Y. Yang and H. Wu, Int. J. Electrochem. Sci., 2009, 4, 1052–1062 CAS.
- M. Cempel and G. Nikel, Pol. J. Environ. Stud., 2006, 3, 375–382 Search PubMed.
- E. A. Takara, S. D. Pasini-Cabello, S. Cerutti, J. A. Gasquez and L. D. J. Martinez, J. Pharm. Biomed. Anal., 2005, 39, 735–739 CrossRef CAS PubMed.
-
(a) M. Mazloum, M. S. Niassary and M. K. Amini, Sens. Actuators, B, 2002, 82, 259–264 CrossRef CAS;
(b) M. Giorgetti, E. Scavetta, M. Berrettoni and D. Tonelli, Analyst, 2001, 126, 2168–2171 RSC.
- K. Jankowski, J. Yao, K. Kasiura, A. Jackowska and A. Sieradzka, Spectrochim. Acta, Part B, 2005, 60, 369–375 CrossRef.
- D. Zendelovska, G. Pavlovska, K. Cundeva and T. Stafilov, Talanta, 2001, 54, 139–146 CrossRef CAS PubMed.
- A. Sunil and S. J. Rao, J. Anal. Chem., 2015, 70, 154–158 CrossRef CAS.
-
(a) S. Goswami, S. Chakraborty, S. Paul, S. Halder and A. C. Maity, Tetrahedron Lett., 2013, 54, 5075–5077 CrossRef CAS;
(b) D. Peralta-Domínguez, M. Rodríguez, G. Ramos-Ortíz, J. L. Maldonadoa, M. A. Meneses-Navaa, O. Barbosa-García, R. Santillan and N. Farfán, Sens. Actuators, B, 2015, 207, 511–517 CrossRef.
-
(a) L. Wang, D. Ye and D. Cao, Spectrochim. Acta, Part A, 2012, 90, 40–44 CrossRef PubMed;
(b) J. W. Nugent, H. Lee, H. Lee, J. H. Reibenspies and R. D. Hancock, Inorg. Chem., 2014, 53, 9014–9026 CrossRef CAS PubMed;
(c) D. Singhal, N. Gupta and A. K. Singh, RSC Adv., 2015, 5, 65731–65738 RSC.
- X. Liu, Q. Lin, T. Wei and Y. Zhang, New J. Chem., 2014, 38, 1418–1423 RSC.
-
(a) N. Gupta, A. K. Singh, S. Bhardwaj and D. Singhal, Electroanalysis, 2015, 27, 1165–1175 CrossRef;
(b) D. Singhal, A. K. Singh and A. Upadhyay, Mater. Sci. Eng., C, 2014, 45, 216–224 CrossRef CAS PubMed.
- T. Ghosh, B. G. Maiya and A. Samanta, Dalton Trans., 2006, 795–801 RSC.
- J. Liu, X. He, J. Zhang, T. He, L. Huang, J. Shen, D. Li, H. Qiu and S. Yin, Sens. Actuators, B, 2015, 208, 538–545 CrossRef CAS.
-
(a) H. Zhu, J. Fan, B. Wang and X. Peng, Chem. Soc. Rev., 2015, 44, 4337–4366 RSC;
(b) N. Aksuner, E. Hendena, I. Yilmaz and A. Cukurovali, Sens. Actuators, B, 2012, 166, 269–274 CrossRef;
(c) L. Fabbrizzi, M. Licchelli, P. Pallavicini, D. Sacchi and A. Taglietti, Analyst, 1996, 121, 1763–1768 RSC;
(d) Z. Li, W. Zhao, X. Li, Y. Zhu, C. Liu, L. Wang, M. Yu, L. Wei, M. Tang and H. Zhang, Inorg. Chem., 2012, 51, 12444–12449 CrossRef CAS PubMed;
(e) M. Dutta and D. Das, Trends Anal. Chem., 2012, 32, 113–132 CrossRef CAS.
-
(a) S. Sangeetha, G. Sathyaraj, D. Muthamilselvan, V. G. Vaidyanathan and B. U. Nair, Dalton Trans., 2012, 41, 5769–5773 RSC;
(b) S. Blanchard, F. Neese, E. Bothe, E. Bill, T. Weyhermuller and K. Wieghardt, Inorg. Chem., 2005, 44, 3636–3656 CrossRef CAS PubMed;
(c) Z. Li, W. Zhao, X. Li, Y. Zhu, C. Liu, L. Wang, M. Yu, L. Wei, M. Tang and H. Zhang, Inorg. Chem., 2012, 51, 12444–12449 CrossRef CAS PubMed;
(d) H. C. Clark and R. J. O'Brien, Can. J. Chem., 1961, 39, 1030–1036 CrossRef CAS.
- A. Caballero, R. Martínez, V. Lloveras, I. Ratera, J. Vidal-Gancedo, K. Wurst, A. Tárraga, P. Molina and J. Veciana, J. Am. Chem. Soc., 2005, 127, 15666–15667 CrossRef CAS PubMed.
-
(a) K. Ghosh, V. Mohan, P. Kumar, S. W. Ng and E. R. T. Tiekink, Inorg. Chim. Acta, 2014, 416, 76–84 CrossRef CAS;
(b) J. Jee, M. C. Chang and C. Kwak, Inorg. Chem. Commun., 2004, 7, 614–617 CrossRef CAS;
(c) C. J. Dhanaraj and J. Johnson, Spectrochim. Acta, Part A, 2014, 118, 624–631 CrossRef PubMed;
(d) C. D. Pieve and B. Spingler, Inorg. Chim. Acta, 2012, 380, 230–235 CrossRef;
(e) A. Biswas, M. G. B. Drew and A. Ghosh, Polyhedron, 2010, 29, 1029–1034 CrossRef CAS;
(f) B. Shafaatian, A. Soleymanpour, N. K. Oskouei, B. Notash and S. A. Rezvani, Spectrochim. Acta, Part A, 2014, 128, 363–369 CrossRef CAS PubMed.
-
H. B. Schlegel, G. E. Scuseria, M. A. Robb, J. R. Cheeseman, G. Scalmani, V. Barone, B. Mennucci, G. A. Petersson, H. Nakatsuji, M. Caricato, X. Li, H. P. Hratchian, A. F. Izmaylov, J. Bloino, G. Zheng, J. L. Sonnenberg, M. Hada, M. Ehara, K. Toyota, R. Fukuda, J. Hasegawa, M. Ishida, T. Nakajima, Y. Honda, O. Kitao, H. Nakai, T. Vreven, J. A. Montgomery, Jr., J. E. Peralta, F. Ogliaro, M. Bearpark, J. J. Heyd, E. Brothers, K. N. Kudin, V. N. Staroverov, R. Kobayashi, J. Normand, K. Raghavachari, A. Rendell, J. C. Burant, S. S. Iyengar, J. Tomasi, M. Cossi, N. Rega, J. M. Millam, M. Klene, J. E. Knox, J. B. Cross, V. Bakken, C. Adamo, J. Jaramillo, R. Gomperts, R. E. Stratmann, O. Yazyev, A. J. Austin, R. Cammi, C. Pomelli, J. W. Ochterski, R. L. Martin, K. Morokuma, V. G. Zakrzewski, G. A. Voth, P. Salvador, J. J. Dannenberg, S. Dapprich, A. D. Daniels, Ö. Farkas, J. B. Foresman, J. V. Ortiz, J. Cioslowski and D. J. Fox, Gaussian 09, Revision A.02, Gaussian, Inc., Wallingford, CT, 2009 Search PubMed.
- B. B. Petkovi, M. Milci, D. Stankovi, I. Stamboli, D. Manojlovi, V. M. Jovanovi and S. P. Sovilj, Electrochim. Acta, 2013, 89, 680–687 CrossRef.
- V. D. Miletic, A. Meetsma, P. J. van Koningsbruggen and Z. D. Matovic, Inorg. Chim. Acta, 2009, 12, 720–723 CAS.
-
(a) Z. Dong, Y. Guo, X. Tian and J. Ma, J. Lumin., 2013, 134, 635–639 CrossRef CAS;
(b) N. Kaur and P. Alreja, Tetrahedron Lett., 2015, 56, 182–186 CrossRef CAS;
(c) L. Huang, J. Zhang, X. Yu, Y. Ma, T. Huang, X. Shen, H. Qiu, X. He and S. Yin, Spectrochim. Acta, Part A, 2015, 145, 25–32 CrossRef CAS PubMed;
(d) C. Zhang, C. Sun, Y. Lu, J. Wang, X. He, J. Lu, S. Yin and H. Qiu, Spectrochim. Acta, Part A, 2015, 150, 731–736 CrossRef CAS PubMed.
- M. R. Ganjali, M. Hosseini, M. Motalebi, M. Sedaghat, F. Mizani, F. Faridbod and P. Norouzi, Spectrochim. Acta, Part A, 2015, 140, 283–287 CrossRef CAS PubMed.
Footnote |
† Electronic supplementary information (ESI) available. See DOI: 10.1039/c5nj02118a |
|
This journal is © The Royal Society of Chemistry and the Centre National de la Recherche Scientifique 2016 |
Click here to see how this site uses Cookies. View our privacy policy here.