Engineering molecular self-assembly of perylene diimide through pH-responsive chiroptical switching†
Received
10th February 2016
, Accepted 16th March 2016
First published on 5th April 2016
Abstract
The perturbation of non-covalent interactions induced by pH-responsive protonation–deprotonation in HPH resulted in reversible supramolecular chiroptical switching (left-handed to right-handed helical self-assembly) and tunable 1D nanostructures.
Design and engineering of molecular self-assembly and nanoscale morphology by employing external stimuli have been attracting considerable attention in the area of functional and adaptive smart materials.1 In particular, the design of chiroptical switches, with reversible chiral transformation in response to external stimuli, is a prime endeavour in many advanced applications; these include chiral analysis, absolute configurational assignment, optical devices, information storage, light modulators, electro-optical displays, catalysis and sensors.2 Many molecular, supramolecular and polymeric chiroptical switches have been proposed that are responsive to light, temperature, solvent, mechanical forces, chiral/achiral guests, redox/electrochemical potential agents, metal ions and anions.2–3 Given the fact that protonation–deprotonation events are crucial for many of the structural and functional features of biopolymers, the development of pH-responsive supramolecular chiroptical switches is of prime interest in many biomedical applications.4 Although a wide variety of stimuli-responsive self-assembled chiroptical systems are known, pH-triggered chiroptical inversion switching (left or M-type to right or P-type assembly and or vice versa) still remains elusive.
On the other hand, chiral molecular alignment of the electrical and optically active component in one-dimensional (1D) nanostructures with controllable size is of paramount importance in nanotechnology.5,6 Recent efforts made by us and others demonstrated bioinspired molecular design strategies for the creation and engineering of supramolecular chiral organization of functional π-conjugated systems with diverse structural, chemical and physical properties.5–13 However, the art of pre-programmed pH-responsive supramolecular chiral inversion switching in water has not been mastered yet. In an effort to embark on this challenging task, herein we developed histidine-functionalized perylene diimide (HPH) as a pH-responsive reversible engineering of chiroptical and 1D nanoscale morphology switching properties in water (Fig. 1). Perylene diimides (PDIs) are one of the extensively studied classes of n-type organic semiconductor materials. On account of high π-acidity, well-defined redox behaviour, and photophysical properties, PDI and its derivatives have been employed for a wide range of applications ranging from materials to biomedicine.7 The imidazole side chain of histidine can act as an acid as well as a base over the physiological pH range. Due to these peculiar properties, including pH-responsive protonation–deprotonation and the presence of hydrogen bond donors and acceptors on the imidazole side chain, histidine plays a significant role in protein assembly, stability and function. Therefore, the designed HPH can exist in the zwitterionic form under physiological pH with two pKa values of 4.1 (carboxylic acid) and 7.3 (imidazole ring) and is expected to exhibit distinct pH-dependent self-organization properties (Fig. 1). Remarkably, the extensive spectroscopy and microscopy investigations during this study indicated the pH-responsive and reversible supramolecular chiroptical (left-handed to right-handed helical aggregates) and 1D morphology (thin fibers, thick fibers and belts) switching properties of HPH over several cycles, as shown in Fig. 1.
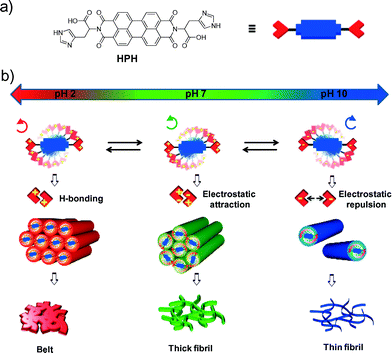 |
| Fig. 1 a) Molecular structure and schematic representation of HPH. b) Schematic illustration of pH-responsive reversible chiroptical switching and protonation–deprotonation induced perturbation in non-covalent interactions leading to the tunable 1D morphology of HPH, respectively. Curly arrows indicate the handedness of the molecular assembly of HPH. | |
HPH was prepared through a straightforward one-step reaction by the condensation of L-histidine with 3,4,9,10-perylene tetracarboxylic dianhydride (PDA) in the presence of imidazole at 120 °C for 1 h in good yield (76%).7b,8 The self-assembly behaviour of HPH in water was studied by recording the optical properties using UV-vis absorption, fluorescence emission, and circular dichroism (CD) spectroscopy (Fig. 2). The absorption spectrum of HPH in DMSO solution exhibited well-resolved vibronic absorption bands at 525 nm (A0–0 transition), 489 nm (A0–1 transition), 458 nm (A0–2 transition) and 430 nm (A0–3 transition) with a Franck–Condon progression (A0–0/A0–1) of 1.59, and the characteristics of S0–S1 transitions polarized along the long axis of the molecularly dissolved PDI chromophore (Fig. 2a).7b The corresponding emission spectrum showed the non-aggregated molecular state of HPH in DMSO solution by displaying slightly Stokes shifted (∼12 nm) mirror image emission bands with sharp emission bands centered at 537 nm, 577 nm and 630 nm, respectively (Fig. 2b). In contrast, the absorption spectrum of HPH in pure water exhibited significantly diminished but red-shifted broad absorption bands, along with a new band at 560 nm (Fig. 2a). The absorption intensity of the A0–0 (532 nm) transition was found to be smaller than that of the A0–1 (503 nm) transition band with a Franck–Condon progression (A0–0/A0–1) value of 0.90. On the other hand, the fluorescence emission spectrum displayed large Stokes shifted (∼23 nm) emission bands with λmax at 548 nm, as compared to the DMSO solution (Fig. 2b). This signifies the formation of J-type aggregates of HPH through the PDI π-surface enforced by the hydrophobic forces in water.9 The effect of the L-histidine (chiral) conjugation in HPH on its long-range molecular ordering in water was investigated by circular dichroism (CD) spectroscopy measurements (Fig. 2c). The DMSO solution exhibited a flat CD signal attributed to its molecularly dissolved state. Remarkably, HPH in pure water displayed an intense bisignate CD signal in the PDI absorption region (250–610 nm) with negative Cotton effects at 594 nm and 350 nm, followed by positive Cotton effects at 506 nm and 303 nm, and zero crossings at 529 nm and 328 nm, respectively. These are characteristic features of excitonically coupled left-handed (M-type) helical organization of PDI chromophores (Fig. 2c and 1b).10 This chiral self-organization of HPH in water clearly signifies the transcription of chiral information from L-histidine to self-assembled PDI chromophores.6c In order to gain further insights into the chiral self-assembly of HPH, we carried out CD measurements at different temperatures. The melting curve derived by monitoring the CD data at 594 nm (at 0.1 K intervals with a ramp rate of 1 K min−1) as a function of the fraction of aggregates (α) versus temperature (K) showed a smooth sigmoidal curve, indicative of the isodesmic self-assembly mechanism. The isodesmic self-assembly of HPH in water was further confirmed by the excellent fitting of the melting curve using the Boltzmann equation.11 Using this data, we have extracted a melting temperature of 322.05 K at α(T) = 0.5, and average stack lengths (DPN) of 10.7 and 1.1 at 298.15 K and 333.15 K, respectively, in water.
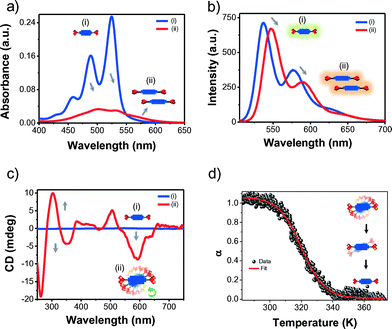 |
| Fig. 2 Optical studies and corresponding schematic illustration of the molecular assembly of HPH in DMSO (i) and water (ii): a) UV-vis absorption spectra; inset: schematic illustration of the molecularly dissolved (i) and J-type molecular organization (ii). b) Fluorescence emission spectra; inset: schematic illustration of emissive molecularly dissolved (i) and J-type aggregated (ii) HPH, respectively. c) CD spectra; inset: schematic illustration of the molecularly dissolved (i) to left-handed helical organization (ii). d) Temperature-dependent CD spectra as a function of the fraction of aggregates (α) versus temperature (K) recorded at 594 nm and isodesmic model fitting; inset: schematic illustration of the temperature-induced disassembly of HPH. Arrows indicate the changes in the spectral features going from DMSO to water. The curly arrow indicates the handedness of the molecular assembly of HPH. | |
In line with our strategy, we next studied the pH-responsive self-assembly modulation of HPH by measuring the changes in optical properties. As the pH of the solution increased from 1 to 11, HPH displayed distinct absorption intensities at A0–0 (545 nm) and A0–1 (500 nm) electronic transitions (Fig. 3a inset and S1†). The respective Franck–Condon progression (A0–0/A0–1) values were found to be 0.99 at low pH (from 1 to 2), 0.67 at neutral pH 7 and 1.05 at basic pH 11. These distinct Franck–Condon progression values clearly suggested the formation of pH-tunable π-stacked aggregates of HPH in aqueous solution. In agreement with the absorption data, the pH-dependent emission spectra displayed a decrease in fluorescence intensity as a function of increasing pH of the solution (Fig. 3c inset and S2†). To probe the pH-responsive chiral organization of HPH, we carried out CD measurements (Fig. 3e inset and S3†). Interestingly, the CD spectra showed opposite Cotton effects corresponding to extreme acidic and basic conditions. At pH 1–2, HPH exhibited an intense bisignate CD signal in the PDI absorption region with a negative Cotton effect at 565 nm, followed by a positive Cotton effect at 473 nm and zero crossovers at 514 nm, which is a characteristic feature of excitonically coupled M-type helical organization of PDI chromophores. A further increase in pH from 2 to 9 resulted in red-shifted (∼28 nm) but relatively less intense CD signals with similar spectral features to those observed for pH 1 to 2. However, under strongly basic conditions (pH 10 and 11), a relatively less intense but inverted bisignate CD spectrum was observed with a positive Cotton effect at 565 nm followed by a negative Cotton effect at 533 nm and zero crossovers at 551 nm, indicating the formation of excitonically coupled P-type helical organization of PDI chromophores. Therefore, the handedness of the self-assembled HPH is pH-responsive in nature as it exhibited M-type helical organization at acidic and neutral pH and P-type helical organization at basic pH.
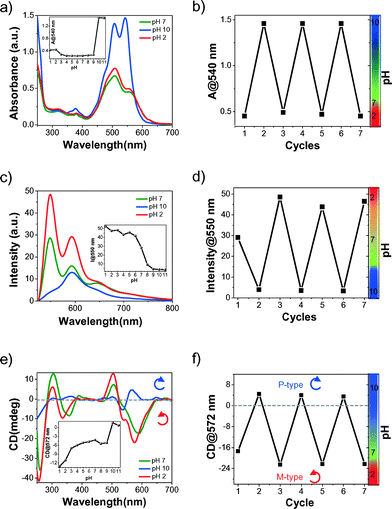 |
| Fig. 3 pH-responsive optical switching of HPH. a) UV-vis absorption spectra, inset: plot of absorbance at 540 nm versus pH. b) Reversible change in absorbance at 540 nm by repeated pH cycles. c) Fluorescence emission spectra, inset: plot of fluorescence intensity at 550 nm versus pH. d) Reversible change in fluorescence emission intensity at 550 nm by repeated pH cycles. e) CD spectra, inset: plot of CD intensity at 572 nm versus pH. f) Reversible chiroptical switching at 572 nm as a function of pH cycles. Curly arrows indicate the handedness of the molecular assembly of HPH. | |
We further investigated the reversibility of the chiro-optical switching properties of HPH self-assembly between M-type and P-type as a function of pH. Initially, spectral studies were performed at neutral pH 7 followed by adjusting the pH from 2 to 10 using 1 M HCl and 1 M NaOH aqueous solutions, respectively. This procedure was repeated over several cycles and the UV-vis absorption, fluorescence emission, and CD spectral changes were recorded. UV-vis absorption studies showed reversible switching of 0–0 (545 nm) and 0–1 (500 nm) transitions. The corresponding Franck–Condon progression values varied from 0.99 to 1.05, as the pH changed from 2 to 10, and vice versa (Fig. 3a). The plot of absorbance at the 0–0 (545 nm) transition versus the number of cycles clearly showed the reversible self-assembly switching of HPH and this was found to be effective even after seven cycles, as shown in Fig. 3b. In agreement with the absorption studies, fluorescence emission studies also showed a similar reversible turn on-off emission intensity at 550 nm as the pH of the solution switched between 2 and 10 (Fig. 3c). This fluorescence switching behaviour, as a function of pH, was reversible over several cycles (Fig. 3d). Next, we carried out CD studies to understand the reversibility of the chiroptical switching between M- and P-type helical assemblies of HPH at pH 2 and pH 10, respectively, in cycles. The CD data clearly demonstrated pH-induced chiroptical switching, as reflected in the changeover of the negative to positive CD features of HPH at 570 nm between pH 2 and pH 10, corresponding to M- and P-helical organization, respectively (Fig. 3e). This pH-induced chiroptical switching was found to be highly reversible and was achieved over several cycles (Fig. 3f). These results clearly suggested the protonation (at pH 2) and deprotonation (at pH 10) of imidazole nitrogen and carboxylic acid functionality, which induced the inversion of the self-assembly of HPH from M-type to P-type, respectively.
In order to study the effect of the pH-induced molecular self-organization of HPH on the nanoscale morphology, we carried out field emission scanning electron microscopy (FESEM) studies under variable pH (2, 7, and 10) conditions. Interestingly, going from lower (2) to higher (10) pH, a drastic difference in the morphology of HPH was observed (Fig. 4). At pH 10, HPH self-assembled into a uniformly distributed, micrometer long thin nanofibril network with a 12 ± 1 nm width (Fig. 4c). At pH 7, relatively thickly intertwined fibril bundles with a 22 ± 1 nm width were observed (Fig. 4b). However, the loss of supramolecular chirality at the nanoscale level may be due to the lack of long-range transcription of the configurational chirality at the molecular level to the morphological chirality of the superstructure.12 Interestingly, at pH 2, the fiber bundles transformed into interconnected belt structures 120 ± 5 nm in width and a few micrometers in length, indicative of interfibrillar interactions (Fig. 4a). In addition, Dynamic Light Scattering (DLS) experiments showed a gradual decrease in the mean size of the self-assembled HPH as the pH of the solution increased from 2 to 10. The acidic solution (pH 2) showed larger self-assembled aggregates with a size distribution over 2000–6400 nm (Fig. 4d). On the other hand, the basic solution (pH 10) displayed relatively small aggregates with a mean size distribution of 80–140 nm (Fig. 4f). Interestingly, in the neutral solution (pH 7), the coexistence of both smaller and larger self-assembled aggregates was clearly indicated by displaying a broad size distribution and scattering signals in the range of 80–6400 nm with characteristic features similar to those found in pH 10 as well as pH 2, respectively (Fig. 4e). Therefore, DLS data confirmed the existence of free-standing aggregates, which is in good agreement with the FESEM data and rules out the presence of any surface artefacts. Thus, the observed pH-responsive self-assembly and corresponding tunable nanoscale morphology of HPH can be explained based on the variation in the charge of imidazole nitrogen and carboxylic acid (histidine) on either side of the PDI chromophore. Under basic pH conditions, the fibrils become negatively charged (deprotonation of carboxylic acid to form a carboxylate anion), resulting in repulsive interfibrillar interactions and favoured thin nanofibrils. As the pH decreased, the imidazole nitrogen and carboxylate groups were gradually protonated, minimizing the interfibrillar repulsion, thus allowing greater interfibrillar interactions that resulted in the transformation of the fibril bundles into a belt structure, as revealed by the FESEM images (Fig. 4g). Overall, the detailed spectroscopy and microscopy studies showed the pH-responsive switching of the HPH self-assembly through modulation of the electrostatic attractive and repulsive interactions followed by hydrogen bonding and π–π interactions (Fig. 4g). This tunable molecular self-assembly of HPH resulted in chiroptical inversion and 1D morphology switching at different pH values, which are reversible over several cycles.
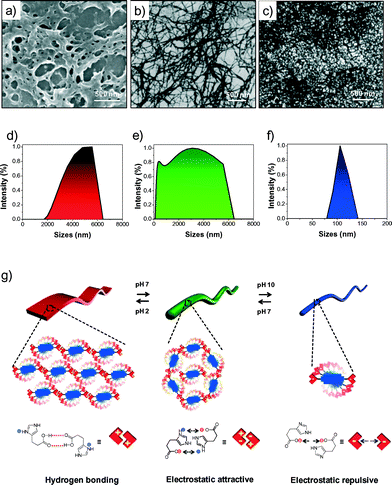 |
| Fig. 4 FESEM micrographs (a–c) and dynamic light scattering (DLS) studies (d–f) of HPH at pH 2 (a and d), pH 7 (b and e) and pH 10 (c and f). g) Schematic representation of the pH-responsive reversible morphology and chiral self-assembly switching via electrostatic repulsion, attraction and hydrogen bonding interactions among π-stacked HPH in water. | |
Conclusions
In summary, a bioinspired PDI bolaamphiphile with histidine auxiliaries (HPH) was designed and its pH-responsive self-assembly behaviour was investigated using various spectroscopy and microscopy techniques. The pH-responsive helical molecular organization and reversible chiro-optical inversion switching (M- to P-type or vice versa) of HPH were validated through UV-visible absorption, fluorescence emission and CD studies. Microscopy studies showed a 1D nanostructure with pH-tunable lateral dimensions from thin fibers (pH 10) to thick fibers (pH 7) and belts (pH 2). Overall, for the first time, we demonstrated the pH-responsive perturbation of non-covalent interactions affecting the self-assembly of HPH in water and resulting in reversible supramolecular chiroptical inversion and morphology switching properties. On account of different molecular packing geometries, the observed 1D nanostructures shown in Fig. 4 are expected to exhibit distinct charge transport properties.13 This study provides valuable insights into the design and engineering of bioinspired chiral self-assembly in water for exploring future smart bioelectronics with chiroptical and biomedical applications.
Acknowledgements
The authors thank Prof. C. N. R. Rao for constant support, JNCASR, DBT, and the Govt. of India (IYBA grant BT/03/IYBA/2010) for financial support.
Notes and references
-
(a) Z. Qi and C. A. Schalley, Acc. Chem. Res., 2014, 47, 2222–2233 CrossRef CAS PubMed
;
(b) Y. Lu and J. Liu, Acc. Chem. Res., 2007, 40, 315–323 CrossRef CAS PubMed
;
(c) S. Mura, J. Nicolas and P. Couvreur, Nat. Mater., 2013, 12, 991–1003 CrossRef CAS PubMed
;
(d) A. P. Blum, J. K. Kammeyer, A. M. Rush, C. E. Callmann, M. E. Hahn and N. C. Gianneschi, J. Am. Chem. Soc., 2015, 137, 2140–2154 CrossRef CAS PubMed
;
(e) T. Aida, E. W. Meijer and S. I. Stupp, Science, 2012, 335, 813–817 CrossRef CAS PubMed
;
(f) M. Aono and K. Ariga, Adv. Mater., 2016, 28, 989–992 CrossRef CAS PubMed
;
(g) M. Yoshida and J. Lahann, ACS Nano, 2008, 2, 1101–1107 CrossRef CAS PubMed
;
(h) T. Fenske, H.-G. Korth, A. Mohr and C. Schmuck, Chem. – Eur. J., 2012, 18, 738–755 CrossRef CAS PubMed
;
(i) H. Kar and S. Ghosh, Nat. Chem., 2015, 7, 765–767 CrossRef CAS PubMed
;
(j) M. B. Avinash and T. Govindaraju, Nanoscale, 2014, 6, 13348–13369 RSC
.
-
(a) G. Haberhauer, Angew. Chem., Int. Ed., 2010, 49, 9286–9289 CrossRef CAS PubMed
;
(b) T. Yamada, Y. Nagata and M. Suginome, Chem. Commun., 2010, 46, 4914–4916 RSC
;
(c) B. L. Feringa, R. A. van Delden, N. Koumura and E. M. Geertsema, Chem. Rev., 2000, 100, 1789–1816 CrossRef CAS PubMed
;
(d) M. Irie, Chem. Rev., 2000, 100, 1685–1716 CrossRef CAS PubMed
;
(e) J. W. Canary, Chem. Soc. Rev., 2009, 38, 747–756 RSC
;
(f) K. J. Chang, B. N. Kang, M. H. Lee and K. S. Jeong, J. Am. Chem. Soc., 2005, 127, 12214–12215 CrossRef CAS PubMed
;
(g) H. Maeda, Y. Bando, K. Shimomura, I. Yamada, M. Naito, K. Nobusawa, H. Tsumatori and T. Kawai, J. Am. Chem. Soc., 2011, 133, 9266–9269 CrossRef CAS PubMed
;
(h) A. Martinez, L. Guy and J. P. Dutasta, J. Am. Chem. Soc., 2010, 132, 16733–16734 CrossRef CAS PubMed
;
(i) H. Miyake, K. Yoshida, H. Sugimoto and H. Tsukube, J. Am. Chem. Soc., 2004, 126, 6524–6525 CrossRef CAS PubMed
;
(j) J. M. Suk, V. R. Naidu, X. Liu, M. S. Lah and K. S. Jeong, J. Am. Chem. Soc., 2011, 133, 13938–13941 CrossRef CAS PubMed
;
(k) T. Ogoshi, T. Akutsu, D. Yamafuji, T. Aoki and T. A. Yamagishi, Angew. Chem., Int. Ed., 2013, 52, 8111–8115 CrossRef CAS PubMed
;
(l) M. Liu, L. Zhang and T. Wang, Chem. Rev., 2015, 115, 7304–7397 CrossRef CAS PubMed
;
(m) P. C. Knipe, S. Thompson and A. D. Hamilton, Chem. Sci., 2015, 6, 1630–1639 RSC
;
(n) N. Marino, D. Armentano, E. Pardo, J. Vallejo, F. Neve, L. Di Donna and G. De Munno, Chem. Sci., 2015, 6, 4300–4305 RSC
;
(o) K. M. Bak and M. J. Chmielewski, Eur. J. Org. Chem., 2015, 19, 4077–4080 CrossRef
;
(p) J. Brioche, S. J. Pike, S. Tshepelevitsh, I. Leito, G. A. Morris, S. J. Webb and J. Clayden, J. Am. Chem. Soc., 2015, 137, 6680–6691 CrossRef CAS PubMed
;
(q) J. Zheng, W. Q. Qiao, X. H. Wan, J. P. Gao and Z. Y. Wang, Chem. Mater., 2008, 20, 6163–6168 CrossRef CAS
;
(r) G. Beer, C. Niederalt, S. Grimme and J. Daub, Angew. Chem., Int. Ed., 2000, 39, 3252–3255 CrossRef CAS
;
(s) Z. Y. Wang, E. K. Todd, X. S. Meng and J. P. Gao, J. Am. Chem. Soc., 2005, 127, 11552–11553 CrossRef CAS PubMed
;
(t) X. Jiang, Y.-K. Lim, B. J. Zhang, E. A. Opsitnick, M.-H. Baik and D. Lee, J. Am. Chem. Soc., 2008, 130, 16812–16822 CrossRef CAS PubMed
;
(u) S. Akine, S. Sairenji, T. Taniguchi and T. Nabeshima, J. Am. Chem. Soc., 2013, 135, 12948–12951 CrossRef CAS PubMed
;
(v) Z. Dai, J. Lee and W. Zhang, Molecules, 2012, 17, 1247–1277 CrossRef CAS PubMed
.
-
(a) J. Kim, J. Lee, W. Y. Kim, H. Kim, S. Lee, H. C. Lee, Y. S. Lee, M. Seo and S. Y. Kim, Nat. Commun., 2015, 6, 6959 CrossRef CAS PubMed
;
(b) E. Ohta, H. Sato, S. Ando, A. Kosaka, T. Fukushima, D. Hashizume, M. Yamasaki, K. Hasegawa, A. Muraoka, H. Ushiyama, K. Yamashita and T. Aida, Nat. Chem., 2011, 3, 68–73 CrossRef CAS PubMed
;
(c) S. Manchineella, V. Prathyusha, U. D. Priyakumar and T. Govindaraju, Chem. – Eur. J., 2013, 19, 16615–16624 CrossRef CAS PubMed
;
(d) S. Takashima, H. Abe and M. Inouye, Chem. Commun., 2011, 47, 7455–7457 RSC
;
(e) B. L. Feringa, J. Organomet. Chem., 2007, 72, 6635–6652 CrossRef CAS PubMed
;
(f) Y. Hua and A. H. Flood, J. Am. Chem. Soc., 2010, 132, 12838–12840 CrossRef CAS PubMed
;
(g) R. Katoono, Y. Tanaka, K. Kusaka, K. Fujiwara and T. Suzuki, J. Organomet. Chem., 2015, 80, 7613–7625 CrossRef CAS PubMed
;
(h) H. Maeda, T. Shirai, Y. Bando, K. Takaishi, M. Uchiyama, A. Muranaka, T. Kawai and M. Naito, Org. Lett., 2013, 15, 6006–6009 CrossRef CAS PubMed
;
(i) K. Takaishi, A. Muranaka, M. Kawamoto and M. Uchiyama, Org. Lett., 2012, 14, 276–279 CrossRef CAS PubMed
;
(j) M. Pandeeswar and T. Govindaraju, J. Inorg. Organomet. Polym. Mater., 2015, 25, 293–300 CrossRef CAS
;
(k) A. Gopal, M. Hifsudheen, S. Furumi, M. Takeuchi and A. Ajayaghosh, Angew. Chem., Int. Ed., 2012, 51, 10505–10509 CrossRef CAS PubMed
.
-
(a) H. Frisch, J. P. Unsleber, D. Luedeker, M. Peterlechner, G. Brunklaus, M. Waller and P. Besenius, Angew. Chem., Int. Ed., 2013, 52, 10097–10101 CrossRef CAS PubMed
;
(b) H. Frisch and P. Besenius, Macromol. Rapid Commun., 2015, 36, 346–363 CrossRef CAS PubMed
.
-
(a) L. Zang, Y. Che and J. S. Moore, Acc. Chem. Res., 2008, 41, 1596–1608 CrossRef CAS PubMed
;
(b) Y. S. Zhao, H. Fu, A. Peng, Y. Ma, Q. Liao and J. Yao, Acc. Chem. Res., 2010, 43, 409–418 CrossRef CAS PubMed
;
(c) S. Chen, P. Slattum, C. Wang and L. Zang, Chem. Rev., 2015, 115, 11967–11998 CrossRef CAS PubMed
;
(d) B. Su, Y. Wu and L. Jiang, Chem. Soc. Rev., 2012, 41, 7832–7856 RSC
;
(e) J. D. Tovar, Acc. Chem. Res., 2013, 46, 1527–1537 CrossRef CAS PubMed
;
(f) S. H. Kim and J. R. Parquette, Nanoscale, 2012, 4, 6940–6947 RSC
;
(g) G. Echue, G. C. Lloyd-Jones and C. F. J. Faul, Chem. – Eur. J., 2015, 21, 5118–5128 CrossRef CAS PubMed
;
(h) J. Hu, W. Kuang, K. Deng, W. Zou, Y. Huang, Z. Wei and C. F. J. Faul, Adv. Funct. Mater., 2012, 22, 4149–4158 CrossRef CAS
;
(i) Y. Huang, J. Hu, W. Kuang, Z. Wei and C. F. J. Faul, Chem. Commun., 2011, 47, 5554–5556 RSC
.
-
(a) M. Pandeeswar, H. Khare, S. Ramakumar and T. Govindaraju, RSC Adv., 2014, 4, 20154–20163 RSC
;
(b) M. Pandeeswar and T. Govindaraju, RSC Adv., 2013, 3, 11459–11462 RSC
;
(c) M. Pandeeswar, M. B. Avinash and T. Govindaraju, Chem. – Eur. J., 2012, 18, 4818–4822 CrossRef CAS PubMed
;
(d) D. Adam, P. Schuhmacher, J. Simmerer, L. Haeussling, K. Siemensmeyer, K. H. Etzbach, H. Ringsdorf and D. Haarer, Nature, 1994, 371, 141–143 CrossRef CAS
;
(e) V. Percec, M. Glodde, T. K. Bera, Y. Miura, I. Shiyanovskaya, K. D. Singer, V. S. K. Balagurusamy, P. A. Heiney, I. Schnell, A. Rapp, H. W. Spiess, S. D. Hudson and H. Duan, Nature, 2002, 419, 384–387 CrossRef CAS PubMed
;
(f) X. L. Feng, V. Marcon, W. Pisula, M. R. Hansen, J. Kirkpatrick, F. Grozema, D. Andrienko, K. Kremer and K. Mullen, Nat. Mater., 2009, 8, 421–426 CrossRef CAS PubMed
;
(g) J. Autschbach, L. Nitsch-Velasquez and M. Rudolph, Top. Curr. Chem., 2011, 298, 1–98 CrossRef CAS PubMed
;
(h) Y. Zhang, P. Chen, L. Jiang, W. Hu and M. Liu, J. Am. Chem. Soc., 2009, 131, 2756–2757 CrossRef CAS PubMed
;
(i) Y. Yang, R. C. da Costa, M. J. Fuchter and A. J. Campbell, Nat. Photonics, 2013, 7, 634–638 CrossRef CAS
;
(j) M. Pandeeswar, H. Khare, S. Ramakumar and T. Govindaraju, Chem. Commun., 2015, 51, 8315–8318 RSC
.
-
(a) F. Würthner, C. R. Saha-Möller, B. Fimmel, S. Ogi, P. Leowanawat and D. Schmidt, Chem. Rev., 2016, 116, 962–1052 CrossRef PubMed
;
(b) A. K. Dwivedi, M. Pandeeswar and T. Govindaraju, ACS Appl. Mater. Interfaces, 2014, 6, 21369–21379 CrossRef CAS PubMed
;
(c) M. B. Avinash and T. Govindaraju, Adv. Mater., 2012, 24, 3905–3922 CrossRef CAS PubMed
;
(d) Y. Che, X. Yang and L. Zang, Chem. Commun., 2008, 1413–1415, 10.1039/b719384j
;
(e) K. Peneva, G. Mihov, A. Herrmann, N. Zarrabi, M. Borsch, T. M. Duncan and K. Mullen, J. Am. Chem. Soc., 2008, 130, 5398–5399 CrossRef CAS PubMed
;
(f) X. Chen, M. J. Jou and J. Yoon, Org. Lett., 2009, 11, 2181–2184 CrossRef CAS PubMed
;
(g) B. Wang and C. Yu, Angew. Chem., Int. Ed., 2010, 49, 1485–1488 CrossRef CAS PubMed
;
(h) H. Szelke, S. Schubel, J. Harenberg and R. Kramer, Chem. Commun., 2010, 46, 1667–1669 RSC
;
(i) M. Sadrai and G. R. Bird, Opt. Commun., 1984, 51, 62–64 CrossRef CAS
;
(j) W. E. Ford and P. V. Kamat, J. Phys. Chem., 1987, 91, 6373–6380 CrossRef CAS
;
(k) L. Schmidt-Mende, A. Fechtenkotter, K. Mullen, E. Moons, R. H. Friend and J. D. MacKenzie, Science, 2001, 293, 1119–1122 CrossRef CAS PubMed
;
(l) J. Li, F. Dierschke, J. Wu, A. C. Grimsdale and K. Muellen, J. Mater. Chem., 2006, 16, 96–100 RSC
;
(m) T. Ye, R. Singh, H. J. Butt, G. Floudas and P. E. Keivanidis, ACS Appl. Mater. Interfaces, 2013, 5, 11844–11857 CrossRef CAS PubMed
;
(n) R. Singh, M. Mróz Marta, F. Di Fonzo, J. Cabanillas-Gonzalez, E. Marchi, G. Bergamini, K. Müllen, J. Jacob and E. Keivanidis Panagiotis, Org. Photonics Photovoltaics, 2013, 1, 24–38 Search PubMed
;
(o) Y. Liu, N. Wang, Y. Li, H. Liu, Y. Li, J. Xiao, X. Xu, C. Huang, S. Cui and D. Zhu, Macromolecules, 2005, 38, 4880–4887 CrossRef CAS
;
(p) X. Feng, Y. An, Z. Yao, C. Li and G. Shi, ACS Appl. Mater. Interfaces, 2012, 4, 614–618 CrossRef CAS PubMed
;
(q) X. Liu, N. Zhang, J. Zhou, T. Chang, C. Fang and D. Shangguan, Analyst, 2013, 138, 901–906 RSC
;
(r) F. Bo, B. Gao, W. Duan, H. Li, H. Liu and Q. Bai, RSC Adv., 2013, 3, 17007–17010 RSC
;
(s) A. Kalita, S. Hussain, A. H. Malik, N. V. V. Subbarao and P. K. Iyer, J. Mater. Chem. C, 2015, 3, 10767–10774 RSC
;
(t) F. Würthner, C. Thalacker, S. Diele and C. Tschierske, Chem. – Eur. J., 2001, 7, 2245–2253 CrossRef
.
- Y. Xu, S. Leng, C. Xue, R. Sun, J. Pan, J. Ford and S. Jin, Angew. Chem., Int. Ed., 2007, 46, 3896–3899 CrossRef CAS PubMed
.
- F. Würthner, T. E. Kaiser and C. R. Saha-Möller, Angew. Chem., Int. Ed., 2011, 50, 3376–3410 CrossRef PubMed
.
-
(a) J. Gawronski, M. Brzostowska, K. Kacprzak, H. Kolbon and P. Skowronek, Chirality, 2000, 12, 263–268 CrossRef CAS PubMed
;
(b) G. Gottarelli, S. Lena, S. Masiero, S. Pieraccini and G. P. Spada, Chirality, 2008, 20, 471–485 CrossRef CAS PubMed
.
- N. Ponnuswamy, G. D. Pantos, M. M. Smulders and J. K. Sanders, J. Am. Chem. Soc., 2012, 134, 566–573 CrossRef CAS PubMed
.
- Y. Wang, J. Xu, Y. Wang and H. Chen, Chem. Soc. Rev., 2013, 42, 2930–2962 RSC
.
-
(a) F. S. Kim, G. Ren and S. A. Jenekhe, Chem. Mater., 2011, 23, 682–732 CrossRef CAS
;
(b) A. L. Briseno, S. C. B. Mannsfeld, C. Reese, J. M. Hancock, Y. Xiong, S. A. Jenekhe, Z. Bao and Y. Xia, Nano Lett., 2007, 7, 2847–2853 CrossRef CAS PubMed
.
Footnote |
† Electronic supplementary information (ESI) available: Synthetic procedure, characterization, experimental procedure and additional pH-responsive optical studies. See DOI: 10.1039/c6me00012f |
|
This journal is © The Royal Society of Chemistry 2016 |