DOI:
10.1039/C5MB00627A
(Paper)
Mol. BioSyst., 2016,
12, 102-109
Genetic analysis of the clonal stability of Chinese hamster ovary cells for recombinant protein production†
Received
18th September 2015
, Accepted 4th November 2015
First published on 6th November 2015
Abstract
Chinese hamster ovary (CHO) cells are frequently used for the production of recombinant proteins for therapeutical applications. However, the recombinant protein expression level of CHO cells may reduce during long-term culture. The physiological changes related to the stability of expression were not well understood. In this study, we performed a series of genetic analysis on stable and unstable clonal derived populations. Transcriptome analysis revealed that a large number of differentially expressed genes (>100) were identified in the unstable population between early and late generations, while only a few differentially expressed genes were found in the stable population, suggesting that the gene expression change is related to the instability of recombinant protein production. On the other hand, no significant differences were found in promoter methylation or gene copy numbers in the unstable population. Taken together, our data help better understand the molecular mechanism underlying the stability of recombinant protein production in CHO cells.
Introduction
Chinese hamster ovary (CHO) cells are the most widely used mammalian cell lines for the production of recombinant proteins, such as monoclonal antibodies, Fc fusion proteins, and cytokines, which require proper folding and glycosylation for their full biological activity.1,2 Many CHO cell lines employ dihydrofolate reductase (DHFR) to amplify the gene copy number to increase the expression level of recombinant proteins.3,4 This is achieved by treating the CHO cells with methotrexate (MTX), a DHFR inhibitor. As a result, the region containing the DHFR gene is amplified to many copies.3,5 One unsolved problem is the instability of production using the gene amplification system, especially over long-term culture, leading to loss of productivity.6,7 Constructing a stable CHO cell line for recombinant protein expression could be time-consuming (over three or six months) and labor intensive.2,6,8
Chromosome instability is one of the major reasons for the instability of production cell lines.9 During MTX treatment the CHO cells may experience severe genetic rearrangement10,11 and intraclonal heterogeneity is one of the inherent characteristics for CHO cell lines.12,13 The chromosomes of CHO cells are highly heterogenic14,15 and the landing site of the heterologous gene in chromosome is related to the stability of amplified gene caused by MTX selection.16–20 In addition to the decrease in gene copy number,21 it is suggested that the loss of production from CHO cell lines during long-term culture is also attributed to the modification of the promoter and histone acetylation.6,22
High-throughput mRNA sequencing (RNA-seq) offers the ability to measure global gene expression in a single assay.23–27 It could be used as a tool to better understand the molecular mechanisms of the instability of recombinant protein production in CHO cells, especially considering that the genomic sequence of the CHO cell line had been completed.28
In this study, two clonal derived populations, one stable and one unstable for recombinant protein production, were isolated from a single population of CHO cells treated with MTX. A series of genetic analyses were conducted in order to better understand the molecular mechanism of the instability of CHO cells for recombinant protein production during long term culture.
Results
Selection of stable and unstable cell lines
The DHFR deficient CHO cells (DG44) were transfected with a Transmembrane Activator and CAML Interactor Fc (TACI-Fc) fusion protein gene and the DHFR gene. Six clonal derived populations with the highest productivity of the TACI-Fc protein (>4 pg per cell per day) were selected for further analysis. After MTX treatment, the TACI-Fc productivity of one population increased from 4.2 pg per cell per day to 18.7 pg per cell per day. This population was named B19A6 and was selected for sub-clonal screening. After multiple selections, one sub-clonal population was identified to have a stable production of TACI-Fc during long-term culture (∼50 generations). The TACI-Fc expression level ranged from 24 pg per cell per day (13th generation) to 22 pg per cell per day (56th generation). This population was defined as a stable cell line and named B19A6-S. A second sub-clonal population was identified to have a reduced protein production, ranging from 42.0 pg per cell per day (11th generation) to 10.5 pg per cell per day (32nd generation). This population was defined as unstable and named B19A6-U. No significant decrease of protein production from the 32nd (10.5 pg per cell per day) to 56th generation (12.6 pg per cell per day) was observed in B19A6-U. This stable production in later generations could be caused by clearing out the unstable chromosomal regions or entire chromosomes, which has been reported as a main driver in MTX amplified cells.9,19 The selection process is illustrated in Fig. 1, and the stability of cell specific productivity of the two clonal derived populations is shown in Fig. 2.
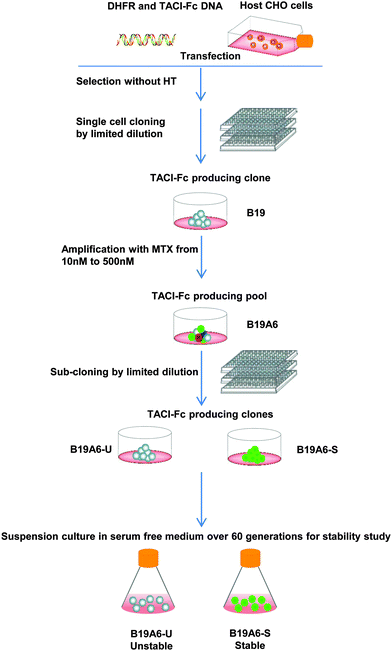 |
| Fig. 1 Flow chart of the experimental process of transfection, selection, cloning, amplification, and sub-cloning. Dots with different colors represent the heterogeneous populations in B19A6. | |
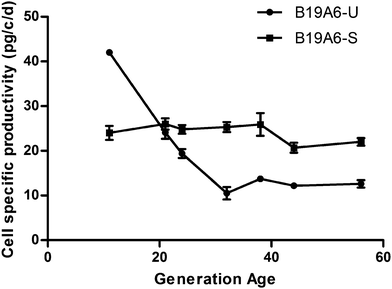 |
| Fig. 2 Detection of cell specific productivity of B19A6-S and B19A6-U during long-term culture. | |
CAG promoter methylation status
DNA methylation has been known to correlate with inhibition of gene expression.29 Recent studies have shown that the loss of productivity in recombinant CHO cell lines is partially due to promoter methylation, causing gene silencing.6,30,31 The methylation status of the CAG promoter, which was used to drive high levels of gene expression of the TACI-Fc protein, was investigated and compared in both B19A6-S and B19A6-U cell lines. Two sample groups were used in each cell line: generation 13 of B19A6-U (B19A6-U-13, high production), generation 39 B19A6-U (B19A6-U-39, low production), generation 14 of B19A6-S (B19A6-S-14) and generation 41 of B19A6-S (B19A6-S-41). Ten individual clones from each group were randomly selected and the methylation of 32 CpG nucleotides was analyzed by bisulfite sequencing PCR (BSP). The average methylation levels for the four groups (B19A6-U-13, B19A6-U-39, B19A6-S-14 and B19A6-S-41) were: 5.3 ± 4.4%, 5.9 ± 3.3%, 3.4 ± 5.3%, and 4.7 ± 4.7%, respectively (Fig. 3). No significant differences were found between any groups (P = 0.74 between B19A6-U-13 and B19A6-U-39; P = 0.60 between B19A6-S-14 and B19A6-S-41). We further analysed the putative transcription factor binding sites using the searching tool of Jaspar database (http://jaspar.genereg.net/cgi-bin/jaspar_db.pl). No significant changes were found on the methylation level of CpG sites that were co-localized with putative transcription factor binding sites. These results suggest that methylation of the CAG promoter was not involved in the levels of TACI-Fc fusion protein expression.
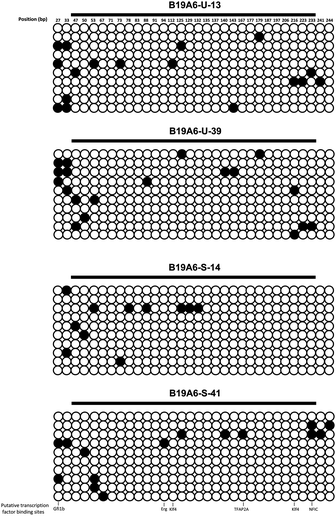 |
| Fig. 3 Methylation levels of the CAG promoter in the high generation and low generation of stable and instable cell lines. Each horizontal line represents an individually sequenced clone (total 10 clones) and each circle represents one CpG residue (32 in each clone). White and black circles represent unmethylated and methylated cytosines, respectively. | |
Gene copy number of cell lines
Another major cause of instability for protein production is the loss of gene copy numbers.6,21 To test if they were involved in the production level and stability of the TACI-Fc protein, the gene copy numbers of the two cell lines were assayed using qPCR. Our results showed that the gene copy numbers for B19A6-U-13 and B19A6-U-39 remained at the same level (P = 0.96), being 170.11 ± 13.79 and 168.83 ± 18.45, respectively. However, compared with the gene copy number of B19A6-S-14 (102.36 ± 3.56), the gene copy number of B19A6-S-41 (79.40 ± 6.68) showed a statistically significant decrease (P = 0.015) (Fig. 4). Using the β-actin and GADPH as the reference gene, respectively, the results of the relative copy number of the TACI region showed no significant difference (P > 0.05) (see Fig. S1, ESI†). Furthermore, the relative quantity PCR was employed to analyze changes in the gene copy number of the CAG promoter, TACI, Fc and the downstream sequence of Fc in high generation cells relative to low generation cells. No significant difference was found in all gene regions (see Fig. S2, ESI†).
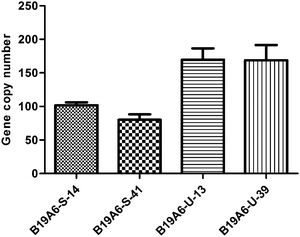 |
| Fig. 4 Relative gene copy number of the unstable cell line (B19A6-U-13 and B19A6-U-39) and the stable cell line (B19A6-S-14 and B19A6-S-41). β-actin was used for normalization. | |
Transcriptome analysis of the stable and unstable cell lines
To further understand the mechanism of the stability for protein expression, RNA-seq was used to analyze the gene expression differences among different groups. As shown in Table S1 (ESI†), the greatest degree of differentially-expressed genes was seen between B19A6-U-13 and B19A6-U-39. Compared to B19A6-U-13, 104 genes were down-regulated and 12 were up-regulated in B19A6-U-39. In contrast, only 11 genes were down-regulated and two genes were up-regulated in B19A6-S-41, compared to B19A6-S-14. In addition, compared to B19A6-S-14, 20 genes were down-regulated and 31 genes were up-regulated in B19A6-U-13.
The RNAseq analysis showed that the mRNA level of TACI-Fc of B19A6-U-39 was reduced to 57 percent of the B19A6-U-13 expression value. The TACI-Fc mRNA level between low and high generations of the stable cell line B19A6-S did not change significantly.
Verification of RNA-seq
To validate the RNA-seq results, qPCR was performed to test the expression levels of the five genes that were most significantly changed between B19A6-U-13 and B19A6-U-39 (Fig. 5A). The house-keeping gene β-actin was used as a control. As shown in Fig. 5B, qPCR results showed a similar trend as RNA-seq, suggesting that RNA-seq is a useful tool to study the gene expression at a large scale.
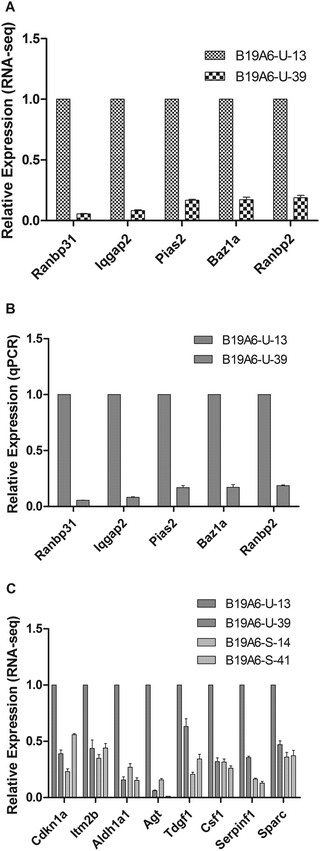 |
| Fig. 5 Fold changes of significantly changed genes. Fold changes of the five most significantly changed genes between B19A6-U-13 and B19A6-U-39 assayed by RNA-seq (A) and by qPCR (B). Fold changes of eight genes enriched in cell proliferation and an apoptosis process in B19A6-U-13, compared with B19A6-S-14, B19A6-S-41 and B19A6-U-39 (C). | |
Gene ontology analysis of differentially expressed genes
The program DAVID,32–34 an online software for gene ontology (GO) analysis, was used to further investigate the physiological functions of the differentially expressed genes. Our results showed that among the 104 down-regulated genes between B19A6-U-13 and B19A6-U-39, the most enriched biological processes were clustered in steroid/sterol metabolic processes, macromolecule catabolic processes, glycosaminoglycan metabolic processes and mRNA transportation and localization processes (see Table S1, ESI†). Three down-regulated genes G3bp2 (GTPase activating protein (SH3 domain) binding protein 2), Ranbp2 (RAN binding protein 2) and Nxf7 (nuclear RNA export factor 7) were enriched in the mRNA transportation process (P = 0.055). The decrease of G3bp2, Ranbp2, and Nxf7 was 64%, 82%, and 88%, respectively. The molecular functions of these genes were enriched in metal ion binding, cation binding and enzyme inhibitor activity (Fig. 6). There were no enriched biological processes for regulated genes in the stable cell line (see Table S1, ESI†).
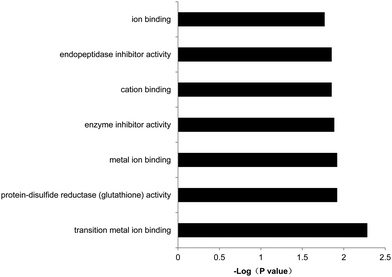 |
| Fig. 6 Gene ontology (GO) analysis of the molecular function of the differentially expressed genes between B19A6-U-39 and B19A6-U-13. X-axis shows the enrichment p value of each gene (−log base 10). | |
Furthermore, comparison between the stable clonal derived populations B19A6-S-14 and the unstable clonal derived populations B19A6-U-13 showed eight up-regulated genes (Agt, Csf1, Cdkn1a, Tdgf1, Sparc, Aldh1a1, Itm2b and Serpinf1) in B19A6-U-13. These genes were involved in either cell proliferation or apoptosis. Agt, Csf1, Cdkn1a, Tdgf1 and SPARC are involved in the PI3K-Akt, MAPK or Ras/Raf signalling pathway.35–37 Aldh1a1 is related to the retinoic acid signalling pathway.38 Serpinf1 promotes proliferation activity in tumor cells.39 Except for Itm2b, which promotes apoptosis activity,40 all the other up-regulated genes were shown to promote cell survival and proliferation, which may lead to decrease of the expression of exogenous recombinant genes to save energy. Interestingly, the expression level of these genes in B19A6-U-39 was similar to that of B19A6-S-14 and maintains a relatively low level in B19A6-S-41 (Fig. 5C).
Conclusions
The stability of gene expression in mammalian cell lines is important for the production of recombinant antibodies and antibody-fusion proteins.2,41 The majority of these proteins are expressed in CHO cells. We employed a series of genetic analyses to gain insights into its molecular mechanism. The increased copy number in the unstable chromosome integrations is prone to loss when cells grow in medium without MTX.9 We found that the gene copy number of B19A6-U was not significantly changed during passage in the absence of MTX. This is consistent with a previous report,11 in which the unstable cells have a significant decrease in GOI (gene of interest) mRNA levels with little change in the gene copy number. Our transcriptome analysis revealed that more genes were down-regulated in the unstable cell lines than the stable cell line. GO analysis and KEGG pathway enrichment, such as the metabolism of fatty acids (see Table S2, ESI†), further showed that these down-regulated genes were mostly involved in metabolism, suggesting that a metabolic slowdown might be the major phenomenon accompanied by production reduction. The down-regulation of metabolism related genes are more obvious in three RNA transportation related genes: G3bp2, Ranbp2, and Nxf7. G3bp2 is one of the RNA binding proteins (RBPs) that is important in controlling of mRNA translation and stability,42–44 formation of stress granule,45,46 and regulation cell cycle.47,48 Ranbp2 acts as a regulator of nucleocytoplasmic transport.49,50 Nxf7 belongs to the nuclear RNA export factor (NXF) family proteins and is involved in mRNA localization in cytoplasm, degradation and sorting of mRNAs.51–53 Down-regulation of these three genes would interrupt the mRNA metabolism and decrease the TACI-Fc mRNA abundance, resulting in the reduction of productivity.
GO analysis revealed that differences in gene expression between B19A6-U-13 and B19A6-S-14 were mostly related to the regulation of cell proliferation and apoptosis. Meanwhile the cell specific productivity of B19A6-U was not further decreased from the 32nd to 56th generation, suggesting that the up-regulation of cell proliferation related genes might be the major cause of instability of recombinant gene production.
A previous study suggests that CHO cells do not undergo further chromosome rearrangement during culture in the absence of MTX.11 On the other hand, as a type of immortalized cells, CHO cells exhibit cell to cell chromosome variations,54,55 especially from cells treated with MTX.9,19 The chromosome instability might be the original force to drive the differential gene expression and decrease productivity in unstable clonal populations.
It has been reported that the loss of gene copy number correlates with the instability of protein production.6 Surprisingly, we observed that the gene copy number remained unchanged in the unstable cell line, but there was a slight decrease in the stable cell line. This decrease does not influence the mRNA abundance of TACI-Fc, suggesting that these genes that are located in non-transcribed integration sites might be lost in high generation cells of the stable cell line.
This report provides a systematic study of the mechanism of protein expression stability of CHO cells. Although this study probably could not completely solve the issue of CHO stability, it will help establish better and more stable cell lines for production of antibodies and other recombinant proteins.
Experimental
Materials and methods
Cell culture and transfection.
The dihydrofolate reductase (DHFR) deficient CHO cell line DG44 (Life Technology, Carlsbad, CA) was used as the host cell line. Cells were cultured in IMDM medium (Thermo Scientific, Logan, UT) supplemented with 10% fetal bovine serum (FBS) (Thermo Scientific, Logan, UT) and 1× hypoxanthine and thymidine (HT) media supplement (Sigma-Aldrich, St. Louis, MO) at 37 °C and 5% CO2. The expression vector, which contains a TACI-Fc fusion protein and a mouse DHFR gene driven by a CAG promoter and a SV40 promoter, was transformed into CHO cells by electroporation. Following transfection, cells were cultured in the serum free Ex-cell 302 medium (Sigma-Aldrich) in a flask without HT for two weeks. Cells were then seeded in 96 well plates at an average of 0.5 cell per well. This was achieved by adjusting the cell density to 1 cell/400 μl in Ex-cell 302 medium supplemented with 10% fetal bovine serum, then 200 μl was added to each well. After the cells grew in 96-well plates for 12 days, single clonal derived populations were picked and transferred to new 96-well plates. The plates were cultured for about 14 days until medium nutrients were depleted. The supernatant from each well was collected, and the concentration of the TACI-Fc fusion protein was measured using a sandwich ELISA assay. Goat anti-human IgG-Fc antibody (1
:
2000) (Bethyl laboratories, Montgomery, TX) was used as the primary antibody for coating and a horse radish peroxidase (HRP)-conjugated goat anti-human IgG-Fc antibody (1
:
5000) (Bethyl laboratories) was used as the secondary antibody for detection. ABST peroxidase substrate solution A (KPL, Gaithersburg, MD) and peroxidase substrate solution B (KPL) were mixed together. The mixed solution was used as the substrate and the absorption was measured at 405 nm on a microplate reader. 10% of the total clonal derived populations with the highest OD values were picked up and seeded in 6-well plates. Then the cell specific productivity for each population was measured using the equation qp = Δp/Δt × ln(Ct/C0)/ΔC, where qp represents cell specific productivity of TACI-Fc; Δp represents the change in TACI-Fc concentration; Δt represents time interval; ΔC represents the change of viable cell density from time 0 to time t; Ct represents viable cell density at time t and C0 represents viable cell density at time 0. Populations with the highest specific productivity were selected for further gene amplification. Viable cell density was measured by a dye exclusion method. Cells were first stained with 0.2% trypan blue, which stains dead cells only, for few seconds. Cells were then examined and counted using a microscope.
Gene amplification, sub-cloning and stability study.
Selected clonal populations were seeded in a 10 cm dish at 5 × 105 cells per dish and cultured in Ex-cell 302 medium containing 10% FBS with 10 nM methotrexate (MTX) (Sigma Aldrich). After 80% confluence, cells were treated with trypsin and reseeded in medium with increased MTX concentration. The concentration of MTX was increased step by step as follows: 10 nM, 20 nM, 50 nM, 100 nM, 200 nM, and 500 nM. Following MTX treatment, specific productivity of the clonal derived populations was measured again. The population with the highest specific production was selected and sub-cloned in 96-well plates using Ex-cell 302 medium containing 10% FBS. The 2–3 sub-clones with high productivity were selected and cultured in suspension in serum free Ex-cell 302 medium without MTX over 50 generations. The specific productivity during the long-term culture was measured using ELISA.
Promoter methylation analysis.
Genomic DNA (gDNA) of cells was extracted using a AxyPrep Multisource Genomic DNA Mini-prep Kit (Axygen, Tewksbury, MA) according to the manufacturer's instruction. Bisulfite conversion was performed on 0.2 mg gDNA from each sample using a BisulFlash™ DNA Modification Kit (Epigentek, Farmingdale, NY) according to the manufacturer's instruction. The bisulfite treated DNA was amplified by nested PCR. The primers (Table 1) were designed using Methyl Primer Express Software v1.0 (Applied Biosystems). The primers specifically amplify a 276 bp CpG island region of the CAG promoter with 32 CpG sites. The amplification of bisulfite modified gDNA was performed using TaKaRa Taq HS as DNA polymerase, with the following PCR reaction program: 95 °C for 3 min, followed by 35 three-step cycles at 95 °C for 30 s, 53 °C for 30 s and at 72 °C for 40 s, followed by a final elongation step at 72 °C for 10 min. The PCR products were separated on 2% agarose gel, and purified using an Axyprep DNA Gel Extraction Kit (Axygen) according to the manufacturer's instruction. The purified DNA fragments were cloned in a PMD-18T vector (Takara, Dalian). At least ten clones were sequenced (Genscript, Nanjing, China) to investigate the methylation status of the CAG promoter.
Table 1 Primers used for CAG promoter methylation detection by BSP and verification of RNA-seq by qPCR
Gene name |
Sequence of primers |
CAG methylation detection |
Outside F: GTAGTTATTGTTTTTTATGGTAAT |
Outside R: TAATAAAACAACACAATAACCAACAC |
Inside F: GGGATTTTTTTTGTTTTAAATTTGTG |
Inside R: ACATAAACATAATTAACAAAAACTC |
Ranbp3l |
F: TGGTCACAGTGCATCCAAAT |
R: CATCTCGATGTTGCCTCTGA |
Iqgap2 |
F: CCCAGCACTACCAGGATGTT |
R: GACCAGTGAAACCCATTGCT |
Pias2 |
F: CCACGGACACTTGAAGGACT |
R: TGAAGCAGCACAGAACCAAC |
Baz1a |
F: GATCGCAGTGTGATGTGGTC |
R: TTTGGTCGACATTCTGGACA |
Ranbp2 |
F: CAAGCCAAGGAGAAAGCAAG |
R: TGTTCCAAACACAGCTGCTC |
Isolation of gDNA and quantification of gene copy numbers.
Genomic DNA (from 1 × 106 cells) was isolated using a TaKaRa MiniBEST Universal Genomic DNA Extraction kit according to the manufacturer's protocol (TaKaRa, Dalian). The concentration and purity of Genomic DNA were measured using a Nanodrop 2000 (Thermo Scientific, Waltham, MA). The gene copy number of the TACI-Fc fusion protein was determined by quantitative real-time PCR (qPCR) using the ABI 7500 Prism Sequence Detection System (Applied Biosystems, Foster City, CA). Real-time PCR was performed using a TaqMan GTXpress Master Mix (Applied Biosystems) and 10 μM of each primer. The forward and reverse primers for Fc were 5′-GAGGAGCAGTACAACAGCACGTA-3′ and 5′-TGCACTTGTACTCCT TGCCATT-3′. The TaqMan probe for Fc was FAM-TCAGCGTCCTCACCGTCCTGCA-TAMRA. 50 ng gDNA was input in each reaction, respectively. CHO genomic DNA with a known amount of Fc fragment was used as the standard. β-actin was used for normalization. qPCR was performed with a pre-incubation cycle at 95 °C for 10 min followed by 40 cycles of amplification (10 s at 95 °C and 60 s at 60 °C). The specificity of qPCR was confirmed by the presence of a single peak in the dissociation curve. The cycle threshold (Ct) was determined after manually setting the threshold in the linear amplification phase of the reactions and averaged from duplicate samples. Relative qPCR quantification of gene copy numbers was examined by the ΔΔCT method.56 CAG, TACI, Fc and 3′ flanking sequences of Fc were used as target genes. GAPDH or β-actin was used as a reference gene. The forward and reverse oligonucleotide primers for CAG were 5′-TATTGTGCTGTCTCATCATTTTGGC-3′ and 5′-CGGAACTAGATCCTGTGAGAAGCAG-3′. The primers for TACI were 5′-GGGCACCGTCAGTCTTCCTCTT-3′ and 5′-GCGGCTTTGTCTTGGCATTAT-3′. The primers for the 3′ flanking sequence of Fc were 5′-CCATCTGTTGTTTGCCCCTCC-3′ and 5′-CCTACTCAGACAATGCGATGCA-3′. The primers for β-actin were 5′-GTCGTACCACTGGCATTGTG-3′ and 5′-AGGGCAACATA GCACAGCTT-3′. The primers for GAPDH were 5′-GCGACTTCAACAGTGACTCCCA-3′ and 5′-TGAGGTCCACC ACTCTGTTGCT-3′. All experiments were run in triplicate.
Total RNA extraction and cDNA synthesis.
The selected CHO clonal populations (from 1 × 106 cells each) were collected at the exponential phase (80% confluence) and total RNA was extracted using the TRIzol (Life Technologies) method. The quality of the extracted RNA was measured by a Nanodrop 2000 (Thermo Scientific). The RNA was subsequently used as a template for cDNA. First-strand and second-strand cDNA were synthesized using a cDNA Synthesis Kit (TaKaRa biotechnology) according to the manufacturer's protocol.
RNA sequencing and data analyses.
RNA sequencing (RNA-seq) and data analyses were performed as previously described.57 Briefly, the extracted mRNA was enriched from total RNA using the TRIzol (Life Technologies) method and the mRNA was cut into short fragments ranging from 200 to 500 bp using an RNA fragmentation kit (Ambion, Austin, TX). The double-strand cDNA was synthesized by random primers and purified using a QIAquick PCR extraction kit (Qiagen, Hilden, Germany) and used for end repair and base addition. For high-throughput RNA-seq, the sequencing library was constructed according to the manufacturer's instructions of BGI-Shenzhen (Beijing Genomics Institute, Shenzhen, China). Paired-end sequence reads were generated using an Illumina HiSeq 2000 instrument with TruSeq v3 reagents (BGI-Shenzhen).
For RNA-Seq sequence reads, one base was trimmed from the 5′ end and the reads were aligned to the CHO genome database and transcriptome using the TopHat program (version 2.0.4).25 The Refseq gene annotation file was also provided for TopHat. The Cuffdiff program58 was used to calculate the relative abundance of each gene. A gene was defined to be differentially expressed with statistical significance if the q-value calculated by Cuffdiff was less than 0.05. Gene ontology (GO) analysis was performed using the online program DAVID32–34 (http://david.abcc.ncifcrf.gov/).
Quantitative real-time PCR.
A QRT-PCR assay was conducted in triplicate using 80 ng of cDNA in a 20 μl reaction volume using the SYBR Green master mix (TaKaRa biotechnology) on a 7500 ABI Prism Sequence Detection System (Applied Biosystems). QRT-PCR was performed with a pre-incubation cycle at 95 °C for 10 min followed by 40 cycles of amplification (10 s at 95 °C and 60 s at 60 °C). The measured genes were assayed using qRT-PCR. β-actin was used for normalization. The primers used for each gene are listed in Table 1.
Statistical analysis.
Data are expressed as mean ± standard derivation (S.D.). Differences in promoter methylation status and gene copy numbers between low and high generation groups were tested for significances using the student's t test. Differences were considered as statistically significant when P < 0.05.
Acknowledgements
This study was supported by National Science and Technology Key Projects for “Major Drug Innovation and Development”, grant #2013ZX09401002, National High Technology R&D Program (863 Program) of China, grant #2012AA02A302, and National Science Foundation of China (NSFC), grant #1470896.
Notes and references
- S. Hammond and K. H. Lee, Biotechnol. Bioeng., 2012, 109, 528–535 CrossRef CAS PubMed.
- F. M. Wurm, Nat. Biotechnol., 2004, 22, 1393–1398 CrossRef CAS PubMed.
- S. J. Kim and G. M. Lee, Biotechnol. Bioeng., 1999, 64, 741–749 CrossRef CAS PubMed.
- W. F. Flintoff, E. Livingston, C. Duff and R. G. Worton, Mol. Cell. Biol., 1984, 4, 69–76 CrossRef CAS PubMed.
- R. J. Kaufman, P. A. Sharp and S. A. Latt, Mol. Cell. Biol., 1983, 3, 699–711 CrossRef CAS PubMed.
- M. Kim, P. M. O'Callaghan, K. A. Droms and D. C. James, Biotechnol. Bioeng., 2011, 108, 2434–2446 CrossRef CAS PubMed.
- L. A. Bailey, D. Hatton, R. Field and A. J. Dickson, Biotechnol. Bioeng., 2012, 109, 2093–2103 CrossRef CAS PubMed.
- R. J. Boado, E. K. Hui, J. Z. Lu and W. M. Pardridge, Biotechnol. Bioeng., 2011, 108, 186–196 CrossRef CAS PubMed.
- F. M. Wurm and C. J. Petropoulos, Biologicals: journal of the International Association of Biological Standardization, 1994, 22, 95–102 CAS.
- N. S. Kim, T. H. Byun and G. M. Lee, Biotechnol. Prog., 2001, 17, 69–75 CrossRef CAS PubMed.
- J. Chusainow, Y. S. Yang, J. H. Yeo, P. C. Toh, P. Asvadi, N. S. Wong and M. G. Yap, Biotechnol. Bioeng., 2009, 102, 1182–1196 CrossRef CAS PubMed.
- D. S. Abramian and O. K. Glebov, Tsitologiia, 1981, 23, 1031–1040 CAS.
- W. Pilbrough, T. P. Munro and P. Gray, PLoS One, 2009, 4, e8432 Search PubMed.
- R. G. Worton, C. C. Ho and C. Duff, Somatic Cell Genet., 1977, 3, 27–45 CrossRef CAS PubMed.
- C. S. Kaas, C. Kristensen, M. J. Betenbaugh and M. R. Andersen, BMC Genomics, 2015, 16, 160 CrossRef PubMed.
- J. H. Nunberg, R. J. Kaufman, R. T. Schimke, G. Urlaub and L. A. Chasin, Proc. Natl. Acad. Sci. U. S. A., 1978, 75, 5553–5556 CrossRef CAS.
- R. J. Kaufman, P. C. Brown and R. T. Schimke, Proc. Natl. Acad. Sci. U. S. A., 1979, 76, 5669–5673 CrossRef CAS.
- M. G. Pallavicini, P. S. DeTeresa, C. Rosette, J. W. Gray and F. M. Wurm, Mol. Cell. Biol., 1990, 10, 401–404 CrossRef CAS PubMed.
- F. M. Wurm, M. G. Pallavicini and R. Arathoon, Dev. Biol. Stand., 1992, 76, 69–82 CAS.
- T. Yoshikawa, F. Nakanishi, S. Itami, D. Kameoka, T. Omasa, Y. Katakura, M. Kishimoto and K. Suga, Cytotechnology, 2000, 33, 37–46 CrossRef CAS PubMed.
- T. F. Beckmann, O. Kramer, S. Klausing, C. Heinrich, T. Thute, H. Buntemeyer, R. Hoffrogge and T. Noll, Appl. Microbiol. Biotechnol., 2012, 94, 659–671 CrossRef CAS PubMed.
- V. Paredes, J. S. Park, Y. Jeong, J. Yoon and K. Baek, Biotechnol. Lett., 2013, 35, 987–993 CrossRef CAS PubMed.
- S. Hammond, J. C. Swanberg, S. W. Polson and K. H. Lee, Biotechnol. Bioeng., 2012, 109, 1371–1375 CrossRef CAS PubMed.
- J. Becker, M. Hackl, O. Rupp, T. Jakobi, J. Schneider, R. Szczepanowski, T. Bekel, N. Borth, A. Goesmann, J. Grillari, C. Kaltschmidt, T. Noll, A. Puhler, A. Tauch and K. Brinkrolf, J. Biotechnol., 2011, 156, 227–235 CrossRef CAS PubMed.
- C. Trapnell, A. Roberts, L. Goff, G. Pertea, D. Kim, D. R. Kelley, H. Pimentel, S. L. Salzberg, J. L. Rinn and L. Pachter, Nat. Protoc., 2012, 7, 562–578 CrossRef CAS PubMed.
- Z. Wang, M. Gerstein and M. Snyder, Nat. Rev. Genet., 2009, 10, 57–63 CrossRef CAS PubMed.
- J. C. Yee, K. F. Wlaschin, S. H. Chuah, P. M. Nissom and W. S. Hu, Biotechnol. Bioeng., 2008, 101, 1359–1365 CrossRef CAS PubMed.
- X. Xu, H. Nagarajan, N. E. Lewis, S. Pan, Z. Cai, X. Liu, W. Chen, M. Xie, W. Wang, S. Hammond, M. R. Andersen, N. Neff, B. Passarelli, W. Koh, H. C. Fan, J. Wang, Y. Gui, K. H. Lee, M. J. Betenbaugh, S. R. Quake, I. Famili, B. O. Palsson and J. Wang, Nat. Biotechnol., 2011, 29, 735–741 CrossRef CAS PubMed.
- Z. Siegfried and I. Simon, Wiley Interdiscip. Rev.: Syst. Biol. Med., 2010, 2, 362–371 CrossRef CAS PubMed.
- Y. Yang, Mariati, J. Chusainow and M. G. Yap, J. Biotechnol., 2010, 147, 180–185 CrossRef CAS PubMed.
- A. Osterlehner, S. Simmeth and U. Gopfert, Biotechnol. Bioeng., 2011, 108, 2670–2681 CrossRef CAS PubMed.
- G. Dennis, Jr., B. T. Sherman, D. A. Hosack, J. Yang, W. Gao, H. C. Lane and R. A. Lempicki, Genome Biol., 2003, 4, P3 CrossRef.
- D. W. Huang, B. T. Sherman and R. A. Lempicki, Nat. Protoc., 2009, 4, 44–57 CrossRef CAS PubMed.
- D. W. Huang, B. T. Sherman and R. A. Lempicki, Nucleic Acids Res., 2009, 37, 1–13 CrossRef CAS PubMed.
- A. J. George, W. G. Thomas and R. D. Hannan, Nat. Rev. Cancer, 2010, 10, 745–759 CrossRef CAS PubMed.
- M. Klauzinska, N. P. Castro, M. C. Rangel, B. T. Spike, P. C. Gray, D. Bertolette, F. Cuttitta and D. Salomon, Semin. Cancer Biol., 2014, 29, 51–58 CrossRef CAS PubMed.
- S. L. Thomas, R. Alam, N. Lemke, L. R. Schultz, J. A. Gutierrez and S. A. Rempel, Neuro-Oncology, 2010, 12, 941–955 CrossRef CAS PubMed.
- M. Rhinn and P. Dolle, Development, 2012, 139, 843–858 CrossRef CAS PubMed.
- S. P. Becerra and V. Notario, Nat. Rev. Cancer, 2013, 13, 258–271 CrossRef CAS PubMed.
- A. Fleischer, V. Ayllon, L. Dumoutier, J. C. Renauld and A. Rebollo, Oncogene, 2002, 21, 3181–3189 CrossRef CAS PubMed.
- L. M. Barnes, C. M. Bentley and A. J. Dickson, Biotechnol. Bioeng., 2003, 81, 631–639 CrossRef CAS PubMed.
- K. Bidet, D. Dadlani and M. A. Garcia-Blanco, PLoS Pathog., 2014, 10, e1004242 Search PubMed.
- M. M. Kim, D. Wiederschain, D. Kennedy, E. Hansen and Z. M. Yuan, Oncogene, 2007, 26, 4209–4215 CrossRef CAS PubMed.
- K. Taniuchi, I. Nishimori and M. A. Hollingsworth, Cancer Res., 2011, 71, 895–905 CrossRef CAS PubMed.
- H. Matsuki, M. Takahashi, M. Higuchi, G. N. Makokha, M. Oie and M. Fujii, Genes to cells: devoted to molecular & cellular mechanisms, 2013, 18, 135–146 CAS.
- T. Kobayashi, S. Winslow, L. Sunesson, U. Hellman and C. Larsson, PLoS One, 2012, 7, e35820 CAS.
- E. Guitard, F. Parker, R. Millon, J. Abecassis and B. Tocque, Cancer Lett., 2001, 162, 213–221 CrossRef CAS PubMed.
- H. Zhang, S. Zhang, H. He, W. Zhao, J. Chen and R. G. Shao, Cancer Sci., 2012, 103, 1848–1856 CrossRef CAS PubMed.
- B. Culjkovic-Kraljacic, A. Baguet, L. Volpon, A. Amri and K. L. Borden, Cell Rep., 2012, 2, 207–215 CrossRef CAS PubMed.
- M. Hamada, A. Haeger, K. B. Jeganathan, J. H. van Ree, L. Malureanu, S. Walde, J. Joseph, R. H. Kehlenbach and J. M. van Deursen, J. Cell Biol., 2011, 194, 597–612 CrossRef CAS PubMed.
- J. Pan, S. Eckardt, N. A. Leu, M. G. Buffone, J. Zhou, G. L. Gerton, K. J. McLaughlin and P. J. Wang, Dev. Biol., 2009, 330, 167–174 CrossRef CAS PubMed.
- J. Katahira, T. Miki, K. Takano, M. Maruhashi, M. Uchikawa, T. Tachibana and Y. Yoneda, Nucleic Acids Res., 2008, 36, 616–628 CrossRef CAS PubMed.
- I. Tretyakova, A. S. Zolotukhin, W. Tan, J. Bear, F. Propst, G. Ruthel and B. K. Felber, J. Biol. Chem., 2005, 280, 31981–31990 CrossRef CAS PubMed.
- T. C. Hsu, Int. Rev. Cytol., 1961, 12, 69–161 CAS.
- F. M. Wurm, Processes, 2013, 1, 296–311 CrossRef CAS.
- K. J. Livak and T. D. Schmittgen, Methods, 2001, 25, 402–408 CrossRef CAS PubMed.
- D. Li, K. Xie, G. Ding, J. Li, K. Chen, H. Li, J. Qian, C. Jiang and J. Fang, Cancer Lett., 2014, 346, 45–52 CrossRef CAS PubMed.
- C. Trapnell, B. A. Williams, G. Pertea, A. Mortazavi, G. Kwan, M. J. van Baren, S. L. Salzberg, B. J. Wold and L. Pachter, Nat. Biotechnol., 2010, 28, 511–515 CrossRef CAS PubMed.
Footnote |
† Electronic supplementary information (ESI) available. See DOI: 10.1039/c5mb00627a |
|
This journal is © The Royal Society of Chemistry 2016 |
Click here to see how this site uses Cookies. View our privacy policy here.