DOI:
10.1039/C5MB00492F
(Paper)
Mol. BioSyst., 2016,
12, 133-144
Discovery of urinary metabolomic biomarkers for early detection of acute kidney injury†
Received
21st July 2015
, Accepted 2nd November 2015
First published on 2nd November 2015
Abstract
The discovery of new biomarkers for early detection of drug-induced acute kidney injury (AKI) is clinically important. In this study, sensitive metabolomic biomarkers identified in the urine of rats were used to detect cisplatin-induced AKI. Cisplatin (10 mg kg−1, i.p.) was administered to Sprague-Dawley rats, which were subsequently euthanized after 1, 3 or 5 days. In cisplatin-treated rats, mild histopathological alterations were noted at day 1, and these changes were severe at days 3 and 5. Blood urea nitrogen (BUN) and serum creatinine (SCr) levels were significantly increased at days 3 and 5. The levels of new urinary protein-based biomarkers, including kidney injury molecule-1 (KIM-1), glutathione S-transferase-α (GST-α), tissue inhibitor of metalloproteinase-1 (TIMP-1), vascular endothelial growth factor (VEGF), calbindin, clusterin, neutrophil, neutrophil gelatinase-associated lipocalin (NGAL), and osteopontin, were significantly elevated at days 3 and 5. Among urinary metabolites, trigonelline and 3-indoxylsulfate (3-IS) levels were significantly decreased in urine collected from cisplatin-treated rats prior to histological kidney damage. However, carbon tetrachloride (CCl4), a hepatotoxicant, did not affect these urinary biomarkers. Trigonelline is closely associated with GSH depletion and results in insufficient antioxidant capacity against cisplatin-induced AKI. The predominant cisplatin-induced AKI marker appeared to be reduced in urinary 3-IS levels. Because 3-IS is predominantly excreted via active secretion in proximal tubules, a decrease is indicative of tubular damage. Further, urinary excretion of 3-IS levels was markedly reduced in patients with AKI compared to normal subjects. The area under the curve receiver operating characteristics (AUC-ROC) for 3-IS was higher than for SCr, BUN, lactate dehydrogenase (LDH), total protein, and glucose. Therefore, low urinary or high serum 3-IS levels may be more useful for early detection of AKI than conventional biomarkers.
1. Introduction
Acute kidney injury (AKI), defined as a rapid decline in glomerular filtration rate (GFR), may be associated with a higher mortality rate in patients. Despite considerable advances in nephrology and medical care over the past decade, protective mechanisms underlying AKI are still not clearly understood.1 AKI is a limiting factor in the development of new drugs that are needed for treating various disorders.2 Thus, new and more effective biomarkers are required for early detection of AKI.
Serum creatinine (SCr) and blood urea nitrogen (BUN) are the most routinely used clinical parameters for detecting AKI. These conventional nephrotoxicity biomarkers are not sufficiently sensitive for indicating the presence of renal damage, however, because alterations in SCr and BUN only become apparent after severe renal epithelial mass loss has already occurred.3,4 Changes in SCr and BUN are not specific for AKI. They are influenced by a variety of other non-renal factors, such as age, muscle mass, and diet, which hinders the diagnosis of AKI.5,6 Recently, several alternatives to SCr and BUN were proposed for detecting nephrotoxicity.7,8 Protein-based biomarkers in urine, such as total protein, kidney injury molecule-1 (KIM-1), clusterin, and cystatin C, have been suggested as new biomarkers of renal damage by the Food and Drug Administration (FDA) and the European Medicines Agency (EMEA).9,10 There have been attempts to find reliable biomarkers that provide a more rapid and sensitive means of detecting AKI. The FDA and EMEA considered several urinary proteins as novel biomarkers that may serve as important tools for early detection of drug-induced kidney injury. This directive was based upon proposed biomarkers submitted by the Predictive Safety Testing Consortium (PSTC), comprised of scientists from 16 pharmaceutical companies.11 It may be possible for these biomarkers to be used as indicators of whole-organ nephrotoxicity and also to determine the specific renal site and degree of damage. The use of these new biomarkers may prove beneficial in facilitating early diagnosis, guiding targeted intervention, and monitoring disease progression and resolution.12
Metabolomics is an emerging bioanalytical field that offers the potential to understand disease states and elucidate mechanisms underlying physiological and metabolic pathways involved in drug-induced toxicity.13–18 Because of greater stability and species independence compared with protein-based methods, metabolomics provides a means to identify novel biomarkers for early, reliable detection of nephrotoxicity that may be preferable to methods based on protein data.19–21 The aim of the present study was to identify new biomarkers for early detection of nephrotoxicity in the urine of rats treated with cisplatin, a known nephrotoxicant.22,23 The sensitivity of conventional biomarkers was compared to that of new metabolic and protein-based urinary biomarkers. To determine whether the cisplatin-induced effects on these biomarkers are tissue-specific, the cisplatin-mediated effects were compared to those produced by carbon tetrachloride (CCl4), a known hepatotoxicant.21
2. Experimental section
2.1. Chemicals
Cisplatin and CCl4 were purchased from Sigma-Aldrich (St. Louis, MO, USA). 3-(Trimethylsilyl) propionic-2,2,3,3-d4 acid (TSP), imidazole, and sodium azide were also purchased from Sigma-Aldrich (St. Louis, MO, USA).
2.2. Animals and husbandry
Male Sprague-Dawley rats (weighing approximately 180 g, 6 weeks old) were purchased from Charles River Laboratories (Orient, Seoul, Korea). Animals were housed under controlled temperatures (22 ± 2 °C) and relative humidity (50–60%) with a 12 h light–dark cycle. The animals were provided with food (PMI, Brentwood, MO, USA) and tap water ad libitum. Rats were acclimated for 1 week prior to use. They were randomly allocated to control and drug treatment groups with 6 rats per group. The animal protocol used in this study was approved for ethical procedures and scientific care by the Pusan National University-Institutional Animal Care and Use Committee (Approval Number PNU-2012-00211).
2.3. Experimental design
The cisplatin-mediated AKI model is a method involving intraperitoneal injection of cisplatin at 10 mg kg−1 to produce AKI 3–5 days following treatment.22 In this study, animals were injected with cisplatin (10 mg kg−1 body weight, ip, dissolved in 0.9% saline) or CCl4 (0.5 mg kg−1 body weight, ip, dissolved in 0.9% saline) while vehicle control received 0.9% saline. The animals were anesthetized with carbon dioxide 1, 3, or 5 days following cisplatin treatment. For CCl4-treated animals, euthanasia with carbon dioxide also occurred after 1, 3, or 5 days. Blood was collected from the abdominal aorta in heparinized tubes, and plasma samples were separated by centrifugation at 3000g for 15 min. Samples were immediately stored at −80 °C and kept until analysis. After blood collection, both kidneys (right and left) were excised. The left kidney was immediately frozen in liquid nitrogen and stored at −80 °C. The right kidney was fixed overnight in 10% neutral formalin and dehydrated in 70% ethanol. Urine samples were collected from animals at 1, 3, and 5 days. Each animal was kept in a rat metabolic cage overnight, and urine samples were collected every 24 h in the morning. Urine samples were immediately centrifuged at 1500g for 15 min and stored in aliquots at −80 °C for subsequent analysis.
2.4. Histopathological examination
After euthanasia, the right kidney and liver were fixed overnight in 10% neutral buffered formalin and dehydrated with 70% ethanol. Each tissue was embedded in paraffin and 5 μm sections were cut and mounted on slides. Slide sections were stained with hematoxylin and eosin (H&E). The slides were histologically processed and microscopically examined. Photomicrographs of the kidney were taken at 200× magnification using a Zeiss Axiphot light microscope (Zeiss, Oberkochen, Germany) fitted with a Sony 3CCD camera (AVT Horn, Aalen, Germany).
2.5. Clinical chemistry
For clinical chemistry measurements, serum creatinine (SCr), blood urea nitrogen (BUN), and serum glucose levels were measured using the VetScan analyzer (Abaxis, lnc. Union City, CA, USA). Levels of urinary glucose, lactate dehydrogenase (LDH), and protein were determined using a Vitros 700XR (Ortho-Clinical Diagnostics, Neckarsgemuend, Germany) at the Laboratory for Clinical Chemistry of the Medical School, Pusan National University.
2.6. Detection of newly developed urinary biomarkers
The evaluation of novel urinary kidney markers was performed using a WideScreen™ Rat Kidney Toxicity Panel 1 and 2. This panel was based on an immunosandwich assay immobilized on microparticle beads and used according to the manufacturer's instructions, as described by Hoffmann et al.24
2.7. Western blot analysis
Preserved urine samples were mixed with an activation/wash buffer, and the resulting slurry containing bound proteins was loaded onto a Mini Filter Spin Column (Norgen, Biotec Corp., Thorold, ON, Canada). The bound proteins were washed to remove any remaining impurities. Finally, purified total urine proteins were dissolved in 150–300 μl of the provided elution buffer. The method employed removes highly concentrated salts and metabolic wastes to allow isolation of high-quality proteins. Purified urinary proteins (20 μg) were loaded onto 10–15% SDS–polyacrylamide (PAGE) gels. After electrophoresis, the proteins were transferred to a polyvinylidene difluoride (PVDF) membrane (Millipore, Billerica, MA, USA). The membranes were incubated for 1 h in TNA (10 mM Tris-Cl, pH-7.6, 100 mM NaCl, and 0.5% Tween 20) buffer containing 5% skim milk and then incubated with different primary antibodies at 4 °C overnight. Reactive bands were revealed using an enhanced chemiluminescence (ECL)-plus kit (Amersham Biosciences, Buckinghamshire, UK).
2.8.
1H NMR spectroscopy and data processing
The analysis of urine samples was carried out using equipment purchased from Varian (Varian Inc., Palo Alto, CA, UAS) at a working frequency of 600.167 MHz and temperature of 299.1 K. Deuterium oxide and TSP-d4 were used for field frequency locking and internal chemical shift references, respectively. Single-pulse spectra were acquired using a CPMG (Carr-Purcell-Meiboom-Gill) pulse sequence to suppress the water signal. Each 1H NMR spectrum was recorded at 128 scans into 32 K data points, which resulted in an acquisition time of 12 min. All data were apodized automatically with an exponential function using line broadening of 0.2 Hz prior to Fourier transformation. Data were then calibrated to TSP-d4 at δ 0.00 ppm. Spectral assignment was performed by Chenomx NMR Suite 7.1 software (Chenomx Inc., Edmonton, Canada) and compared with published data. After processing, data were reduced into 920 spectral integral regions corresponding to a chemical shift range of δ 0.2–10 ppm using the Chenomx NMR Suite, as described previously.15,18
2.9. Multivariate data analysis
Principal component analysis (PCA) was initially used to identify and remove outliers from analysis, and partial least squares-discriminant analysis (PLS-DA) was applied to maximize the separation between groups using SIMCA-P (version 11, Umetrics, Umeå, Sweden). Multivariate statistical data analysis, including unsupervised principal component analysis (PCA) was performed using SIMCA-P (version 11, Umetrics, Umeå, Sweden). PCA is an exploratory unsupervised pattern recognition method that transforms a set of observations of possibly correlated variables into a set of linearly uncorrelated variables called principal components (PCs). This was used to provide an overview of the dataset and to aid in the identification of metabolic alterations due to the injury. The statistical confidence level was 95% and significance level for distance to model (DModX) and Hotelling's T2 was 0.05. Center (Ctr) scaling was selected before fitting. Cross validation groups are based on every Nth observation assigned to the same group.
2.10. Urine collection from normal and AKI patients
Seven AKI patients were studied for detection of urinary 3-IS levels. Protein biomarkers were also measured in the urine of seven patients with AKI and 5 control individuals with no apparent evidence of renal disease. The seven patients with various causes of acute renal failure were randomly selected from patients seen for renal consultation at Korea University (Seoul, Korea) Hospital from June to July 2012. Ten ml urine was collected and then centrifuged at 1000g for 5 min. The supernatants were stored at −80 °C.
2.11. LC-MS/MS conditions and sample preparation
A 50 μl aliquot of biological sample was deproteinized with 150 μl aliquot acetonitrile containing 1 μg ml−1 of LDD966 (internal standard). The mixture was vortexed for 30 s and centrifuged for 10 min at 10
000g. Then, 2 μl supernatant was injected into HPLC. The LC-MS/MS system used was an UltiMate® 3000 LC unit (Thermo Fisher Scientific Inc., Waltham MA, USA) connected to an API3200 quadruple MS/MS unit (AB Sciex Inc., Framingham, MA, USA). System control and data analysis were carried out using Analyst software (Version 1.5.0, AB Sciex Inc.). Biological samples were analyzed by HPLC using a Gemini-NX C18 (Phenomenex, Torrance, CA, USA) with a 100 mm × 2 mm, 3 μm particle size main column. The mobile phases were (A) purified water containing 0.1% formic acid and (B) acetonitrile containing 0.1% formic acid. The flow rate was 0.3 ml min−1, with a gradient starting at 80% of A that decreased linearly to 5% within 1 min and was then held for 0.5 min. The injection volume was 2 μl. The column oven was maintained at 50 °C and the run time was 8 min. Multiple reaction monitoring (MRM) transitions and collision energy (eV) for 3-IS and LDD966 (internal standard) were m/z 211.8 → 80.0 (−34 eV) and 327.9 → 298.1 (−30 eV), respectively. Scan and dwell times were 1.2 and 0.4 s, respectively. MS analysis was performed by electrospray ionization (ESI) interface in negative ion mode with a spray voltage of −4500 V at 400 °C.
2.12. Statistical analysis
All data were expressed as means ± SD of at least three independent experiments. Statistical analysis was performed using one-way analysis of variance (ANOVA) followed by Bonferroni's multiple comparison tests in the StatView 5.0.1 software package (SAS Inc., Cary, NC, USA). Receiver-operator characteristic (ROC) curves for each individual study were plotted using the Graph Pad Prism 5 software package (GraphPad Software Inc., La Jolla, CA, USA). A *p-value < 0.05 was considered statistically significant.
3. Results
3.1. Effects on clinical chemistries
Following cisplatin treatment, serum BUN, SCr, and glucose levels significantly increased from day 3 to 5 (Fig. 1A). In contrast, CCl4 did not markedly affect BUN, SCr, or glucose levels. In cisplatin-treated rats, AST and ALT enzyme levels were unchanged at all times compared with the control (Fig. 1C). Significant elevation in serum AST and ALT activities were observed 1 day following CCl4 treatment, but enzyme activities returned to control levels at days 3 and 5. These findings correlated with histological observations in the liver, where lesions were primarily detectable on day 1 following CCl4 treatment (Fig. 2B). As shown in Table 1, significant changes were observed in urinary parameters following cisplatin treatment. Levels of urinary total protein and glucose were markedly increased at days 1, 3, and 5. Enhanced excretion of urinary LDH was observed only after day 3. In contrast, elevation in urinary total protein at day 5 was the only significant change noted in urinary biomarkers following CCl4 treatment.
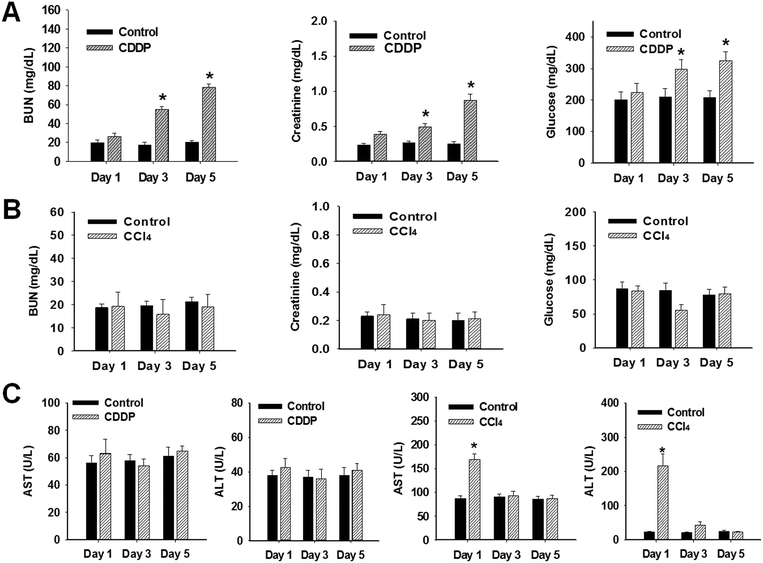 |
| Fig. 1 Effect of cisplatin or CCl4 on biochemical parameters. Male Sprague-Dawley rats were injected with cisplatin (10 mg kg−1) or CCl4 (0.5 mg kg−1) and euthanized at indicated time points. (A) Blood urea nitrogen (BUN), serum creatinine (SCr), and glucose levels in serum of rats at 1, 3, or 5 days after cisplatin treatment. (B) BUN, creatinine, and glucose levels in serum of rats at 1, 3, or 5 days after CCl4 treatment. (C) Serum aspirate aminotransferase (AST) and alanine aminotransferase (ALT) activity levels in rats at 1, 3, or 5 days after cisplatin or CCl4 treatment. Data are represented as mean values of individual animals. Statistical analysis was performed by Bonferroni's multiple comparison test. Statistically significant changes compared with controls are indicated as *p < 0.05. | |
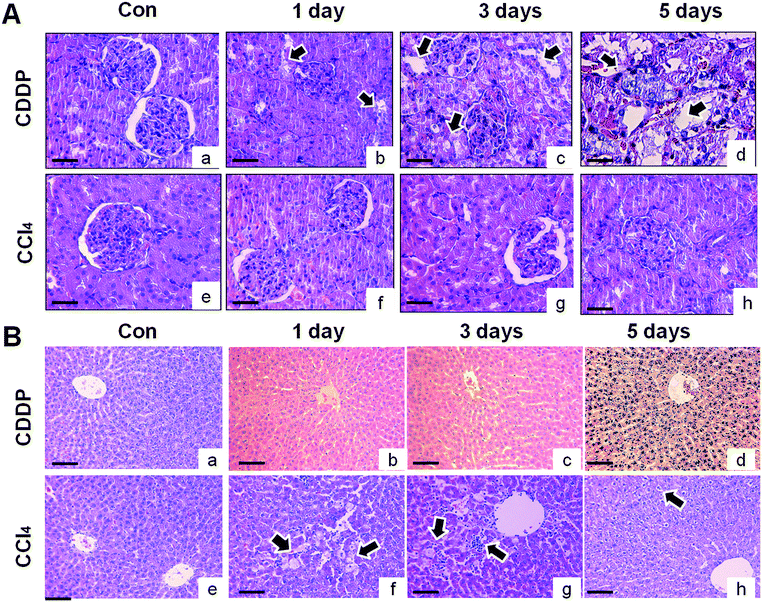 |
| Fig. 2 Kidney and liver histopathology after cisplatin or CCl4 treatment. Male Sprague-Dawley rats were injected with cisplatin (10 mg kg−1) or CCl4 (0.5 mg kg−1). After indicated time points, the right kidney and liver were collected for hematoxylin & eosin (H&E) staining. (Aa and Ae) Representative histology of control kidney. (Ab) Cisplatin treatment produced mild or no changes after 1 day. (Ac) Loss of tubular cells, tubular dilation, and cytoplasm shrinking were observed in tubular cells after 3 days. (Ad) Tubular degeneration and severe necrosis occurred in some parts of tubules after 3 days. (Af–Ah) CCl4 did not induce any lesions in the kidney at any time point. Arrow indicates loss of tubular cells and tubular degeneration in the cortex. (B) Representative histology of livers in Sprague-Dawley rats treated with cisplatin (Ba–Bd) or CCl4 (Be–Bh). (Bf) Centrilobular liver region at day 1 after administration of CCl4 (0.5 mg kg−1) was changed, with hepatocellular necrosis and vacuolation. (Bg) Immune cell proliferation and mild clear cell foci occurred by day 3, (Bh) but there was no liver injury apparent at day 5. Original magnification ×200. | |
Table 1 Changes in body weight and urinary parameters in response to cisplatin and CCl4 treatment
|
Nephrotoxicant |
Hepatotoxicant |
Day 1 |
Day 3 |
Day 5 |
Day 1 |
Day 3 |
Day 5 |
Con |
CDDP |
Con |
CDDP |
Con |
CDDP |
Con |
CCl4 |
Con |
CCl4 |
Con |
CCl4 |
Male Sprague-Dawley rats were injected with cisplatin (10 mg kg−1) or CCl4 (0.5 mg kg−1). After the indicated time points, each parameter was measured as described in Experimental section. Data are presented as mean ± SD. The statistical analysis was performed by ANOVA followed by Bonferroni's multiple comparison tests. Statistically significant changes compared with controls are indicated as *p < 0.05 and **p < 0.01. |
Final body weight (g) |
214.65 ± 3.03 |
227.47 ± 2.55 |
225.20 ± 2.88 |
229.57 ± 2.06 |
231.48 ± 2.06 |
231.48 ± 2.06 |
214.30 ± 5.44 |
215.24 ± 4.63 |
222.06 ± 2.97 |
221.20 ± 2.62 |
249.54 ± 5.12 |
251.93 ± 7.68 |
Urine volume (ml) |
7.07 ± 0.60 |
12.83 ± 2.00* |
7.75 ± 1.62 |
9.60 ± 0.69* |
9.60 ± 0.69* |
9.60 ± 0.69* |
7.50 ± 0.42 |
7.22 ± 0.30 |
7.75 ± 0.41 |
8.00 ± 0.40 |
8.42 ± 0.42 |
7.65 ± 0.20 |
Urine pH |
6.58 ± 0.07 |
6.88 ± 0.10 |
6.78 ± 0.16 |
7.08 ± 0.30 |
7.08 ± 0.30 |
7.08 ± 0.30 |
6.75 ± 0.17 |
6.67 ± 0.21 |
6.92 ± 0.24 |
7.00 ± 0.22 |
6.92 ± 0.15 |
7.08 ± 0.15 |
Creatinine (mg dl−1) |
0.25 ± 0.02 |
0.55 ± 0.07* |
0.28 ± 0.01 |
0.45 ± 0.14* |
0.27 ± 0.14 |
0.45 ± 0.14* |
0.26 ± 0.03 |
0.28 ± 0.04 |
0.24 ± 0.02 |
0.21 ± 0.01 |
0.24 ± 0.03 |
0.26 ± 0.03 |
BUN (mg dl−1) |
1508 ± 125 |
1212 ± 214 |
1418 ± 102 |
539 ± 37** |
1537 ± 165 |
357 ± 28** |
17.98 ± 0.64 |
18.76 ± 2.90 |
19.02 ± 1.20 |
14.36 ± 2.82 |
19.60 ± 1.01 |
17.94 ± 1.78 |
Total protein (mg dl−1) |
78.96 ± 8.41 |
112.37 ± 25.74 |
72.38 ± 9.36 |
156.33 ± 6.89** |
69.45 ± 11.34 |
212.56 ± 25.31** |
95.05 ± 14.37 |
68.24 ± 10.29 |
93.51 ± 9.75 |
67.32 ± 9.28 |
102.34 ± 8.71 |
201.45 ± 32.53* |
Glucose (mg dl−1) |
87.25 ± 9.83 |
254.37 ± 20.16 |
75.74 ± 6.32 |
1002.5 ± 36.94** |
71.31 ± 8.92 |
1564.3 ± 48.7** |
85.42 ± 6.34 |
80.12 ± 16.95 |
81.25 ± 8.76 |
63.08 ± 6.88 |
55.79 ± 6.95 |
68.25 ± 6.33 |
LDH (mg dL−1) |
10.35 ± 1.27 |
10.87 ± 2.43 |
14.52 ± 3.17 |
247.38 ± 29.65** |
15.78 ± 2.39 |
280.63 ± 31.25** |
10.09 ± 1.58 |
10.82 ± 1.27 |
7.21 ± 0.28 |
7.34 ± 1.59 |
7.29 ± 0.16 |
8.45 ± 1.05 |
3.2. Histopathological examinations of kidney and liver
A single cisplatin treatment produced morphological changes in the kidney after 1 day as evidenced by moderate inflammation and degeneration in proximal and distal tubules. However, the morphological changes were largely considered to be mild or unchanged (Fig. 2A). The severity of cisplatin-induced kidney injury was greater at day 3, including loss of tubular cells, tubular vacuolization, and cytoplasm shrinking in the proximal tubule. At day 5, tubular degeneration and severe necrosis occurred in some tubule portions. There were karyorrhectic tubular cells (Fig. 2A), but no marked alterations in glomeruli were noted. No renal lesions were observed in the vehicle control or CCl4-treated groups (Fig. 2A). At 1 day, CCl4 produced minimal hepatocellular vacuolation and single cell necrosis in the liver, as evidenced by a moderate increase in Kupffer cell number and fibrosis (Fig. 2B). Mild clear cell foci were observed at day 3, but there was no evidence of hepatic changes at day 5. These data indicate that the animals recovered from CCl4-induced hepatic injury by day 5. Cisplatin treatment did not produce any apparent hepatocellular injury, indicating tissue-specific effects on the kidney (Fig. 2B).
3.3. Changes in urinary protein-based biomarkers for nephrotoxicity
Cisplatin-treatment significantly increased urinary excretion of protein-based biomarkers (TIMP-1, VEGF, osteopontin, KIM-1, calbindin, clusterin, NGAL, and GST-α) in a time-dependent manner (Fig. 3A). No significant excretion in protein biomarkers was observed at day 1 after cisplatin injection. However, there was marked elevation in TIMP-1 (48.5-fold), KIM-1 (4.1-fold), calbindin, clusterin, NGAL (3.8-fold), and osteopontin levels in the urine of cisplatin-treated animals after 3 days (Fig. 3A). Levels of KIM-1 (4.1-fold), NGAL (3.8-fold) and TIMP-1 (48.5-fold) were significantly elevated at day 3. In contrast, no significant changes in urinary excretion of protein-based biomarkers were observed in CCl4-treated animals (Fig. 3A). Urinary excretion of these protein-based biomarkers was also confirmed by Western blot analysis, as shown in Fig. 3B.
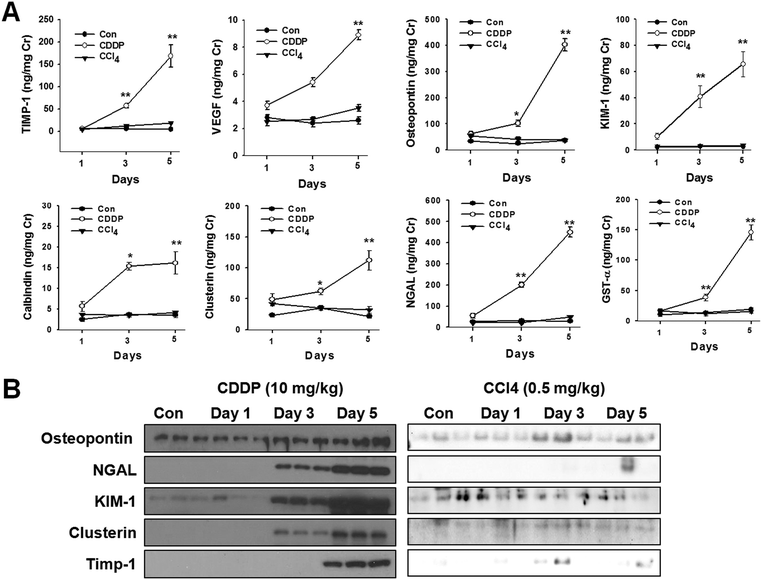 |
| Fig. 3 Changes in urinary biomarkers in Sprague-Dawley rats following cisplatin treatment. Male Sprague-Dawley rats were injected with cisplatin (10 mg kg−1) or CCl4 (0.5 mg kg−1) and rats were sacrificed after indicated time points. (A) Urine was collected and urinary excretion levels of biomarkers (VEGF, Timp-1, osteopontin, KIM-1, calbindin, clusterin, NGAL, and GST-alpha) were assayed using WideScreen™ Rat Kidney Toxicity Panel 1 and 2. The statistical significance of differences between control and treated groups was determined using Bonferroni's multiple comparison tests. *p < 0.05, **p < 0.01 at each sample point. (B) Urinary protein was isolated and urinary excretion of protein-based biomarkers was detected by Western blot analysis. Data are representative of three independent experiments. | |
3.4. Metabolomics and multivariate data analysis
1H NMR spectra were obtained from the urine of control or cisplatin-treated rats. Based on 1H NMR spectra, each metabolite was identified using ChenomX NMR suite 6.0 professional. A typical 1H NMR spectrum showing metabolites in urine is presented in Fig. 4A. The presence of metabolites such as trigonelline, 3-indoxyl sulfate (3-IS), hippurate, and cis-aconitate was determined. Several metabolites were decreased at day 1 to 3 relative to the control, including citrate, cis-aconitate, trigonelline, hippurate, and 3-indoxylsulfate (Table 2). Reduction in renal osmolytes, predominantly methylamine, was evident from day 1 to 3, and dimethlyamine levels rose at day 1 but fell significantly at day 3. Lower levels of Krebs cycle intermediates in urine, including fumarate, cis-aconitate, and citrate, were noted (Table 2). Citrate was markedly lower at day 1 and further reduced by day 3. Trigonelline, also known as N-methylnicotinate, was significantly decreased at both time points. Hippurate and 3-IS levels, which are generated by gut microflora, were significantly reduced at both days 1 and 3. In contrast, urinary excretion of glucose, acetate, pyroglutarate, alanine, lactate and formate was markedly elevated (ESI,† Fig. S1).
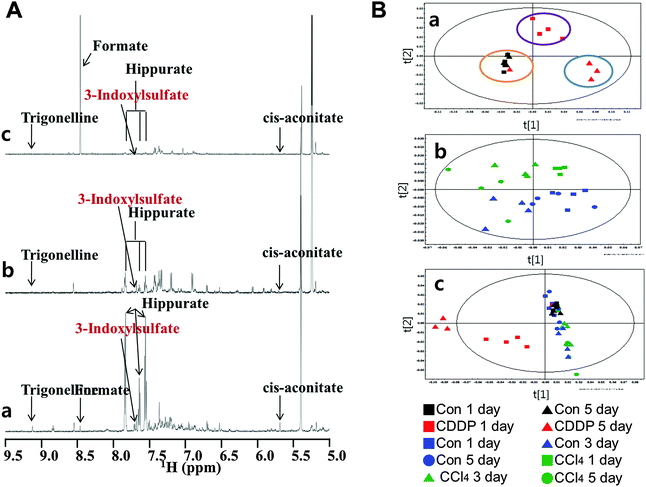 |
| Fig. 4 Representative series of 600 MHz single pulse 1H NMR spectra (δ 5.0–9.5) of urine obtained from control and cisplatin-treated (10 mg kg−1) rats. (Aa) Control at day 1, (Ab) day 1, and (Ac) day 3 after cisplatin treatment. (B) Principal component analysis of urinary 1H NMR spectra from control rats, cisplatin (10 mg kg−1), or CCl4 (0.5 mg kg−1,) groups. 1H NMR CA score plots of (a) cisplatin, (b) CCl4, (c) both of 1H NMR. | |
Table 2 Changes in urinary metabolites detected by 1H-NMR in Sprague-Dawley rats after cisplatin administration
|
Fold changes |
Statistical p value |
Super pathway |
Metabolite |
1 day |
3 day |
1 day |
3 day |
Male Sprague-Dawley rats were administered with saline (control) or cisplatin (10 mg kg−1) and urine samples were collected at the indicated time points. The analysis of metabolites in urine samples was carried out on a Varian (Varian Inc., Palo Alto, CA) with a working frequency of 600.167 MHz at a temperature of 299.1 K. Fold changes between cisplatin-treated and vehicle control group are shown, with their corresponding p values. |
Amino acid |
Tryptophan |
6.54↓ |
3.87↓ |
ND |
0.233 |
Amino acid |
Aspartate |
1.18↓ |
4.44↓ |
0.010 |
0.025 |
Peptide |
Glutathione |
1.97↓ |
7.45↓ |
0.139 |
0.011 |
TCA intermediates |
Fumarate |
1.98↓ |
21.54↓ |
0.069 |
0.070 |
TCA intermediates |
cis-Aconitate |
3.87↓ |
12.48↓ |
0.001 |
0.001 |
TCA intermediates |
Citrate |
5.24↓ |
65.33↓ |
0.001 |
0.001 |
Renal osmolytes |
Formate |
4.95↓ |
3.14↑ |
0.820 |
0.102 |
Renal osmolytes |
Dimethylamine |
1.13↑ |
14.65↓ |
0.010 |
0.002 |
Renal osmolytes |
Methylamine |
4.87↓ |
14.52↓ |
0.018 |
0.011 |
Gut microflora metabolites |
Trigonelline |
7.01↓ |
69.84↓ |
0.006 |
0.030 |
Gut microflora metabolites |
3-Indoxylsulfate |
15.43↓ |
87.43↓ |
0.001 |
ND |
Gut microflora metabolites |
Hippurate |
5.25↓ |
60.24↓ |
ND |
ND |
Gut microflora metabolites |
Lactate |
6.75↑ |
9.19↑ |
0.051 |
0.005 |
|
Creatinine |
3.25↓ |
11.45↓ |
ND |
ND |
CCl4 treatment exerted no marked effects on 3-IS or trigonelline levels at any time point. Following injection of CCl4, Krebs cycle intermediates citrate, trans-aconitate, and 2-oxoglutarate decreased over the course of the study, as reported previously.11,21 Hppurate, methylamine, and malonate levels were also reduced in animals following CCl4 treatment. Interestingly, formate and taurine were initially elevated on day 1 and 3, but decreased to less than control levels at day 5 (data not shown).
To further investigate differences between control, cisplatin, and CCl4 treatments, partial least-squares discriminant analysis (PLS-DA) model were constructed (Fig. 4B). A score plot of the PLS-DA model showed that cisplatin-treated group could be separated from the vehicle-treated group (Fig. 4B). Multivariate data analysis of 1H NMR showed clear separation of the controls from cisplatin-treated animals along the first principal component [1]. Similarly, urine samples from the cisplatin-treated groups were distinct in a time-dependent manner along the second principal component [2] plot. However, one of the cisplatin-treated animals at day 1 fell into the control cluster (Fig. 4Ba). The CCl4 treatment group demonstrated clear separation from the vehicle control group and animals treated from day 1 onwards along the discriminating principal component [2], except for one of the CCl4-treated animals at day 5. In the PCA model plot with cisplatin, control and CCl4 treatments, discriminative separation of cisplatin from the other groups was noted, but there was no apparent discrimination of control from CCl4 (Fig. 4B).
3.5. Changes in 3-IS level in rats
Based on metabolite profiling, 3-IS, which appeared to be the most affected by cisplatin, was quantified in the urine, left kidney, and serum using HPLC. Endogenous 3-IS concentrations in the urine, serum and left kidney treated with cisplatin are shown in Fig. 5A. Urinary excretion of 3-IS was significantly decreased in a time-dependent manner after cisplatin treatment. Significant elevation of 3-IS in the left kidney (8.6-fold) and serum (10.6-fold) was observed at 3 days after cisplatin treatment (Fig. 5A). In contrast, treatment with CCl4 did not produce a significant change in 3-IS levels in the urine, left kidney and serum (ESI,† Fig. S2).
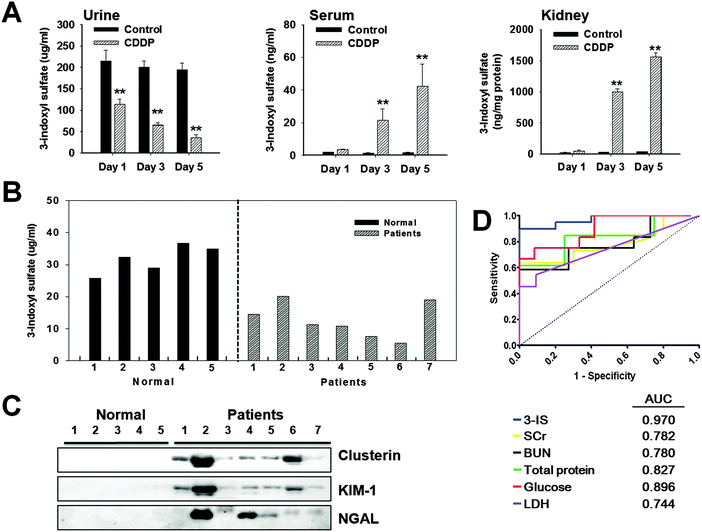 |
| Fig. 5 3-Indoxyl sulfate levels and urinary excretion of biomarkers in patients with acute kidney injury. (A) Male Sprague-Dawley rats were treated with cisplatin (10 mg kg−1) and 3-indoxyl sulfate (3-IS) levels were measured in urine, serum, and left kidney tissues at 1, 3, or 5 days after drug-treatment. Statistically significant changes compared with controls are indicated as **p < 0.01. (B) Changes in 3-IS level in the urine of control subjects and patients with acute kidney injury (AKI). (A) Black (control) and gray (AKI patients) bars represent individual levels of 3-IS. (C) Western blot analysis of urine collected from control subjects and patients with AKI. Each lane represents protein immunoextracted from urine of patients with AKI. Data are representative of three independent experiments. (D) Receiver operator characteristic (ROC) curve for candidate urinary biomarker (3-indoxyl sulfate) compared with conventional parameters. Area under the ROC curve, which serves as a measure of the overall ability of a biomarker to discriminate normal (control) versus diseased (treated) animals, is given in parentheses. | |
3.6. Changes in urinary excretion of 3-IS levels and urinary protein biomarkers in patients with AKI
The individual datum for changes in urinary excretion of 3-IS are shown in Fig. 5B. Urinary excretion of 3-IS was markedly decreased in patients with AKI compared with controls. The mean concentration of urinary 3-IS was markedly reduced in 7 patients, although the fall was numerically small in 2 patients. Correlation of 3-IS levels and excreted protein-based biomarkers was also compared in the urine of AKI patients and control subjects. As shown in Fig. 5C, urinary excretion of clusterin, KIM-1, NGAL, and HMGB1 was markedly increased in the urine of patients with AKI, but not in control subjects.
3.7. Receiver operating characteristic (ROC) analysis
ROC curves were plotted to compare the performance of the metabolite biomarker 3-IS to traditional biomarkers SCr and BUN as well as to urinary LDH, glucose, and total protein. The reliability and sensitivity of these biomarkers for detection of acute renal injury was investigated. These analyses demonstrated that 3-IS appeared to be a sensitive and reliable indicator of cisplatin-induced AKI (Fig. 5D).
4. Discussion
Cisplatin treatment produced tubular vacuolization and severe necrosis in some portions of rat kidney proximal tubules at days 3 and 5. These findings are in agreement with previously published observations on cisplatin-induced nephrotoxicity23,25 indicating that cisplatin as used in our study was an effective model for studying AKI. Histopathology was not useful as an early biomarker for renal damage, as the damage had already occurred.26 In the present study, cisplatin numerically increased levels of SCr and BUN. However, urinary parameters such as LDH activity and glucose levels were markedly elevated. It should be noted that SCr, BUN, urinary LDH activity, and glucose are not recognized as early sensitive biomarkers of injury.27–29 In addition to identifying early metabolomics biomarkers of renal injury, the tissue specificity of biomarkers was validated CCl4, a hepatoxicant. Data comparing the effects of cisplatin with CCl4 on clinical chemistries showed marked differences. While cisplatin increased SCr, BUN, and glucose in plasma, CCl4 did not markedly affect these serum and urinary parameters. Although cisplatin-induced effects were found to be tissue-specific, these biomarkers are not sufficiently sensitive to detect early signs of renal damage.
Cisplatin significantly increased total urinary proteins. Thus, it was of interest to determine which components of total protein were affected. Among urinary protein components, the protein biomarkers of kidney injury induced by cisplatin that were elevated included KIM-1, GST-α, TIMP-1, VEGF, calbindin, clusterin, NGAL, and osteopontin. Although significant elevations were noted in clusterin, NGAL, and KIM-1 at both 3 and 5 days after cisplatin treatment, marked urinary increases were eventually observed in all renal biomarkers. This indicates that clusterin, NGAL, and KIM-1 may be more sensitive indicators of nephrotoxicity than other markers measured in our study. It is of interest that clusterin, NGAL, and KIM-1 were previously characterized as early and sensitive protein biomarkers in urine.30–32 Urinary NGAL, clusterin, and KIM-1 emerged as sensitive biomarkers for the early detection of kidney injury in pediatric patients. Mishra et al.33 reported that urinary NGAL had a high AUC-ROC (0.99) level in many children after undergoing cardiopulmonary bypass surgery. This study also confirmed that urinary excretion levels of these protein-based biomarkers were markedly increased in patients with AKI. On the basis of clinical findings, KIM-1 represents a promising biomarker for early diagnosis of AKI and its clinical outcomes.34,35 Thus, it was postulated that these protein-based biomarkers are secreted into urine from damaged renal tissues.
Based on 1H NMR metabolic profiling, cisplatin treatment decreased citrate, methylamine, dimethylamine, hippurate, trigonelline, and 3-IS; however, this drug elevated lactate and formate. It is postulated that the increase in urinary lactate levels are related to mitochondrial damage, possibly as a consequence of impaired pyruvate oxidation36,37 or altered energy metabolism in proximal tubular cells.38 The Krebs cycle intermediates in urine were significantly lowered, including fumarate, cis-aconitate, and citrate. These findings are similar to those previously reported using cisplatin and other nephrotoxicants.39,40 The reduction in urinary excretion of the Krebs cycle intermediates citrate, 2-oxoglutarate, and fumarate may be a non-specific effect following toxic organ injury because both cisplatin and CCl4 reduced these metabolic constituents in urine. Another prominent change induced by both cisplatin and CCl4 treatment was marked reduction in hippurate levels. Changes in urinary TCA cycle intermediates and hippurate appear to be non-specific responses and may not be considered reliable biomarkers of renal disease. The metabolism of S-adenosyl-L-methionine (SAMe) into S-adenosyl homocysteine (SAH) results in the byproduct trigonelline. In our investigation, cisplatin treatment was found to lower urinary trigonelline and glutathione (GSH) levels. Trigonelline may be associated with alterations in the SAMe cycle as a result of depletion in the transsulfuration pathway for regenerating GSH. Sun et al.14 postulated that the fall in trigonelline levels may be related to decrease in GSH levels in the livers of acetaminophen-treated rats. Similarly, cisplatin-induced reduction in kidney and urine GSH levels in our study was associated with a fall in urinary trigonelline levels.
3-IS is a renal toxin that accumulates in the blood of uremic animals or patients as a consequence of decreased or absent urinary excretion of 3-IS, and is believed to be indicative of renal tubular injury.41 As early as day 1 (15-fold) and day 3 (87-fold), a marked fall in 3-IS excretion was noted following cisplatin treatment. This is especially important to identifying biomarkers, as a decrease in 3-IS preceded any evidence of apparent histopathological kidney damage. 3-IS is predominantly excreted via active secretion through the organic anion transporter (OAT) in proximal tubules42 and not via glomerular filtration, as 3-IS binding to albumin prevents from entering the glomerulus.43 Thus, lower levels of 3-IS noted in urine may be indicative of renal tubular damage, as demonstrated in our human study. Further investigation is required to determine the functional role of 3-IS in AKI patients. Thus, urinary 3-IS might be useful as a marker in clinical trials such that therapy may be initiated at an early stage in the course of AKI.
In conclusion, potential urinary biomarkers of AKI produced by cisplatin treatment were identified, and their usefulness as biomarkers for early detection of kidney damage was compared with traditional biomarkers. Following cisplatin treatment, some protein-based urinary kidney markers were elevated at day 1. All biomarkers examined in this study rose significantly by day 3, suggesting that these biomarkers may be used as sensitive and early indices of adverse effects. Data showed that 3-IS, which was significantly decreased in urine but elevated in kidneys and serum, was a specific, reliable, and sensitive biomarker for the detection of AKI. However, it is difficult at present to compare urinary protein biomarkers with 3-IS levels as early sensitive biomarkers of kidney damage because each of these parameters were similarly affected by cisplatin. Our results suggest that the metabolomics approach may provide a sensitive and early predictive tool for detecting drug-induced nephrotoxicity. However, combinations of multi-biomarkers may be more reliable and predictive for diagnosing nephrotoxicity. Future studies on the use of multiple biomarkers to determine AKI are needed.
Conflicts of interests
The authors declare they have no conflicts of interest.
Acknowledgements
This research was support by a grant (11182KFDA992-1203) from the Korea Food & Drug Administration and the National Research Foundation of Korea (NRF) grants funded by the Korean Government (2013R1A1A2008940). We also thank Dr Whan Sup Cho at Dong-a University (Injae, Korea) for evaluating histological examinations.
References
- Y. P. Ympa, Y. Sakr, K. Reinhart and J. L. Vincent, Has mortality from acute renal failure decreased? A systematic review of the literature, Am. J. Med., 2005, 118, 827–832 CrossRef PubMed.
- S. K. Thukral, P. J. Nordone, R. Hu, L. Sullivan, E. Galambos, V. D. Fitzpatrick, L. Healy, M. B. Bass, M. E. Cosenza and C. A. Afshari, Prediction of nephrotoxicant action and identification of candidate toxicity-related biomarkers, Toxicol. Pathol., 2005, 33, 343–355 CrossRef CAS PubMed.
- P. Espandiari, J. Zhang, B. Rosenzweig, V. S. Vaidya, J. Sun, L. Schnackenberg, E. H. Herman, A. Knapton, J. V. Bonventre, R. D. Beger, K. L. Thompson and J. Hanig, The utility of a rodent model in detecting pediatric drug-induced nephrotoxicity, Toxicol. Sci., 2007, 99, 637–648 CrossRef CAS PubMed.
- Y. Zhou, V. S. Vaidya, R. P. Brown, J. Zhang, B. A. Rosenzweig, K. L. Thompson, T. J. Miller, J. V. Bonventre and P. L. Goering, Comparison of kidney injury molecule-1 andother nephrotoxicity biomarkers in urine and kidney following acute exposure togentamicin, mercury, and chromium, Toxicol. Sci., 2008, 101, 159–170 CrossRef CAS PubMed.
- S. M. Moran and B. D. Myers, Course of acute renal failure studied by a model of creatinine kinetics, Kidney Int., 1985, 27, 928–937 CrossRef CAS PubMed.
- R. A. Star, Treatment of acute renal failure, Kidney Int., 1998, 54, 1817–1831 CrossRef CAS PubMed.
- M. Schmid, D. Dalela, R. Tahbaz, J. Langetepe, M. Randazzo, R. Dahlem, M. Fisch, Q. D. Trinh and F. K. Chun, Novel biomarkers of acute kidney injury: evaluation and evidence in urologic surgery, World J. Nephrol., 2015, 4, 160–168 CrossRef PubMed.
- M. E. Wasung, L. S. Chawla and M. Madero, Biomarkers of renal function, which and when?, Clin. Chim. Acta, 2015, 438, 350–357 CrossRef CAS PubMed.
- Food and Drug Administration (FDA), European medicines agency to consider additional test results when assessing new drug Safety, 2008, http://www.fda.gov/NewsEvents/Newsroom/PressAnnouncements/2008/ucm116911.htm.
- European Medicines Agency (EMEA), Final report on the pilot joint EMEA/FDA VXDs experience on qualification of nephrotoxicity biomarkers, 2008, http://www.emea.europa.eu/pdfs/human/biomarkers/25088508en.pdf.
- Food and Drug Administration (FDA), Predictive safety testing consortium (PSTC), 2009, http://www.fda.gov/oc/initiatives/criticalpath/projectsummary/consortium.html.
- V. S. Vaidya, M. A. Ferguson and J. V. Bonventre, Biomarkers of acute kidney injury, Annu. Rev. Pharmacol. Toxicol., 2008, 48, 463–493 CrossRef CAS PubMed.
- D. G. Robertson, M. D. Reily, R. E. Sigler, D. F. Wells, D. A. Paterson and T. K. Braden, Metabonomics: evaluation of nuclear magnetic resonance (NMR) and pattern recognition technology for rapid in vivo screening of liver and kidney toxicants, Toxicol. Sci., 2000, 57, 326–337 CrossRef CAS PubMed.
- J. Sun, L. K. Schnackenberg, R. D. Holland, T. C. Schmitt, G. H. Cantor, Y. P. Dragan and R. D. Beger, Metabonomics evaluation of urine from rats given acute and chronic doses of acetaminophen using NMR and UPLC/MS, J. Chromatogr. B: Anal. Technol. Biomed. Life Sci., 2008, 871, 328–340 CrossRef CAS PubMed.
- T. H. Kim, M. Y. Ahn, H. J. Lim, Y. J. Lee, Y. J. Shin, U. De, J. Lee, B. M. Lee, S. Kim and H. S. Kim, Evaluation of metabolomic profiling against renal toxicity in Sprague-Dawley rats treated with melamine and cyanuric acid, Arch. Toxicol., 2012, 86, 1885–1897 CrossRef CAS PubMed.
- U. Helena, H. U. Zacharias, G. Schley, J. Hochrein, M. S. Klein, C. Köberle, K.-U. Eckardt, C. Willam, P. J. Oefner and W. Gronwald, Analysis of human urine reveals metabolic changes related to the development of acute kidney injury following cardiac surgery, Metabolomics, 2013, 9, 697–707 CrossRef.
- S. Grison, G. Favé, M. Maillot, L. Manens, O. Delissen, E. Blanchardon, N. Banzet, C. Defoort, R. Bott, L. Dublineau, J. Aigueperse, P. Gourmelon, J. C. Martin and M. Souidi, Metabolomics identifies a biological response to chronic low-dose natural uranium contamination in urine samples, Metabolomics, 2013, 9, 1168–1180 CrossRef CAS PubMed.
- Y. K. Lee, E. Y. Park, S. Kim, J. Y. Son, T. H. Kim, G. W. Kang, T. C. Jeong, K. B. Kim, S. J. Kwack, J. Lee, S. Kim, B. M. Lee and H. S. Kim, Evaluation of cadmium-induced nephrotoxicity using urinary metabolomic profiles in Sprague-Dawley male rats, J. Toxicol. Environ. Health, Part A, 2014, 77, 1384–1398 CrossRef CAS PubMed.
- M. A. Ferguson, V. S. Vaidya and J. V. Bonventre, Biomarkers of nephrotoxic acute kidney injury, Toxicology, 2008, 245, 182–193 CrossRef CAS PubMed.
- K. J. Boudonck, M. W. Mitchell, L. Német, L. Keresztes, A. Nyska, D. Shinar and M. Rosenstock, Discovery of metabolomics biomarkers for early detection of nephrotoxicity, Toxicol. Pathol., 2009, 37, 280–292 CrossRef CAS PubMed.
- R. D. Beger, J. Sun and L. K. Schnackenberg, Metabolomics approaches for discovering biomarkers of drug-induced hepatoxicity and nephrotoxicity, Toxicol. Appl. Pharmacol., 2010, 243, 154–166 CrossRef CAS PubMed.
- A. P. Singh, A. Junemann, A. Muthuraman, A. S. Jaggi, N. Singh, K. Grover and R. Dhawan, Animal models of acute renal failure, Pharmacol. Rep., 2012, 64, 31–44 CrossRef CAS PubMed.
- T. Taguchi, A. Nazneen, M. R. Abid and M. S. Razzaque, Cisplatin-associated nephrotoxicity and pathological events, Contrib. Nephrol., 2005, 148, 107–121 CAS.
- D. Hoffmann, T. C. Fuchs, T. Henzler, K. A. Matheis, T. Herget, W. Dekant, P. Hewitt and A. Mally, Evaluation of a urinary kidney biomarker panel in rat models of acute and subchronic nephrotoxicity, Toxicology, 2010, 277, 49–58 CrossRef CAS PubMed.
- J. T. Santoso, J. A. Lucci 3rd, R. L. Coleman, I. Schafer and E. V. Hannigan, Saline, mannitol, and furosemide hydration in acute cisplatin nephrotoxicity: a randomized trial, Cancer Chemother. Pharmacol., 2003, 52, 13–18 CrossRef CAS PubMed.
- J. V. Bonventre, V. S. Vaidya, R. Schmouder, P. Feigan and F. Dieterle, Next-generation biomarkers for detecting kidney toxicity, Nat. Biotechnol., 2010, 28, 436–440 CrossRef CAS PubMed.
- C. G. Duarte and H. G. Preuss, Assessment of renal function-glomerular and tubular, Clin. Lab. Med., 1993, 13, 33–52 CAS.
- S. M. Hewitt, J. Dear and R. A. Star, Discovery of protein biomarkers for renal diseases, J. Am. Soc. Nephrol., 2004, 15, 1677–1689 CrossRef PubMed.
- J. W. Davis and J. A. Kramer, Genomic-based biomarkers of drug-induced nephrotoxicity, Expert Opin. Drug Metab. Toxicol., 2006, 2, 95–101 CrossRef CAS PubMed.
- R. Correa-Rotter, M. E. Ibarra-Rubio, G. Schwochau, C. Cruz, J. R. Silkensen, J. Pedraza-Chaverri, D. Chmielewski and M. E. Rosenberg, Induction of clusterin in tubules of nephrotic rats, J. Am. Soc. Nephrol., 1998, 9, 33–37 CAS.
- J. Mishra, K. Mori, Q. Ma, C. Kelly, J. Barasch and P. Devarajan, Neutrophil gelatinase-associated lipocalin a novel early urinary biomarker for cisplatin nephrotoxicity, Am. J. Nephrol., 2004, 24, 307–315 CrossRef CAS PubMed.
- W. C. Prozialeck, J. R. Edwards, P. C. Lamar, J. Liu, V. S. Vaidya and J. V. Bonventre, Expression of kidney injury molecule-1(Kim-1) in relation to necrosis and apoptosis during the early stages of Cd-induced proximal tubule injury, Toxicol. Appl. Pharmacol., 2009, 238, 306–314 CrossRef CAS PubMed.
- J. Mishra, C. Dent, R. Tarabishi, M. Mitsnefes, Q. Ma, C. Kelly, S. Ruff, K. Zahedi, M. Shao, J. Bean, K. Mori, J. Barasch and P. Devarajan, Neutrophil gelatinase-associated lipocalin (NGAL) as a biomarker for acute renal injury after cardiac surgery, Lancet, 2005, 365, 1231–1238 CrossRef CAS.
- O. Liangos, M. C. Periyanayagam, V. S. Vaidya, W. K. Han, R. Wald, H. Tighiouart, R. W. MacKinnon, L. Li, V. S. Balakrishnan, B. J. Pereira, J. V. Bonventre and B. L. Jaber, Urinary N-acetyl-beta-(D)-glucosaminidase activity and kidney injury molecule-1 level are associated with adverse outcomes in acute renal failure, J. Am. Soc. Nephrol., 2007, 18, 904–912 CrossRef CAS PubMed.
- W. K. Han, S. S. Waikar, A. Johnson, R. A. Betensky, C. L. Dent, P. Devarajan and J. V. Bonventre, Urinary biomarkers in the early diagnosis of acute kidney injury, Kidney Int., 2008, 73, 863–869 CrossRef CAS PubMed.
- T. Murata, H. Hibasami, S. Maekawa, T. Tagawa and K. Nakashima, Preferential binding of cisplatin to mitochondrial DNA and suppression of ATP generation in human malignant melanoma cells, Biochem. Int., 1990, 20, 949–955 CAS.
- M. Kruidering, B. Van de Water, E. de Heer, G. J. Mulder and J. F. Nagelkerke, Cisplatin-induced nephrotoxicity in porcine proximal tubular cells: mitochondrial dysfunction by inhibition of complexes I to IV of the respiratory chain, J. Pharmacol. Exp. Ther., 1997, 280, 638–649 CAS.
- J. Klawitter, J. Bendrick-Peart, B. Rudolph, V. Beckey, J. Klawitter, M. Haschke, C. Rivard, L. Chan, D. Leibfritz, U. Christians and V. Schmitz, Urine metabolites reflect time-dependent effects of cyclosporine and sirolimus on rat kidney function, Chem. Res. Toxicol., 2009, 22, 118–128 CrossRef CAS PubMed.
- J. C. Park, Y. S. Hong, Y. J. Kim, J. Y. Yang, E. Y. Kim, S. J. Kwack, D. H. Ryu, G. S. Hwang and B. M. Lee, A metabonomic study on the biochemical effects of doxorubicin in rats using 1H-NMR spectroscopy, J. Toxicol. Environ. Health, Part A, 2009, 72, 374–384 CrossRef CAS PubMed.
- M. Sieber, D. Hoffmann, M. Adler, V. S. Vaidya, M. Clement, J. V. Bonventre, N. Zidek, E. Rached, A. Amberg, J. J. Callanan, W. Dekant and A. Mally, Comparative analysis of novel noninvasive renal biomarkers and metabonomic changes in a rat model of gentamicin nephrotoxicity, Toxicol. Sci., 2009, 109, 336–349 CrossRef CAS PubMed.
- T. Niwa, N. Takeda, A. Tatematsu and K. Maeda, Accumulations of indoxyl sulfate, an inhibitor of drug-binding, in uremic serum as demonstrated by internal-surface reversed-phase liquid chromatography, Clin. Chem., 1998, 34, 2264–2267 Search PubMed.
- A. Enomoto, M. Takeda, A. Tojo, T. Sekine, S. H. Cha, S. Khamdang, F. Takayama, L. Aoyama, S. Nakamura, H. Endou and T. Niwa, Role of organic anion transporters in the tubular transport of indoxyl sulfate and the induction of its nephrotoxicity, J. Am. Soc. Nephrol., 2002, 13, 1711–1720 CrossRef CAS PubMed.
- T. Sakai, T. Maruyama, H. Imamura, H. Shimada and M. Otagiri, Mechanism of
stereoselective serum binding of ketoprofen after hemodialysis, J. Pharmacol. Exp. Ther., 1996, 278, 786–792 CAS.
Footnote |
† Electronic supplementary information (ESI) available. See DOI: 10.1039/c5mb00492f |
|
This journal is © The Royal Society of Chemistry 2016 |
Click here to see how this site uses Cookies. View our privacy policy here.