Understanding the hydrologic impacts of wastewater treatment plant discharge to shallow groundwater: before and after plant shutdown†
Received
24th May 2016
, Accepted 17th June 2016
First published on 30th June 2016
Abstract
Effluent-impacted surface water has the potential to transport not only water, but wastewater-derived contaminants to shallow groundwater systems. To better understand the effects of effluent discharge on in-stream and near-stream hydrologic conditions in wastewater-impacted systems, water-level changes were monitored in hyporheic-zone and shallow-groundwater piezometers in a reach of Fourmile Creek adjacent to and downstream of the Ankeny (Iowa, USA) wastewater treatment plant (WWTP). Water-level changes were monitored from approximately 1.5 months before to 0.5 months after WWTP closure. Diurnal patterns in WWTP discharge were closely mirrored in stream and shallow-groundwater levels immediately upstream and up to 3 km downstream of the outfall, indicating that such discharge was the primary control on water levels before shutdown. The hydrologic response to WWTP shutdown was immediately observed throughout the study reach, verifying the far-reaching hydraulic connectivity and associated contaminant transport risk. The movement of WWTP effluent into alluvial aquifers has implications for potential WWTP-derived contamination of shallow groundwater far removed from the WWTP outfall.
Water impact
WWTP cessation is a rare environmental event that tests the assumptions of the hydrologic changes after the effluent is removed from the system. Results show far ranging effects (>300 m into the bank and 3 km downstream). This movement of WWTP effluent into alluvial aquifers has implications for potential WWTP-derived contamination of shallow groundwater far removed from the WWTP outfall.
|
1 Introduction
Water reuse is essential to address water-supply limitations imposed by population growth, increased urbanization, climate change, and related water quality impairments. For a growing number of surface water systems, treated wastewater discharge is required to meet downstream ecological flows, aquifer recharge, and other water-supply requirements1 but such releases may directly impact downgradient aquatic ecosystem health2–9 as well as surface water and groundwater quality.10–15 During periods of low flow, wastewater treatment plant (WWTP) effluent can dominate streamflow, especially in urban areas,16–19 as well as the quality and quantity of local shallow groundwater recharge.20–22 For example, pharmaceutical contamination has been documented in shallow groundwater20 and in bank-filtered water-supply wells23 adjacent to effluent-impacted surface water systems. Likewise, the importance of ongoing wastewater discharge as a driver of downstream and adjacent shallow groundwater levels has been documented.20
In light of the global importance of recharge of subsurface freshwater supplies24–26 and the potential water-quality repercussions of hydrologic transport of wastewater-derived contaminants from effluent-impacted surface water to contiguous groundwater systems,12,14,15,27,28 improved understanding of the impacts of treated wastewater discharge on local hydrologic and biochemical conditions is critical. WWTP closures are rare wastewater remedial actions with the potential to provide unique insight into the impact of WWTP discharge on local hydrologic and biochemical conditions. The U.S. Geological Survey (USGS) conducted a combined pre/post-closure ecosystem assessment at a WWTP facility on Fourmile Creek in Ankeny, Iowa, USA that has been in existence since the 1970's. A two-month assessment conducted one year prior to WWTP closure indicated that effluent discharge was the primary driver of nearfield (80 m upstream to 30 m downstream of the outfall) instream and shallow groundwater levels.20 This paper examines water level changes within the stream, hyporheic zone, and adjacent shallow groundwater system before, during, and after WWTP closure recharge at distances up to 3 km downstream of the WWTP outfall in order to directly test that earlier conclusion20 and to further assess the importance of WWTP discharge as a driver of in-stream/near-stream hydrologic conditions and shallow-groundwater at distances farther removed from the WWTP outfall. In-stream and shallow groundwater biochemical responses to WWTP closure are assessed elsewhere.29 To the authors' knowledge this is the first documentation of local hydrologic conditions before and after a WWTP closure.
2 Methods
2.1 Site description
The study reach was located on Fourmile Creek in Ankeny, Iowa (Fig. 1). Ankeny is located in Polk County, approximately 16 kilometers north of Des Moines. In 2013, the population of Ankeny was an estimated 51
567 people.30 The contributing Fourmile Creek watershed was approximately 161.4 km2 and characterized by 75.3% cultivated crops, 20.0% developed (open space and low, medium, high intensity), and 4.7% other.31 This region has a temperate continental and humid climate. The mean annual temperature is 9.7 °C with a mean January temperature of −6.2 °C and a mean July temperature of 24.1 °C.32
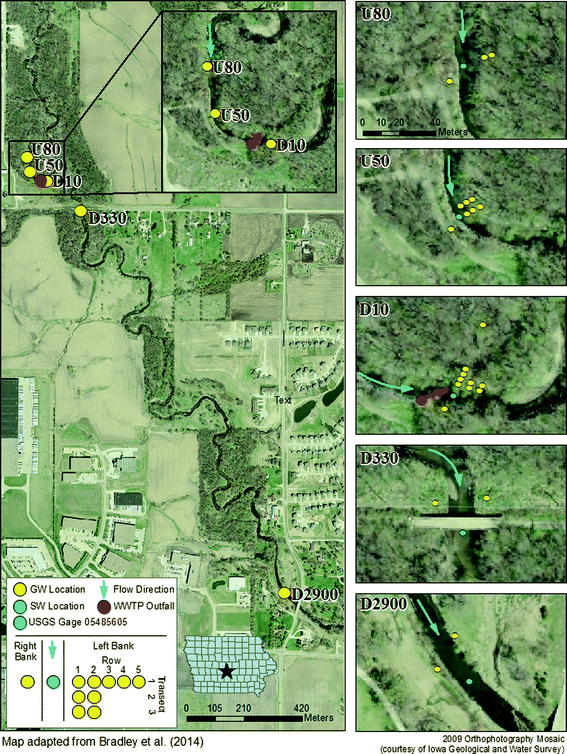 |
| Fig. 1 Map of Fourmile Creek study area in Ankeny, Iowa with groundwater (GW) and surface water (SW) sampling locations at 80 m upstream (U80), 50 m upstream (U50), 10 m downstream (D10), 330 m downstream (D330), and 2900 m downstream (D2900) from the wastewater treatment plant (WWTP) effluent discharge point. Adapted from Bradley et al. (2014). | |
The majority of the Fourmile Creek study watershed is composed of loam and clay loam with 0 to 6% slopes. The dominant soil series are the Canisteo clay loam (24.8%), Nicollet loam (22.6%), Clarion loam (19.8%), and Webster clay loam (16.2%), covering approximately 83.4% of the watershed.33 The Spillville-Coland complex is a relatively deep, occasionally-flooded, 0–2% slope loam/clay loam that characterizes both banks of Fourmile Creek and extends approximately 40–300 meters on either side of the channel within the study reach.
During operation, the Ankeny WWTP was a 46 million liter per day activated sludge treatment facility that continually discharged treated municipal effluent into Fourmile Creek.34 In November of 2013, the city of Ankeny began the process of shutting down the treatment plant and diverting its waste-stream to a Des Moines WWTP. WWTP discharge decreased incrementally over approximately 2 weeks, with the primary effluent discharge line disconnected on 11/08/2013 and remaining ancillary lines disconnected on 11/13/2013.35
The Fourmile Creek study reach extended from approximately 80 m upstream to approximately 2900 m downstream of the WWTP outfall (Fig. 1). Water levels were monitored in select bank piezometers, in-stream piezometers, and hyporheic zone piezometers. The study reach of Fourmile Creek had five sampling networks, with names (U80, U50, D10, D330, and D2900) indicating approximate distance in meters upstream (U) or downstream (D) of the WWTP effluent discharge point (Fig. 1). All sampling locations had mid-channel (in-stream/hyporheic) and out-of-channel (bank) piezometer groundwater locations, in addition to in-stream surface water locations. The pre/post closure assessment extended from October 2012 to October 2014. This paper assesses water level changes during the period of approximately 1.5 months pre- and 0.5 months post-closure (10/01/2013 to 11/30/2013).
2.2 Water level monitoring
Piezometers were installed both mid-channel (in-stream) and out-of-channel (bank) using a hand auger and back-filled with augured substrata. Bank piezometers were polyvinyl chloride (PVC) casing 5 cm in diameter and 3.4 m long with a 30 cm PVC screen (0.25 mm slot size)20 and installed immediately below the long-term water table, approximately 3 m below land surface. In-stream piezometers were 5 cm in diameter, 1.5 m long casing attached to a 30 cm screened interval (0.25 mm slot size).20
Bank piezometers were installed on both the right and left banks (LB) at U80, U50, D10, D330, and D2900. On the left bank at U80, U50, and D10, transects (T) and rows (R) of piezometers were installed (Fig. 1). Left bank D10-T1 piezometers were located approximately 1, 2, 3.5, 6 and 20 m from the stream bank (R1, R2, R3, R4, R5).20 At U80 (T1), U50 (T1, T2), and D10 (T2, T3), piezometers were located at 1 and 2 m, 1, 2, and 3 m, and 1 and 2 m from the stream bank, respectively (Fig. 1). At U80 and U50, one in-stream (IS) piezometer was installed at a nominal depth of 15–45 cm below the bed sediment/water interface (shallow). At D10, D330, and D2900, three in-stream nested piezometers were installed at nominal depths of (1) 15–45 cm below the bed sediment/water interface (shallow or “shall”), (2) 45–75 cm below the bed sediment/water interface (intermediate or “int”), and (3) 75–105 cm below the bed sediment/water interface (deep or “deep”).20
HOBO U20 submersible pressure transducers36 were installed within piezometer screened intervals at the following locations: U50-T2-R2, D10-IS-shall, D10-IS-int, D10-IS-deep, D10-T1-R1, D10-T1-R2, D10-T1-R3, D10-T1-R4, D10-T1-R5, D10-T2-R2, D10-T3-R2, D330-LB, and D2900-LB. A separate transducer was installed above the water table and just below land surface in the D10-RB piezometer to record local barometric pressure changes. Data were stored in 10 minute intervals and downloaded approximately every 3 months. HOBOware software was used to correct the water level data for atmospheric pressure. Before and after each download, a tape measurement was collected from the top of the PVC pipe to water level. Piezometer locations and top-of-casing elevations were surveyed periodically throughout the study using real-time kinematic (RTK) satellite information to monitor piezometer movement and correct water level to mean sea level datum (data available at http://dx.doi.org/10.5066/F7RF5S3P).
2.3 Field measurements
Stream discharge measurements were made by portable acoustic Doppler velocimetry37 according to USGS standard methods.38 Measurements were made upstream (U80) and downstream (D10) of the WWTP effluent discharge point approximately quarterly. Continuous stream stage was also collected approximately 330 m downstream of the WWTP effluent discharge at the USGS streamflow gaging station (05485605) at Fourmile Creek (http://dx.doi.org/10.5066/F7RF5S3P). Continuous streamflow data were computed using USGS standard techniques.39
3 Results
3.1 Stream discharge and precipitation
The Fourmile Creek basin experienced a severe drought during the period of the study (10/01/2013–12/05/2013),40 with a precipitation departure from normal of −19.7 cm in July–August following a +18.7 cm departure from normal in March–April. Stream discharge at Fourmile Creek (05485605) during the period before and just after shutdown was below the median daily mean flow (12 years) with the exception of three events, on October 4, October 15, and October 30, 2013 (Fig. 2http://dx.doi.org/10.5066/F7RF5S3P). Two cessation events had the greatest impact on the WWTP effluent shutdown, a disconnection of a 91 cm line on 11/08/2013 and disconnection of all remaining WWTP flow to the plant on 11/13/2013. A transition period occurred for approximately 7 days after the disconnection on 11/13/2013 whereby flushing out of the infrastructure created differentials in the streamflow and water level data (Fig. 2). After all the flow to the plant was disconnected (11/13/2013), there was a noticeable increase in the difference from the median daily mean flow to continuous streamflow as the previously constant contribution of WWTP effluent ceased (Fig. 2).
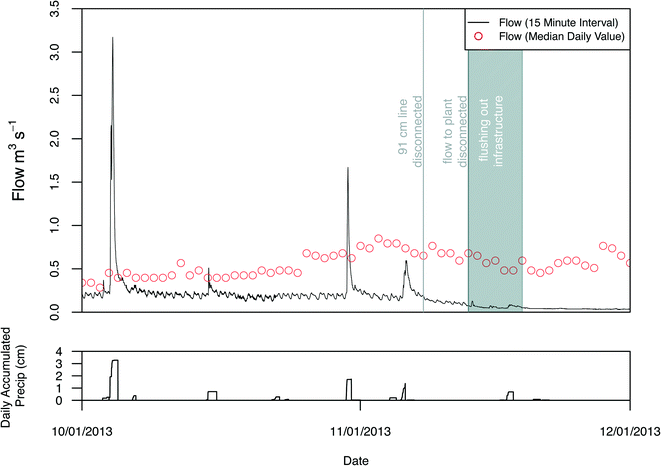 |
| Fig. 2 Continuous flow (15 minute interval; m3 s−1), median daily flow (12 years, m3 s−1), and daily accumulated precipitation (cm) at USGS Fourmile Creek near Ankeny, IA DS1 during the period October 1 to December 1, 2013. Vertical lines denote when the 91 cm line was disconnected (November 8, 2013) and when all flow to the Ankeny wastewater treatment facility was disconnected (November 13, 2013). Gray highlighted area denote the 7 day transitional period that included flushing out the infrastructure. | |
Under low-flow conditions, streamflow was dominated by WWTP effluent prior to shutdown. Immediately before the onset of shutdown on 10/22/2013, discharge was measured at U80 (0.0098 m3 s−1) and D10 (0.18 m3 s−1), with wastewater effluent accounting for 95% of streamflow. After shutdown (11/20/2013), the difference in discharge measured between U80 (0.038 m3 s−1) and D10 (0.034 m3 s−1) was within the 5% measurement error.39
3.2 Effluent influence
In addition to providing nearly all of the flow to Fourmile Creek below the WWTP outfall during the pre-closure study period, the WWTP discharge created a clear diurnal pattern (Fig. 3) in Fourmile Creek (05485605), with the largest flow addition to the creek occurring midmorning to midday (between 9:15 and 14:00, the latter time occurred on weekend days) and the smallest addition occurring in early morning (between 4:15 and 6:30).20,41 Two 7 day periods are included in Fig. 3 to demonstrate the temporal continuity of this diurnal pattern derived from the WWTP discharge. The variation in daily flow typically ranged from 0.062 to 0.082 m3 s−1. Two peaks were observed most days (midday peak and evening peak), indicating two common periods of increased waste loading to the WWTP.
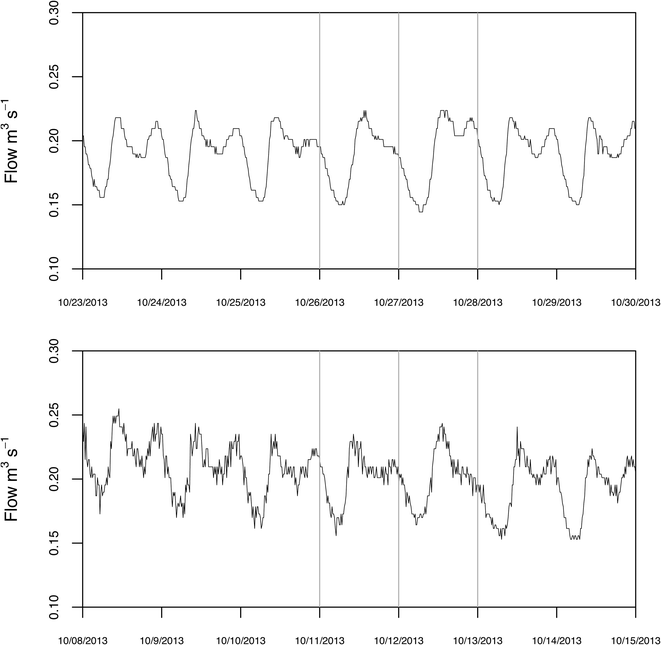 |
| Fig. 3 Continuous flow (15 minute interval; m3 s−1) during two 7 day periods. Vertical lines denote Saturday and Sunday of each week. | |
The same WWTP-derived diurnal pattern documented in streamflow, was also observed in the bank piezometers.20Fig. 4 shows the T1 transect along the left bank at D10, located 10 m downstream of the WWTP effluent discharge location. D10-IS-shall was located in-stream (hyporheic shallow) and was used in place of the D10-IS-SW as the water level data were nearly identical.20 The water table elevation in the bank piezometers closely mirrored the shallow in-stream (WWTP effluent) signal (Fig. 4). During rain events, the diurnal WWTP signal was absent on the rising limb and peak, reappearing late on the falling limb of the hydrograph (Fig. 4). While the signal was slightly muted compared to D10-T1-R1 to D10-T1-R4, the pattern was still discernable at D10-T1-R5 (Fig. 4 and 5). For example, during the rain event on 11/6/2013, the amplitude of the peak was reduced by approximately 41% at D10-T1-R5 compared to D-10-T1-R1.
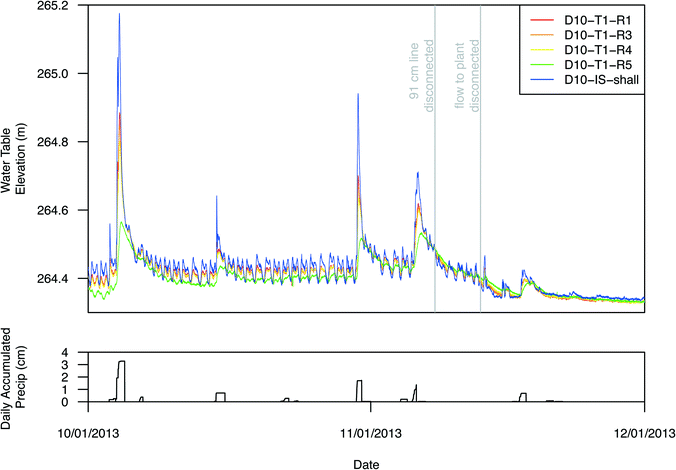 |
| Fig. 4 Continuous water level profiles (NAVD88; 10 minute interval; m) data for in-stream and left bank, transect 1 piezometers at D10 (D10-T1-R1, D10-T1-R2, D10-T1-R3, D10-T1-R4, D10-T1-R5, D10-IS-shall) and daily accumulated precipitation (cm) data for the period October 1 to December 1, 2013. Vertical lines denote date that the 91 cm line was disconnected and date when all flow to the Ankeny wastewater treatment facility was disconnected. | |
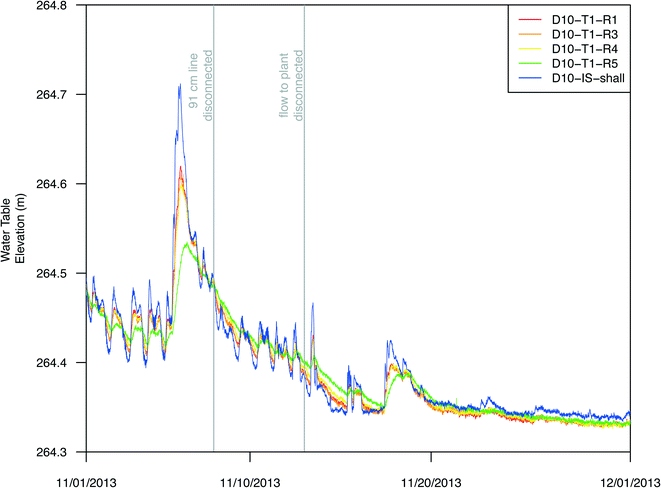 |
| Fig. 5 Continuous water level profiles (NAVD88; 10 minute interval; m) data for in-stream and left bank, transect 1 piezometers at D10 (D10-T1-R1, D10-T1-R2, D10-T1-R3, D10-T1-R4, D10-T1-R5, D10-IS-shall) for the period November 1 to December 1, 2013. Vertical lines denote date that the 91 cm line was disconnected and date when all flow to the Ankeny wastewater treatment facility was disconnected. | |
A noticeable shift in the water levels occurred after disconnection of the primary effluent line. The water levels before the precipitation event on 11/7/2014 indicate a flow from D10-T1-R1 to D10-T1-R5, whereas the water levels after the disconnection on 11/13/2013 indicate a flow from D10-T1-R5 to D10-T1-R1, with the exception of the flushing activities and precipitation event between 11/13/2013 and 11/17/2013 (Fig. 5). Before the disconnection, the hydrologic gradient was from the stream toward the groundwater system (Fig. 5). After the disconnection, the stream and bank water levels decreased and the hydrologic gradient reversed from groundwater toward the stream except during high-flow events (Fig. 5).
Left bank piezometer water level data from U50 to D2900 are shown in Fig. 6. The pre shutdown data (10/01/2013–11/13/2013) show a clear WWTP signal from D10 downstream to D2900 (almost 3 km downstream of the WWTP effluent outfall). U50, although upstream of the effluent discharge point, also has a WWTP signal due to recirculation of effluent upstream of the outfall that occurs during normal to low stream-flow conditions.20 This upstream recirculation was verified by a surface water dye-tracer test conducted in October of 2011 (ESI† Fig. S1). Subsequently, an additional piezometer network was established 80 m (U80) farther upstream of the outfall (data not shown).
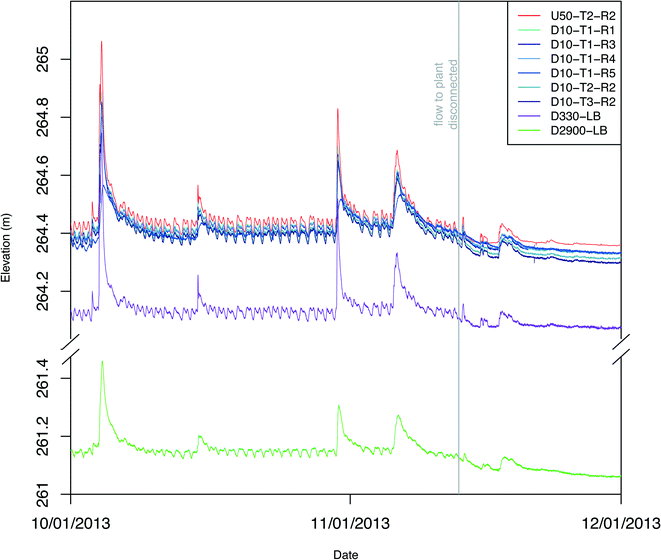 |
| Fig. 6 Continuous water level profiles (NAVD88; 10 minute interval; m) data in left bank piezometers at U50, D10, D330, and D2900 for the period October 1 to December 1, 2013. | |
Fig. 7 shows detailed water level data for bank and instream piezometers at D10. Before disconnection, all the D10 bank piezometers (transects T1, T2, and T3) and D10-IS-shall and D-10-IS-int water levels mirrored the in-stream effluent diurnal signal. D10-IS-deep also responded to the WWTP effluent signal, but the signal was substantially attenuated (Fig. 7). In addition, during higher flow events, D10-IS-shall, D10-IS-int, and D10-IS-deep piezometers were fully submerged by stream water. After the 91 cm line was disconnected, water levels at all D10 locations rapidly declined (Fig. 6 and 7). After disconnection, D10-IS-shall and D10-IS-int water levels were comparable, with D10-IS-deep water level exhibiting a similar but muted signal (Fig. 7). For instance, during the rain event on 11/17/2013, the peak amplitude at D10-IS-deep was 70% less than D10-IS-shall.
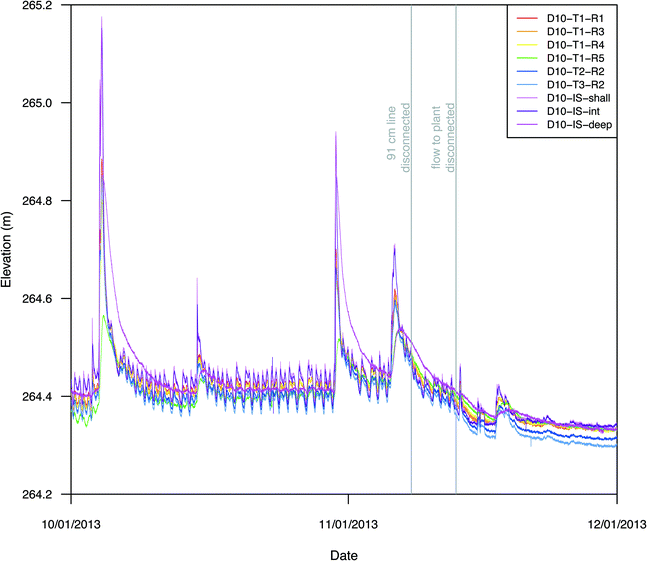 |
| Fig. 7 Continuous water table elevation (10 minute interval; m) data in left bank piezometers at U50 and D10 for the period October 1 to December 1, 2013. | |
4 Discussion
The tight correspondence between stream and bank piezometer water levels indicated strong connectivity between the stream and the shallow groundwater system in this study reach of Fourmile Creek. Similarly, Bradley et al.20 concluded that WWTP effluent discharge was a primary driver of hydrologic conditions in the shallow groundwater system during the period October to December 2012 (pre-closure). Moreover, the mirroring of bank-piezometer and in-stream water levels indicated minimal flow restriction into the left bank (Fig. 4). The WWTP effluent impacts extend greater than 20 m into the bank to D10-T1-R5 (Fig. 4). Signal attenuation from D10-T1-R1 to D10-T1-R5 was approximately 0.03 m over the 20 m horizontal distance. Using values of hydrologic conductivity for coarse sand sediments42,43 characteristic of the screened intervals of the D10-T1 piezometers, Darcy velocities were calculated and used to estimate the lateral extent of the effluent influence in the shallow groundwater system. Assuming a comparable geologic structure, an attenuation of less than 0.5 m was estimated at a distance of 300 m from the stream bank (the approximate width of the Spillville-Coland complex on the left bank at D10), indicating that the hydrologic impact of WWTP effluent extended well beyond the 20 m distance monitored in this study. Good hydraulic connectivity and a substantial, effluent-driven hydraulic gradient between the stream and the bank favored transport of WWTP-associated water soluble contaminants and efficient exchange with the shallow groundwater system under pre-closure conditions. Importantly, however, the limited to negligible hydraulic gradient between shallow groundwater and the stream under post-closure conditions indicates inefficient hydrologic transport, suggesting that long-term persistence (on the scale of years) of residual groundwater contamination may be substantial even for relatively water-soluble contaminants like pharmaceuticals.29
The connectivity between the stream and aquifer were also characterized by estimating hydraulic diffusivity at the D10 study location using the stage-ratio method described by Ferris44 that correlates observed well response to river-stage variation. Assumptions for this method are 1) the river fluctuation is an approximate sinusoidal pattern, 2) the aquifer is homogenous, 3) the river penetrates the aquifer, and 4) no significant vertical ground-water flow components are present. Hydraulic results for D10-T1-R1 and D10-T1-R5 are 12.7 and 12 m2 day−1, respectively. These numbers suggest a large storage capacity of the unconfined alluvial aquifer.
Likewise, the connectivity between the stream and the shallow groundwater system was apparent 2900 m downstream of the outfall (Fig. 6), indicating that WWTP effluent discharge can have a profound effect on the hydrology of the stream and adjacent groundwater system, at least several km downstream of the WWTP outfall. The effluent discharge signal was also observed several meters upstream at U50 (Fig. 6). Fig. 8 shows two separate 7 day periods, one pre-closure and one post-closure. During the pre-closure 7 day period, the daily WWTP discharge signal was clear in the left bank at D2900, although the amplitude was diminished by approximately 40% compared to the D10 network. The wavelength of the WWTP inputs was the same at D10 and D2900, with a 3.5 h delay at D2900. The 3.5 hour delay was visible in the post-closure 7 day period during the rain event on 11/17/2013–11/18/2013. The mirroring of groundwater fluctuations at both D10 and D2900, nearly 3 km from the WWTP effluent source, indicate a strong, spatially extensive connectivity between the stream and the shallow groundwater system. Furthermore, these results demonstrate the transmissivity and hydraulic conductivity of the Spillville-Coland complex and has implications for many alluvial aquifers with similar geologic and hydraulic properties.
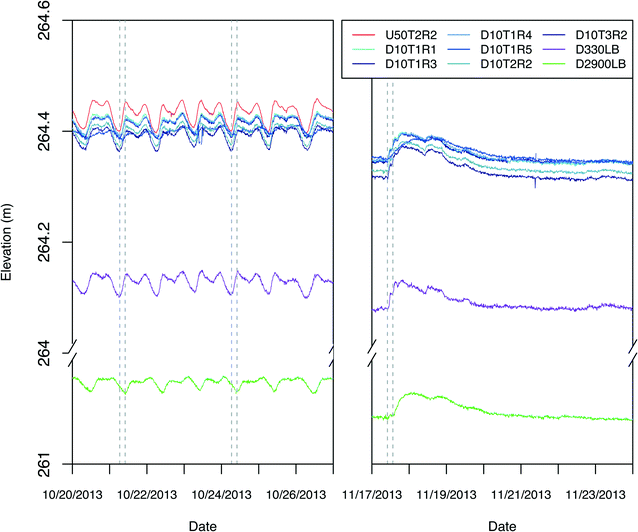 |
| Fig. 8 Continuous water level profiles (NAVD88; 10 minute interval; m) data in left bank piezometers at U50 and D10 for two 7 day periods, one pre-closure (October 20–October 27, 2013) and one post-closure (November 13 to November 20, 2013). Vertical dashed lines denote left bank water level change delay from D10 to D2900. | |
The hydraulic pumping of WWTP effluent into the stream bank in Fourmile Creek directly affected water levels in the groundwater piezometers, with groundwater levels closely mirroring that in the adjacent stream channel (Fig. 4). When this hydraulic pumping was eliminated, the effect was reflected within hours in the bank piezometers, comparable to the response time demonstrated by the WWTP diurnal discharge fluctuations (approximately 3.5 hours). After the stream was no longer receiving WWTP effluent, the stream reacted quickly to precipitation events and the left bank piezometers exhibited an associated water-level response (Fig. 4). In addition, the water level data demonstrated a fundamental shift from a losing stream reach before disconnection into a gaining reach beginning immediately after WWTP closure (Fig. 5). The rapid loss of the WWTP signal in the piezometers after closure is further evidence of the hydrologic connectivity between the stream and shallow groundwater system.
These results have implications for the potential corresponding transport of a variety of organic compounds, including pharmaceuticals and hormones, into the shallow groundwater system km downstream from the discharge point and also several hundred meters into the stream bank. Alluvial aquifer systems are important sources of drinking water in Iowa and throughout the U.S.45 Thus, the current results illustrate the potential risk of hydrologic transport of dissolved wastewater contaminants from surface water to contiguous alluvial aquifers.
5 Conclusions
The shutdown of the Ankeny WWTP provided a unique opportunity to monitor and observe the hydrologic conditions before and after WWTP shutdown. By monitoring the stream, hyporheic zone, and bank, the WWTP effluent's hydrologic effects were examined and characterized. Before shutdown, the WWTP effluent had a strong diurnal pattern that was not only closely mirrored in the opposite bank proximal to the WWTP outfall, but was also observed nearly 3 km downstream. This diurnal, pumping effect quickly ceased when the WWTP went offline. The results illustrate the potential for WWTP effluent to effect in-stream and near-stream hydrology upstream and also far removed (e.g. kilometers) downstream of the outfall. This movement of WWTP effluent into alluvial aquifers has implications for potential WWTP-derived contamination of shallow groundwater supplies far from the WWTP outfall.
Supplementary data available
Supplementary data to this article can be found online at Environ. Sci.: Water Res. Technol. (http://dx.doi.org/10.1039/c6ew00128a) and in machine-readable format at USGS ScienceBase (http://dx.doi.org/10.5066/F7RF5S3P).
Acknowledgements
The research was supported by U.S. Geological Survey Toxic Substances Contaminant Hydrology Program. Any use of trade, firm, or product names is for descriptive purposes only and does not imply endorsement by the U.S. Government. The authors thank Ken Plager and the staff of the Ankeny WWTP for logistical support and access to the Fourmile Creek study area.
References
-
National Research Council, Water Reuse: Potential for Expanding the Nation's Water Supply through Reuse of Municipal Wastewater, Academy Press, Washington, DC, 2012 Search PubMed.
- S. Jobling, M. Nolan, C. Tyler, G. Brighty and J. Sumpter, Widespread sexual disruption in wild fish, Environ. Sci. Technol., 2008, 32, 2498–2506 CrossRef.
- K. A. Kidd, P. J. Blanchfield, K. H. Mills, V. P. Palance, R. E. Evans, J. M. Lazorchak and R. W. Flick, Collapse of a fish population after exposure to a synthetic estrogen, Proc. Natl. Acad. Sci. U. S. A., 2007, 104, 8897–8901 CrossRef CAS PubMed.
- M. R. McGee, M. L. Julius, A. M. Vajda, D. O. Norris, L. B. Barber and H. L. Schoenfuss, Predator avoidance performance of larval fathead minnows (Pimephales promelas) following short-term exposure to estrogen mixtures, Aquat. Toxicol., 2009, 91, 355–361 CrossRef CAS PubMed.
- J. P. Nash, D. E. Kime, L. T. Van der Ven, P. W. Wester, F. Brion, G. Maack, P. Stahlschmidt-Allner and C. R. Tyler, Long-term exposure to environmental concentrations of the pharmaceutical ethynylestradiol causes reproductive failure in fish, Environ. Health Perspect., 2004, 112, 1725–1733 CrossRef CAS PubMed.
- N. J. Neimuth, R. Jordan, J. Crago, C. Blanksma, R. Johnson and R. D. Klaper, Metformin exposure at environmentally relevant concentrations causes potential endocrine disruption in adult male fish, Environ. Toxicol. Chem., 2015, 34, 291–296 CrossRef PubMed.
- M. M. Painter, M. A. Buerkley, M. L. Julius, A. M. Vajda, D. O. Norris, L. B. Barber, E. T. Furlong, M. M. Schultz and H. L. Schoenfuss, Antidepressants at environmentally relevant concentrations affect predator avoidance behavior of larval fathead minnows (Pimephales promelas), Environ. Toxicol. Chem., 2009, 28, 2677–2684 CrossRef CAS PubMed.
- E. J. Rosi-Marshall, D. W. Kincaid, H. A. Bechtold, T. V. Royer, M. Rojas and J. J. Kelly, Pharmaceuticals suppress algal growth and microbial respiration and alter bacterial communities in stream biofilms, Ecol. Appl., 2013, 23, 583–593 CrossRef PubMed.
- A. M. Vajda, L. B. Barber, J. L. Gray, E. M. Lopez, J. D. Woodling and D. O. Norris, Reproductive disruption in fish downstream from and estrogenic wastewater effluent, Environ. Sci. Technol., 2008, 42, 3407–3414 CrossRef CAS PubMed.
- V. Acuna, D. von Schiller, M. J. Garcia-Galan, S. Rodriguez-Mozaz, L. Corominas, M. Petrovic, M. Poch, D. Barcelo and S. Sabater, Occurrence and in-stream attenuation of wastewater-derived pharmaceuticals in Iberian rivers, Sci. Total Environ., 2015, 503–504, 133–141 CrossRef CAS PubMed.
- M. Diaz-Cruz and D. Barcelo, Trace organic chemicals contamination in ground water recharge, Chemosphere, 2008, 72, 333–342 CrossRef CAS PubMed.
- S. Foster and P. J. Chilton, Downstream of downtown: Urban wastewater as groundwater recharge, Hydrogeol. J., 2004, 12, 115–120 CrossRef.
- E. Hamann, P. J. Stuyfzand, J. Greskowiak, H. Timmer and G. Massmann, The fate of organic micropollutants during long-term/long-distance river bank filtration, Sci. Total Environ., 2016, 545–546, 629–640 CrossRef CAS PubMed.
- D. J. Lapworth, N. Baran, M. E. Stuart and R. S. Ward, Emerging organic contaminants in groundwater: A review of sources, fate and occurrence, Environ. Pollut., 2012, 163, 287–303 CrossRef CAS PubMed.
- J. Lewandowski, A. Putschew, D. Schwesig, C. Neumann and M. Radke, Fate of organic micropollutants in the hyporheic zone of a eutrophic lowland stream: Results of a preliminary field study, Sci. Total Environ., 2011, 409, 1824–1835 CrossRef CAS PubMed.
- B. W. Brooks, T. M. Riley and R. D. Taylor, Water quality of effluent-dominated ecosystems: ecotoxicological, hydrological,
and management considerations, Hydrobiologia, 2006, 556, 365–379 CrossRef CAS.
- B. Dong, A. Kahl, L. Cheng, H. Vo, S. Ruehl, T. Zhang, S. Snyder, A. E. Saez, D. Quanrud and R. G. Arnold, Fate of trace organics in a wastewater effluent dependent stream, Sci. Total Environ., 2015, 518–519, 479–490 CrossRef CAS PubMed.
- G. E. Groschen, T. L. Arnold, M. A. Harris, D. H. Dupre, F. A. Fitzpatrick, B. C. Scudder, W. S. Morrow, P. J. Terrio, K. L. Warner and E. A. Murphy, Water quality in the Upper Illinois River Basin Illinois Indiana and Wisconsin 1999-2001, Geol. Surv. Circ., 2004, 1230, 42 Search PubMed.
- K. W. Robinson, S. M. Flanagan, J. D. Ayotte, K. W. Campo, A. Chalmers, J. F. Coles and T. F. Cuffney, Water Quality in the New England Coastal Basins, Maine, New Hampshire, Massachusetts, and Rhode Island 1999-2001, Geol. Surv. Circ., 2004, 1226, 38 Search PubMed.
- P. M. Bradley, L. B. Barber, J. Duris, W. T. Foreman, E. T. Furlong, L. E. Hubbard, K. J. Hutchinson, S. H. Keefe and D. W. Kolpin, Riverbank filtration potential of pharmaceuticals in a wastewater-impacted stream, Environ. Pollut., 2014, 193, 173–180 CrossRef CAS PubMed.
- K. Osenbruck, H. R. Gläser, K. Knoller, S. M. Weise, M. Moder, R. Wennrich, M. Schirmer, F. Reinstorf, W. Busch and G. Strauch, Sources and transport of selected organic micropollutants in urban groundwater underlying the city of Halle (Saale), Germany, Water Res., 2007, 41, 3259–3270 CrossRef PubMed.
- M. Rabiet, A. Togola, F. Brissaud, J. L. Seidel, H. Budzinski and F. Elbaz-Poulichet, Consequences of treated water recycling as regards pharmaceuticals and drugs in surface and ground waters of a medium-sized Mediterranean catchment, Environ. Sci. Technol., 2006, 40, 5282–5288 CrossRef CAS PubMed.
- T. Herberer, I. M. Verstraeten, M. Meyer, A. Mechlinski and K. Reddersen, Occurrence and Fate of Pharmaceuticals During Bank Filtration - Preliminary Results From Investigations in Germany and the United States, J. Contemp. Water Res. Educ., 2001, 120(1), 4–17 Search PubMed.
- E. H. Oelkers, J. G. Hering and C. Zhu, Water: Is There a Global Crisis?, Elements, 2011, 7(3), 157–162 CrossRef.
- F. W. Schwartz and M. Ibaraki, Groundwater: A Resource in Decline, Elements, 2011, 7(3), 175–179 CrossRef.
- J. F. Kenny, N. L. Barber, S. S. Hutson, K. S. Linsey, J. K. Lovelace and M. A. Maupin, Estimated use of water in the United States in 2005, Geol. Surv. Circ., 2009, 1344, 52 Search PubMed.
- S. R. Hughes, P. Kay and L. E. Brown, Global synthesis and critical evaluation of pharmaceutical data sets collected from river systems, Environ. Sci. Technol., 2013, 47, 661–677 CrossRef CAS PubMed.
- I. Michael, L. Rizzo, C. S. McArdell, C. M. Manaia, C. Merlin, T. Schwartz, C. Dagot and D. Fatta-Kassinos, Urban wastewater treatment plants as hotspots for the release of antibiotics in the environment: A review, Water Res., 2013, 47, 957–995 CrossRef CAS PubMed.
- P. M. Bradley, L. B. Barber, J. Clark, J. W. Duris, W. T. Foreman, E. T. Furlong, C. Givens, L. E. Hubbard, K. J. Hutchinson, C. Journey, S. H. Keefe and D. W. Kolpin, Pre/post-closure assessment of groundwater pharmaceutical fate in a wastewater facility-impacted stream reach, Sci. Total Environ., 2016 DOI:10.1016/j.scitotenv.2016.06.104.
-
U. S. Census Bureau, State & County QuickFacts, 2013, http://quickfacts.census.gov/qfd/states/19/1902305.html, (accessed September 2015) Search PubMed.
- C. G. Homer, J. A. Dewitz, L. Yang, S. Jin, P. Danielson, G. Xian, J. Coulston, N. D. Herold, J. D. Wickham and K. Megown, Completion of the 2011 National Land Cover Database for the conterminous United States-Representing a decade of land cover change information, Photogramm. Eng. Remote Sens., 2015, 81(5), 345–354 Search PubMed.
-
National Oceanic and Atmospheric Administration National Climatic Data Center, Climate Data Online Search, http://www.ncdc.noaa.gov/cdo-web/search, (accessed May 2015) Search PubMed.
-
Soil Survey Staff, Natural Resources Conservation Service, United States Department of Agriculture, Web Soil Survey, http://websoilsurvey.nrcs.usda.gov/, (accessed May 2015) Search PubMed.
- L. B. Barber, S. H. Keefe, G. K. Brown, E. T. Furlong, J. L. Gray, D. W. Kolpin, M. T. Meyer, M. W. Sandstrom and S. D. Zaugg, Persistence and potential effects of complex organic contaminant mixtures in wastewater-impacted streams, Environ. Sci. Technol., 2013, 47, 2177–2188 CrossRef CAS PubMed.
-
K. Plager, personal communication.
-
Onset HOBO Data Loggers, HOBO U20 Water Level Data Logger-u20-001-01, http://www.onsetcomp.com/products/data-loggers/u20-series-water-level-data-loggers, (accessed May 2015) Search PubMed.
-
SonTek, FlowTracker Handheld-ADV, http://www.sontek.com/flowtracker.php, (accessed May 2015) Search PubMed.
-
T. J. Buchanan and W. P. Somers, Discharge measurements at gaging stations, U.S. Geological Survey Techniques of Water-Resources Investigations, 1969, vol. 3, p. 65 Search PubMed.
- S. E. Rantz, Measurement and computation of streamflow. Volume 1: Measurement of stage and discharge; Volume 2: Computation of discharge, U.S. Geol. Surv. Water-Supply Pap., 1982, 2175, 1–631 Search PubMed.
-
U.S. Drought Monitor, U.S. Drought Monitor Map Archive, http://droughtmonitor.unl.edu/MapsAndData/MapArchive.aspx, (accessed June 2015) Search PubMed.
-
U.S. Geological Survey, National Water Information System: USGS Water Data for the Nation, http://waterdata.usgs.gov/nwis, (accessed June 2015) Search PubMed.
-
P. A. Domenico and F. W. Schwartz, Physical and Chemical Hydrogeology, New York, NY, John Wiley & Sons, Inc, 1990 Search PubMed.
-
U. S. Department of Agriculture Natural Resources Conservation Service, Saturated Hydraulic Conductivity in Relation to Soil Texture, http://www.nrcs.usda.gov/wps/portal/nrcs/detail/soils/survey/office/ssr10/tr/?cid=nrcs144p2_074846, (accessed October 2015) Search PubMed.
-
J. G. Ferris, Cyclic fluctuations of water level as a basis for determining aquifer transmissibility, U.S. Geological Survey Unnumbered Series, 1952, Note 1, p. 16, DOI:10.3133/70133368.
-
E. M. Sadorf and S. M. Linhart, Ground-water quality in alluvial aquifers in the eastern Iowa basins, Iowa and Minnesota, U.S. Geological Survey Water-Resources Investigations Report, 2000, vol. 00-4106 Search PubMed.
Footnotes |
† Electronic supplementary information (ESI) available. See DOI: 10.1039/c6ew00128a |
‡ Present address: Environmental Consulting & Technology, Inc., 332 S Linn St # 22, Iowa City, IA 52240, USA. |
|
This journal is © The Royal Society of Chemistry 2016 |
Click here to see how this site uses Cookies. View our privacy policy here.