Point-of-use water filters can effectively remove disinfection by-products and toxicity from chlorinated and chloraminated tap water†
Received
9th March 2016
, Accepted 30th June 2016
First published on 1st July 2016
Abstract
Epidemiological risk estimates point toward potential health risks posed by disinfection by-products (DBPs) in chlorinated drinking water. Point-of-use filters can effectively remove regulated DBPs from tap water but the removal of unknown DBPs and toxicity has not yet been assessed. We evaluated 11 tap water filters for their efficacy to abate fluoride, bacteria, and adsorbable organic halogens (AOX) as the sum parameter of known and unknown halogenated DBPs. Biological effects were quantified in water samples enriched with solid phase extraction by use of the Microtox assay for bacterial cytotoxicity, the AREc32 assay for oxidative stress response, and the umuC assay for genotoxicity. Six out of 11 filters effectively removed chlorinated and brominated organic halogens by >60%. Reverse osmosis and one activated carbon based gravity filter were most effective (>94% AOX removal). Four out of five non-membrane pressure filters were less effective for AOX abatement than four out of five activated carbon based gravity filters. Renewal of the filter cartridges significantly improved AOX removal efficacies. Fluoride was removed by >83% only by reverse osmosis and two filters specifically designed for fluoride removal by use of activated alumina. Bacterial counts increased after filtration with most filters, indicating biofilm growth on the filter matrix. Cartridge renewal decreased bacterial counts. Seven out of 11 filters reduced cytotoxicity, oxidative stress response, and genotoxicity by >60%. Activated carbon based tap water filters could provide an important short-term public health benefit through removal of halogenated DBPs, but regular filter cartridge exchange is critical to maintain a good filter efficacy.
Water impact
The life-long consumption of chlorinated drinking water may pose a health risk due to the toxic properties of some disinfection by-products. Point-of-use water filters can effectively remove disinfection by-products as evidenced by abatement of halogenated organic compounds as well as reduction of biological activity of the water constituents determined with bioassays for cytotoxicity, oxidative stress response, and genotoxicity.
|
1. Introduction
Implementation of drinking water disinfection and filtration effectively reduced pathogen-related waterborne diseases, such as typhoid fever and cholera, and therefore can be regarded as one of the most important public health advances of the last century.1–3 However, the discovery of disinfection by-products (DBPs)4—formed by the reaction between chemical disinfectants and natural organic matter (NOM) as well as inorganic precursors (e.g., bromide)—raised concerns due to their suspected adverse health effects.2,5–8 Therefore, application of chlorinous disinfectants is a balancing act between disinfection and the formation of DBPs.8 Epidemiological studies suggested an increased risk of bladder cancer after life-long ingestion of chlorinated drinking water.9–12 Findings of other diseases being associated with DBP exposure were less consistent.11 Based on epidemiological studies, the US-EPA calculated that 2–17% of the bladder cancer cases could be avoided if the exposure to DBPs was absent.13 It has to be emphasized, however, that the causation of urinary bladder cancer by DBP exposure has not been proven but remains a “working hypothesis”.11 The proof of causality between exposure to complex mixtures and adverse health effects is very challenging.14
More than 40 years of DBP research has led to an improved understanding of drinking water treatment processes as well as improved drinking water quality.9 Nevertheless, compounds that could explain the epidemiological outcomes have not been found.9 Given that >50% of the total organic halogens are unknown,15 unknown DBPs could be responsible for increased cancer risks. Unknown DBPs occur—most likely—at low concentrations and thus individual DBPs would need to have an extreme potency if they are to close the gap between toxic effects of known DBPs and potential adverse health outcomes caused by DBPs. Thus, it is highly likely that effects are triggered by additive mixture effects of unknown and known DBPs with similar mechanisms of action.
Point-of-use (PoU) filtration devices may reduce potential risks posed by DBP exposure. PoU filters should be considered as an end-of-pipe solution for distribution systems with chlorinous disinfectants. Preferably, in optimized drinking water systems, the use of chlorine can be minimized or avoided.3
It is estimated that in China (Beijing, Guangzhou, and Shanghai) and in the US around 11–30% of the population use household water purifiers16 and in Korea 90% of the population avoids to drink water directly from the tap.17 Even simple PoU filters, such as pitcher filters or other low-cost activated carbon filters, effectively reduced concentrations of trihalomethanes (THMs) or haloacetic acids (HAAs)16,18,19 as well as MX (3-chloro-4-(dichloromethyl)-5-hydroxy-5H-furan-2-one).20
In this study we assessed the DBP removal efficiency of 11 different PoU tap water filters by analyzing the total organic halogen (AOX) concentration as a sum parameter for halogenated DBPs. We further assessed the toxicity removal by analyzing the cytotoxicity, oxidative stress response induction, and genotoxicity of tap waters before and after filtration. Fluoride was quantified as its removal could be a desired or non-desired side effect of PoU water filtration. Finally, we quantified the bacterial counts to test if water filters reduce or increase the number of bacteria in tap water. These results could aid DBP exposure studies and epidemiological studies, in particular when bioanalytical results are included in investigations assessing exposure and health consequences of DBPs as recommended by Villanueva et al.14
2. Material & methods
2.1. Materials
Organic solvents (methanol, MeOH; methyl tert-butyl ether, MTBE) as well as concentrated sulfuric acid were purchased at a purity of ≥99.9% from Sigma Aldrich (Australia). Ultrapure water was prepared with a Milli-Q water purifier (Merck-Millipore, USA) from reverse osmosis treated tap water.
2.2. Water filter and sampling
We tested 11 different PoU tap water filters (Table S1†) located within the greater Brisbane area as well as Coffs Harbour (Australia) for their capacity to remove AOX and fluoride and ten of them for their performance to reduce biological effects. All drinking water treatment plants in the investigated areas use chlorination or chloramination for disinfection of reservoir water after coagulation and clarification/sand filtration. The distribution systems are connected with all treatment plants in the investigated areas and thus the tap waters could be a mixture originating from different drinking water treatment plants with differing disinfection methods. The AOX composition of the water samples is stated in Table S2.† We assessed one reverse osmosis filter, five non-membrane pressure filters, which are directly attached to a tap, and five gravity filters. Most filters tested in this study were designed for removal of chlorine, chloramine, microorganisms, and some for the additional removal of organic contaminants (e.g., reverse osmosis or filter with activated carbon).
To assess the filter efficiency, tap water was sampled before and after filtration headspace-free in amber glass bottles with PTFE-lined screw caps. Prior to sampling we let the tap water run >3 minutes and sampling was performed causing minimized turbulence (sampling with low water flow and the bottle held at an angle) to reduce the loss of volatile DBPs. The samples were extracted and analyzed on the same day. For each filter, water was sampled two to ten times at different time points. All filters were tested within the recommended service intervals and are described in more detail in Table S1† including the filtered water volume before sampling and the recommended lifetime of the filter cartridge. Filters D, E, G, and H were assessed before (old cartridge) and after exchange of the filter cartridge (new cartridge).
2.3. AOX analysis
Analysis of AOX was performed as described previously21,22 and quantified as adsorbable organic chlorine (AOCl), bromine (AOBr), and iodine (AOI). Before AOX analysis, samples were acidified to approximately pH 2 using 70% HNO3 (10 μL per 10 mL sample) and quenched with a sodium sulfite solution (100 mM, 10 μL per 10 mL sample). Next, 10 mL was loaded on two consecutive activated carbon cartridges (40 mg activated carbon per glass column with 3 mm inner diameter; CPI International, California, USA). To minimize the loss of volatile analytes during AOX analyses, we used a 10 mL gas-tight glass syringe to sample the water without headspace and to load the sample onto the activated carbon cartridges without contact to ambient air. After enrichment, the cartridges were washed with 10 mL of 5 g L−1 nitrate as HNO3 to remove inorganic halides. We used a Mitsubishi AQF-2100 automated furnace unit to incinerate the activated carbon in the presence of oxygen for 260 seconds at 1000 °C. The gases from the pyrolysis process, containing the halide ions from the organic contaminants, were collected in a Mitsubishi GA-210 absorption unit containing 10 mL of absorption solution (ultrapure water with 0.003% hydrogen peroxide) resulting in a one-fold relative enrichment (i.e., diluted to original concentration). The absorption solution also contained phosphate (1 mg L−1) as internal standard to take into account volume variations of absorption solution injected into the IC by the absorption module. Next, 1000 μL of the absorption solution was injected into a Dionex ICS-2100 Ion Chromatograph (Thermo Fisher Scientific, Australia). A Dionex IonPac AS11-HC column (with IonPac AG11-HC guard column) was used with eluent generated by a Dionex Eluent Generator Cartridge III with 30–75 mM KOH, a flow rate of 1 mL min−1, and conductivity detection. The limits of detection were 1.9 μg L−1 (Cl−), 0.3 μg L−1 (Br−), and 0.7 μg L−1 (I−) and limits of quantification were 19 μg L−1 (Cl−), 3 μg L−1 (Br−) and 7 μg L−1 (I−). The coefficient of variation was below 10% between measurements. Recovery of five haloacetic acids was between 87 and 116%.23 AOX removal efficiency by the tested filters were calculated according to eqn (1). | 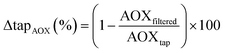 | (1) |
2.4. Fluoride analysis
We quantified fluoride after direct injection of water samples (1000 μL) into the Dionex ICS-2100 ion chromatograph with the same columns and sequence as mentioned in the previous section. The limit of quantification for fluoride analysis was 100 μg L−1 and thus well below the measured concentrations. The coefficient of variation between measurements was below 20%. Fluoride removal was calculated according to eqn (2). | 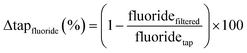 | (2) |
2.5. Bacteria counts
We used the flow cytometer Accuri C6 (BD Biosciences) to count bacteria in the water samples.24 For the bacteria counts we sampled water in sterilized glass vials with PTFE-lined screw caps. 500 μL from each sample was added to a 2 mL microtube (three replicates per sample). 5 μL of SYBR Green I nucleic acid stain (100× diluted from stock) was added, vortexed, and incubated in the dark for 10 minutes at approximately 40 °C. 50 μL of sample was measured at a flow rate of 30 μL min−1 using fluorescent detectors 1 (FL1: 530 ± 15 nm) and 3 (FL3: >670 nm). Data were collected as events per μL and gating was used to isolate the bacteria cell counts from the sample matrix. The system was back-flushed between samples to reduce contamination. Stained Milli-Q water was used with each sample run as a negative control. We calculated the bacteria removal by the tested filters as absolute values (counts per μL) according to eqn (3). Positive values indicate an increase in bacteria counts per μL. | Δtapbacteria(counts per μL) = bacteria countsfiltered − bacteria countstap | (3) |
2.6. Bioassays
For the bioanalytical assessment, water samples were acidified to pH 1.5 using sulfuric acid and solid phase extracted (SPE) with TELOS ENV cartridges (Kinesis, Australia; 1 g sorbent per 2 L of sample) as described previously.22 The 10
000-fold enriched SPE extracts were spiked to the bioassay medium at a maximum relative enrichment factor (REF) of 100 corresponding to a 1% solvent concentration (methanol) in the bioassays.
The bacterial cytotoxicity assay using Aliivibrio fischeri (formerly termed Vibrio fischeri) bioluminescence inhibition (Microtox) was selected because of its high responsiveness to DBPs.7 This Microtox assay was performed as described previously.25,26 The luminescence output of the bacteria was measured prior to addition of sample and after 30 min incubation. The luminescence inhibition was normalized to the solvent control and phenol served as positive control.
The Nrf2-ARE oxidative stress response pathway has been demonstrated in previous studies to be an important step in the toxicity pathway of mono-HAAs27 and appears to play a central role for the toxicity of many more DBPs.7 The AREc32 assay was performed as described previously.28 The used cell line is the human breast cancer cell line MCF7 stably transfected with an ARE reporter plasmid coupled to a luciferase reporter gene.29 The amount of produced luciferase is directly proportional to the ARE activated and thus also to the causative chemical stressor. We used t-butylhydroquinone (tBHQ) as positive control.30
The umuC assay with Salmonella typhimurium (TA1535/pSK1002) was selected as a bacterial screening tool for genotoxicity (Reifferscheid et al. 1991). This assay detects the activation of the cellular SOS-response, which is a global response to DNA damage to induce DNA repair mechanisms, and hence detects a response to genotoxic effects. The assay was performed without metabolic activation with S9 because in the umuC assay S9 addition leads to reduced genotoxicity for most DBPs7 and reduced effects in whole drinking water samples.31,32 4-Nitroquinoline-1-oxide (4NQO) served as positive control. For all assays we ran a negative control and a positive control on each multi-well plate for every experiment.
The assessment endpoint for the Microtox was the 50% effect concentration (EC50) derived from a log-logistic concentration-effect curve.33 For oxidative stress response (AREc32) and genotoxicity (umuC) we used the induction ratio (IR). IR is defined as the ratio of effect of the sample divided by the average effect observed in the controls. The effect concentration that elicits an IR of 1.5 (ECIR1.5; i.e., 1.5-fold or 50% effect increase compared to the negative control), is the assessment endpoint for these assays.33 We selected this benchmark value because for reporter gene assays often no maximum response can be obtained due to cytotoxic interferences.33,34 Therefore, we used the linear part of the concentration-effect curves up to an induction ratio (IR) of 5. IR is the ratio of measured signal to the signal of the negative control. We derived the ECIR1.5 by use of linear regression and selected the threshold of 1.5 because (i) it is employed in several guideline documents, e.g., OECD guideline for the umuC genotoxicity assay, (ii) it differs significantly from the negative control, and (iii) it can be derived when the maximum of the dose–response curve is not known.33 It has been demonstrated before that the ECIR1.5 is a statistically sound and robust benchmark value for the umuC,35 for the AREc32,30 and for the Geneblazer reporter gene assays.33 Removal of effect (Δtapbioassay) was calculated according to eqn (4).
| 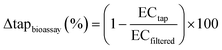 | (4) |
3. Results & discussion
3.1. Removal of adsorbable organic halogens (AOX)
We assessed the removal of AOX as sum parameter of halogenated organic compounds by the selected filtration devices (Fig. 1). At the time of sampling the filters were in use for differing durations (Table S1†). AOX was chosen because its concentration is often positively correlated with biological effects36 indicating that it is a useful parameter to assess the removal of toxicologically relevant contaminants.
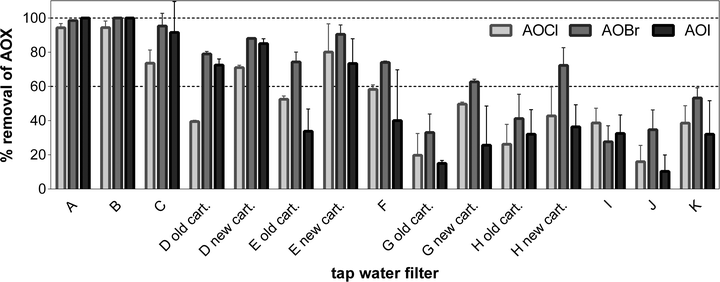 |
| Fig. 1 AOX removal by various water filters (Table S1;† A: reverse osmosis; B: ceramic outer shell with granular activated carbon and activated alumina core; C, E, F: ceramic outer shell with granular activated carbon core; G: activated carbon and ion-exchange resin; D, H, J, K: carbon block; I: activated alumina without carbon); for filters D, E, G, H results are given before (old cart.) and after replacement of the filter cartridge (new cart.). Displayed is the arithmetic mean ± standard deviation (n = 2–6). Detailed results are given in Table S2.† | |
As expected, reverse osmosis (filter A) was most effective for AOX removal together with the gravity filter B (contains activated carbon). Both filters removed >94% of AOCl, AOBr, and AOI (Table S2†). The portable filter C was slightly less effective with removal efficiencies >74%. Filter D was the most effective pressure filter. Its removal efficiency increased significantly after cartridge exchange (p < 0.05, one-way ANOVA with Tuckey's post-test), in particular for the more polar chlorinated organic compounds (AOCl, from 40 to 70%, filter D). The same is true for filter E where AOCl removal increased from 50 to 80% after cartridge exchange. Filter G was the least effective gravity filter in our study for the removal of AOX but again removal efficiency increased after cartridge exchange (for AOCl from 20 to 50%). AOX removal was least effective with the pressure filters H, I, J, and K. However, for filter H AOX removal increased after cartridge exchange (e.g., from 26 to 43% for AOCl).
For most filters, removal efficacy of AOBr was generally higher than for AOCl, which indicates that the sum of brominated organic compounds are likely less polar than chlorinated organic compounds, which facilitates sorption of AOBr to the carbon filter.37 This is an important aspect because brominated organic compounds are usually more potent in bioassays2,6 and hence their removal can be considered as more important. For AOI, the removal pattern was less consistent and the removal efficiencies were often lower than for AOBr. Iodinated organic compounds are commonly more toxic than their brominated analogues but the AOI concentration was approximately 10-times lower than AOBr in most cases (Table S2†).
3.2. Removal of fluoride
Fluoridation of drinking water is mandatory in our sampling areas and the detected fluoride concentrations matched the target concentration of the water providers (0.7–1 mg L−1).38,39 Only filters specifically designed for fluoride removal through addition of activated alumina to the filter matrix (filters B and I) as well as reverse osmosis (filter A) removed fluoride effectively by >83% (Fig. 2).
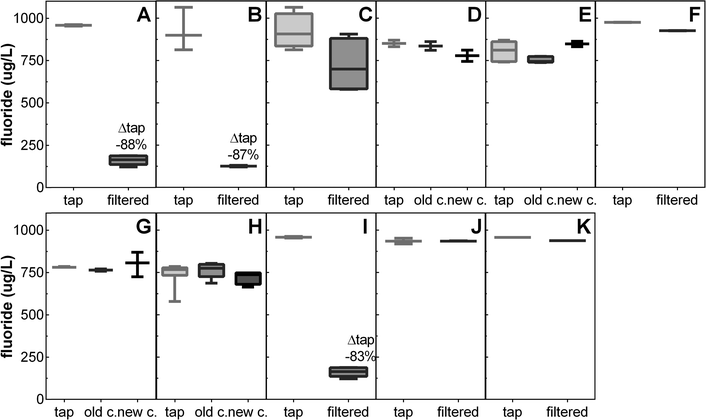 |
| Fig. 2 Fluoride concentrations before (tap) and after different water filters (Table S1;† A: reverse osmosis; B: ceramic outer shell with granular activated carbon and activated alumina core; C, E, F: ceramic outer shell with granular activated carbon core; G: activated carbon and ion-exchange resin; D, H, J, K: carbon block; I: activated alumina without carbon); for filters D, E, G, H results are given before (old c.) and after replacement of the filter cartridge (new c.). Displayed is the median, box extends from the 25th to the 75th percentiles, and the whiskers from minimum to maximum (n = 1–6). Detailed results are given in Table S2.† | |
In some countries, fluoride is added to the drinking water supply to improve dental health and in some regions, fluoride occurs naturally in water sources.40,41 Fluoride has been demonstrated to effectively reduce dental decay while it is often emphasized that fluoride acts topically (at the surface of the teeth) without a clear benefit in actually ingesting it.41 Also detrimental effects of high fluoride concentrations in drinking water (e.g., fluorosis) are documented and the range between beneficial and detrimental concentrations is very narrow.41–43 In geographical areas with high fluoride concentrations in drinking water, fluoride removal with PoU filters can reduce the prevalence of fluorosis syndrome43 but fluoride filters might also counteract tooth decay prevention measures in other regions.
3.3. Effect on bacteria counts
Bacterial counts in most tap water samples were within the range observed in previous studies (<200 μL−1).44–46 Bacteria counts were only effectively reduced with reverse osmosis (filter A) and filter D after cartridge exchange (>89% removal compared to tap water, Fig. S1†). For most filters, bacteria counts increased considerably after filtration, indicating biofilm growth in the filter medium. This is supported by the decreased bacteria counts after filter exchange (filter D, E, G, H). In our study we did not discriminate between pathogenic and non-pathogenic bacteria and hence increased bacteria counts are not necessarily of concern provided that no pathogens enter the filter medium. Biofilm on the sorbent material might even support removal of toxic organic contaminants from drinking water.47
3.4. Effect on cytotoxicity, oxidative stress, and genotoxicity
All tap water samples had to be enriched to exert biological effects in the bioassays. Relative enrichment factors of >3 were necessary to exceed the effect threshold for the most sensitive bioassay (AREc32, Table S4†). Most filters removed cytotoxic effects effectively by >60% compared to tap water except for filters G (pitcher filter) and I (pressure filter; Fig. 3). Reverse osmosis was most effective, followed by filter D (after cartridge exchange; pressure filter) and filter B (gravity filter). Filter cartridge exchange after the recommended exchange interval increased removal of cytotoxicity significantly for filters D, G, and H (p < 0.05, one-way ANOVA with Tukey's post-test).
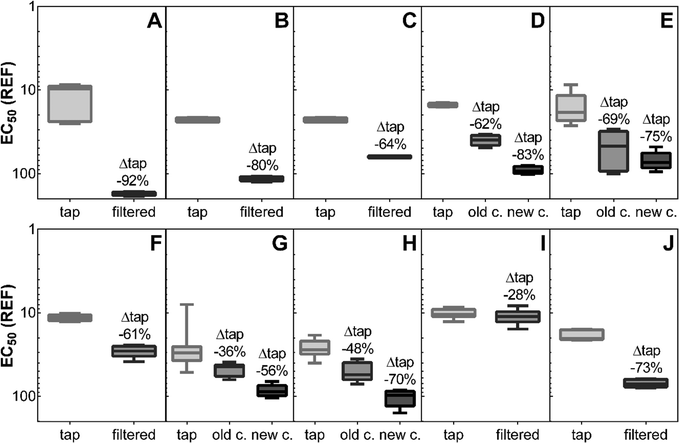 |
| Fig. 3 Cytotoxicity: 50% effect concentration (EC50) as relative enrichment factor (REF) before (tap) and after different water filters (Table S1;† A: reverse osmosis; B: ceramic outer shell with granular activated carbon and activated alumina core; C, E, F: ceramic outer shell with granular activated carbon core; G: activated carbon and ion-exchange resin; D, H, J: carbon block; I: activated alumina without carbon) assessed with the Aliivibrio fischeri bioluminescence inhibition assay; for filters D, E, G, H results are given before (old c.) and after replacement of the filter cartridge (new c.). Displayed is the median, box extends from the 25th to the 75th percentiles, and the whiskers from minimum to maximum (n = 4–10). Detailed results are given in Table S4.† | |
The oxidative stress response activation was reduced by >70% after filtration except for filters F, G, and I (Fig. 4). Again, reverse osmosis (A), filter B, and D (after cartridge exchange) were most effective at reducing biological effects by >97%. The pitcher filter G was least effective with 37% effect removal after cartridge exchange. Cartridge exchange increased the oxidative stress removal significantly for filters D, E, and H (p > 0.01, one-way ANOVA with Tukey's post-test).
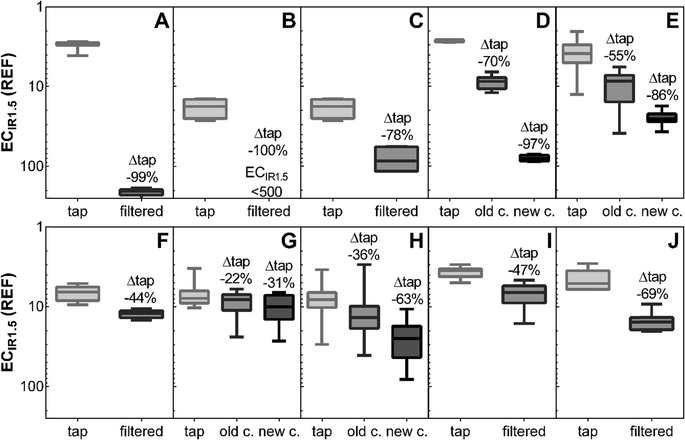 |
| Fig. 4 Activation of NRf2-mediated oxidative stress response assessed with the AREc32 assay: induction ratio of 1.5 (ECIR1.5) as relative enrichment factor (REF) before (tap) and after different water filters (Table S1;† A: reverse osmosis; B: ceramic outer shell with granular activated carbon and activated alumina core; C, E, F: ceramic outer shell with granular activated carbon core; G: activated carbon and ion-exchange resin; D, H, J: carbon block; I: activated alumina without carbon); for filters D, E, G, H results are given before (old c.) and after replacement of the filter cartridge (new c.). Displayed is the median, box extends from the 25th to the 75th percentiles, and the whiskers from minimum to maximum (n = 4–10). Detailed results are given in Table S4.† | |
All filters, except filter I, removed genotoxic effects by >60% (for filter D, G, and H only after cartridge exchange; Fig. S2†). Filters A, B, and E were most effective with removal efficiencies >85%. Cartridge exchange increased the genotoxicity removal significantly for filters E, G, and H (p > 0.01, one-way ANOVA with Tukey's post-test).
All of these assays detect toxicity caused by reactive compounds.7 The observed effect decrease after filtration demonstrates that water filters can effectively remove such toxicologically relevant compounds from tap water. However, the removal efficacy can vary considerably between 25 and 100%. Reverse osmosis (filter A) was most effective in most cases but also low cost activated carbon based gravity filters can effectively remove toxicologically relevant compounds. However, it is important to exchange the filter cartridge regularly according to recommendations of the manufacturer to maintain a good filter capacity. The only filter without a carbon-based sorbent was least effective for toxicity removal (filter I).
3.5. Reverse osmosis as best performing filter?
Reverse osmosis (RO) was one of the most effective systems to remove contaminants from tap water. This can be expected because RO is known to be one of the most efficient methods for trace organic contaminant removal from water (e.g. wastewater).33 However, RO also removes minerals and according to the World Health Organization, very large numbers of people consume levels of calcium and magnesium that are insufficient to support their physiological needs.48 Depending on the mineral content of drinking water, which varies greatly based on the geographical area, drinking water can contribute considerably to the calcium and magnesium intake and hence to the overall nutrition.49 In case of mineral-rich drinking water, mineral removal by RO filtration could counteract potential health benefits by DBP removal.50 Simple carbon-based gravity filters or pressure filters do not remove geogenic minerals because those are in ionic form and hence too polar to sorb to the filter material.
3.6. Alternative DBP mitigation strategies
Centralized DBP mitigation strategies would be most desirable to provide safe drinking water to the whole population and also to minimize dermal and inhalation exposure to DBPs during showering and bathing.2 The reduction of the DBP formation potential at drinking water treatment plants, e.g., via more effective removal of precursor compounds through physical-chemical treatment processes such as reverse osmosis or activated carbon filtration,51,52 would be very costly8 and hence unrealistic for most countries. In countries with newer and well-maintained drinking-water distribution systems, such as Switzerland, the Netherlands, Austria, and Germany, drinking water distribution is possible without residual disinfectants3,8,44 with similar or lower waterborne disease outbreaks compared to systems with residual disinfectant.3 This can be achieved if three factors are considered: (1) effective measures for water resources protection and water pollution control, (2) adapted multibarrier treatment including biological filtration to abate natural organic matter44,45 and (3) maintenance of the distribution system to avoid leakage.3 However, the required infrastructure investments are in the range of tens of millions USD for a 100
000 inhabitant city3 and hence, such a strategy is often out of reach for lower income countries. In contrast, it can be expected that the use of PoU filters is restricted to the more health-conscious and more prosperous part of the population. Considering approximate annual costs in the range of 50 USD for a well-working activated carbon-based PoU filter, centralized solutions might be more cost-efficient in the long run to provide safe drinking water to the whole population.
4. Conclusions
The water filters evaluated in this study effectively removed adsorbable organic halogenated compounds, a sum parameter for DBPs, by 28–94%. Bioassays indicative of cytotoxicity and reactive toxicity endpoints, such as oxidative stress and genotoxicity, revealed an effective removal of toxicologically relevant contaminants from tap water (i.e., reduction of effect concentration by 25–100%). Taking into account the potential increase in bladder cancer incidents through DBP exposure, the removal of DBPs from chlorinated tap water using point-of-use filters could be an important public health benefit.13
In our study, besides reverse osmosis, only filters with activated carbon removed organic contaminants and toxicity effectively, in particular low-cost gravity filters with a cartridge consisting of an outer ceramic shell with a granular activated carbon core. Filter cartridges need to be replaced on a regular basis to maintain good filter efficacy and to minimize bacterial growth in the sorbent material.
Nevertheless, centralized solutions would be most desirable for providing safe drinking water free of DBPs to the whole population without residual disinfectants in the distribution system. Investments in water resource protection, infrastructure renewal and maintenance, as well as well-controlled water treatment including disinfection and removal of natural organic matter, might be more cost-effective in the long run than point-of-use filtration to protect the whole population from detrimental health effects.
Acknowledgements
This research was supported by a Marie Curie International Outgoing Fellowship within the 7th European Community Framework Program (PIOF-GA-2012-329169), by Seqwater (bulk water supply provider in South East Queensland, Australia) and by the Australian Research Council (FT100100694 and DP140102672).
References
- E. W. Akin, J. C. Hoff and E. C. Lippy, Waterborne outbreak control - which disinfectant, Environ. Health Perspect., 1982, 46, 7–12 CrossRef CAS PubMed.
- S. D. Richardson, M. J. Plewa, E. D. Wagner, R. Schoeny and D. M. DeMarini, Occurrence, genotoxicity, and carcinogenicity of regulated and emerging disinfection by-products in drinking water: a review and roadmap for research, Mutat. Res., Rev. Mutat. Res., 2007, 636(1–3), 178–242 CrossRef CAS PubMed.
- F. L. Rosario-Ortiz, J. Rose, V. Speight, U. Von Gunten and J. Schnoor, How do you like your tap water? Safe drinking water may not need to contain a residual disinfectant, Science, 2016, 351(6276), 912–914 CrossRef CAS PubMed.
- J. J. Rook, Formation of haloforms during chlorination of natural waters, Water Treat. Exam., 1974, 23(2), 234–243 Search PubMed.
-
M. J. Plewa, E. D. Wagner, M. G. Muellner, K. M. Hsu and S. D. Richardson, Comparative mammalian cell toxicity of N-DBPs and C-DBPs, in Disinfection by-products in drinking water: occurrence, formation, health effects, and control, ed. T. Karanfil, S. W. Krasner and Y. Xie, American Chemical Society, Washington, 2008, vol. 995, pp. 36–50 Search PubMed.
- M. J. Plewa, J. E. Simmons, S. D. Richardson and E. D. Wagner, Mammalian cell cytotoxicity and genotoxicity of the haloacetic acids, a major class of drinking water disinfection by-products, Environ. Mol. Mutagen., 2010, 51(8–9), 871–878 CrossRef CAS PubMed.
- D. Stalter, E. O'Malley, U. von Gunten and B. I. Escher, Fingerprinting the reactive toxicity pathways of 50 drinking water disinfection by-products, Water Res., 2016, 91, 19–30 CrossRef CAS PubMed.
- D. L. Sedlak and U. von Gunten, The Chlorine Dilemma, Science, 2011, 331(6013), 42–43 CrossRef CAS PubMed.
- S. E. Hrudey and J. Fawell, 40 years on: what do we know about drinking water disinfection by-products (DBPs) and human health?, Water Sci. Technol.: Water Supply, 2015, 15(4), 667–674 CrossRef CAS.
- K. P. Cantor, C. M. Villanueva, D. T. Silverman, J. D. Figueroa, F. X. Real, M. Garcia-Closas, N. Malats, S. Chanock, M. Yeager, A. Tardon, R. Garcia-Closas, C. Serra, A. Carrato, G. Castano-Vinyals, C. Samanic, N. Rothman and M. Kogevinas, Polymorphisms in GSTT1, GSTZ1, and CYP2E1, disinfection by-products, and risk of bladder cancer in spain, Environ. Health Perspect., 2010, 118(11), 1545–1550 CrossRef CAS PubMed.
- S. E. Hrudey, Chlorination disinfection by-products, public health risk tradeoffs and me, Water Res., 2009, 43(8), 2057–2092 CrossRef CAS PubMed.
- C. M. Villanueva, K. P. Cantor, S. Cordier, J. J. K. Jaakkola, W. D. King, C. F. Lynch, S. Porru and M. Kogevinas, Disinfection byproducts and bladder cancer - A pooled analysis, Epidemiology, 2004, 15(3), 357–367 CrossRef PubMed.
- R. Odom, S. Regli, M. Messner, J. Cromwell and M. Javdan, Benefit–cost analysis of the stage 1D/DBP rule, J. - Am. Water Works Assoc., 1999, 91(4), 137–147 CAS.
- C. M. Villanueva, M. Kogevinas, S. Cordier, M. R. Templeton, R. Vermeulen, J. R. Nuckols, M. J. Nieuwenhuijsen and P. Levallois, Assessing Exposure and Health Consequences of Chemicals in Drinking Water: Current State of Knowledge and Research Needs, Environ. Health Perspect., 2014, 122(3), 213–221 Search PubMed.
- S. W. Krasner, H. S. Weinberg, S. D. Richardson, S. J. Pastor, R. Chinn, M. J. Sclimenti, G. D. Onstad and A. D. Thruston, Occurrence of a new generation of disinfection byproducts, Environ. Sci. Technol., 2006, 40(23), 7175–7185 CrossRef CAS PubMed.
- J. M. Wright, P. A. Murphy, M. J. Nieuwenhuijsen and D. A. Savitz, The impact of water consumption, point-of-use filtration and exposure categorization on exposure misclassification of ingested drinking water contaminants, Sci. Total Environ., 2006, 366(1), 65–73 CrossRef CAS PubMed.
-
Korea-Herald, Drink tap water? ‘No way,’ say Koreans - Health concerns linger despite state assurance, The Korea Herald 2015, 26.05.2015 (http://www.koreaherald.com/view.php?ud=20150526000906).
- M. B. Rahman, T. Driscoll, M. Clements, B. K. Armstrong and C. T. Cowie, Effects of tap water processing on the concentration of disinfection by-products, J. Water Health, 2011, 9(3), 507–514 CrossRef CAS PubMed.
- S. Leuesque, M. J. Rodriguez, J. Serodes, C. Beaulieu and F. Proulx, Effects of indoor drinking water handling on trihalomethanes and haloacetic acids, Water Res., 2006, 40(15), 2921–2930 CrossRef PubMed.
- A. I. Egorov, A. A. Tereschenko, L. M. Altshul, T. Vurtiainen, D. Samsonov, B. LaBrecque, J. Maki-Paakkanen, N. L. Drizhd and T. E. Fordb, Exposures to drinking water chlorination by-products in a Russian city, Int. J. Hyg. Environ. Health, 2003, 206(6), 539–551 CrossRef CAS PubMed.
- M. J. Farre, B. A. Lyon, G. A. de Vera, D. Stalter and W. Gernjak, Assessing adsorbable organic halogen formation and precursor removal during drinking water production, J. Environ. Eng., 2016, 142(3), 04015087 CrossRef.
- D. Stalter, L. D. Peters, E. O'Malley, J. Y. M. Tang, M. Revalor, M. J. Farre, K. Watson, U. von Gunten and B. I. Escher, Sample enrichment for bioanalytical quality assessment of disinfected drinking water: concentrating the polar, the volatiles, the unknowns, Environ. Sci. Technol., 2016, 50(12), 6495–6505 CrossRef CAS PubMed.
- R. Y. L. Yeh, M. J. Farré, D. Stalter, J. Y. M. Tang, J. Molendijk and B. I. Escher, Bioanalytical and chemical evaluation of disinfection by-products in swimming pool water, Water Res., 2014, 59, 172–184 CrossRef CAS PubMed.
- P. A. Neale and B. I. Escher, Does co-extracted dissolved organic carbon cause artefacts in cell-based bioassays?, Chemosphere, 2014, 108, 281–288 CrossRef CAS PubMed.
- D. Stalter, M. Dutt and B. Escher, Headspace-free setup of in vitro bioassays for the evaluation of volatile disinfection by-products, Chem. Res. Toxicol., 2013, 26, 1605–1614 CrossRef CAS PubMed.
- J. Y. M. Tang, S. McCarty, E. Glenn, P. A. Neale, M. S. J. Warne and B. I. Escher, Mixture effects of organic micropollutants present in water: Towards the development of effect-based water quality trigger values for baseline toxicity, Water Res., 2013, 47(10), 3300–3314 CrossRef CAS PubMed.
- J. Pals, M. S. Attene-Ramos, M. H. Xia, E. D. Wagner and M. J. Plewa, Human cell toxicogenomic analysis linking reactive oxygen species to the toxicity of monohaloacetic acid drinking water disinfection byproducts, Environ. Sci. Technol., 2013, 47(21), 12514–12523 CrossRef CAS PubMed.
- B. I. Escher, C. van Daele, M. Dutt, J. Y. M. Tang and R. Altenburger, Most oxidative stress response in water samples comes from unknown chemicals: the need for effect-based water quality trigger values, Environ. Sci. Technol., 2013, 47(13), 7002–7011 CAS.
- X. J. Wang, J. D. Hayes and C. R. Wolf, Generation of a stable antioxidant response element-driven reporter gene cell line and its use to show redox-dependent activation of Nrf2 by cancer chemotherapeutic agents, Cancer Res., 2006, 66(22), 10983–10994 CrossRef CAS PubMed.
- B. I. Escher, M. Dutt, E. Maylin, J. Y. M. Tang, S. Toze, C. R. Wolf and M. Lang, Water quality assessment using the AREc32 reporter gene assay indicative of the oxidative stress response pathway, J. Environ. Monit., 2012, 14(11), 2877–2885 RSC.
- M. J. Farré, S. Day, P. A. Neale, D. Stalter, J. Y. M. Tang and B. I. Escher, Bioanalytical and chemical assessment of the disinfection
by-product formation potential: Role of organic matter, Water Res., 2013, 47(14), 5409–5421 CrossRef PubMed.
- P. A. Neale, A. Antony, M. E. Bartkow, M. J. Farre, A. Heitz, I. Kristiana, J. Y. M. Tang and B. I. Escher, Bioanalytical assessment of the formation of disinfection byproducts in a drinking water treatment plant, Environ. Sci. Technol., 2012, 46(18), 10317–10325 CAS.
- B. I. Escher, M. Allinson, R. Altenburger, P. A. Bain, P. Balaguer, W. Busch, J. Crago, N. D. Denslow, E. Dopp, K. Hilscherova, A. R. Humpage, A. Kumar, M. Grimaldi, B. S. Jayasinghe, B. Jarosova, A. Jia, S. Makarov, K. A. Maruya, A. Medvedev, A. C. Mehinto, J. E. Mendez, A. Poulsen, E. Prochazka, J. Richard, A. Schifferli, D. Schlenk, S. Scholz, F. Shiraish, S. Snyder, G. Y. Su, J. Y. M. Tang, B. van der Burg, S. C. van der Linden, I. Werner, S. D. Westerheide, C. K. C. Wong, M. Yang, B. H. Y. Yeung, X. W. Zhang and F. D. L. Leusch, Benchmarking organic micropollutants in wastewater, recycled water and drinking water with in vitro bioassays, Environ. Sci. Technol., 2014, 48(3), 1940–1956 CrossRef CAS PubMed.
-
B. I. Escher and F. D. L. Leusch, in Bioanalytical tools in water quality assessment, IWA Publishing, London, 2012 Search PubMed.
- M. Macova, S. Toze, L. Hodgers, J. F. Mueller, M. Bartkow and B. I. Escher, Bioanalytical tools for the evaluation of organic micropollutants during sewage treatment, water recycling and drinking water generation, Water Res., 2011, 45(14), 4238–4247 CrossRef CAS PubMed.
- B. A. Lyon, R. Y. Milsk, A. B. DeAngelo, J. E. Simmons, M. P. Moyer and H. S. Weinberg, Integrated Chemical and Toxicological Investigation of UV-Chlorine/Chloramine Drinking Water Treatment, Environ. Sci. Technol., 2014, 48(12), 6743–6753 CrossRef CAS PubMed.
- A. M. Kennedy, A. M. Reinert, D. R. U. Knappe, I. Ferrer and R. S. Summers, Full- and pilot-scale GAC adsorption of organic micropollutants, Water Res., 2015, 68, 238–248 CrossRef CAS PubMed.
-
Seqwater, Fluoridation of the water supply. Fluoride Information Sheet Seqwater., http://www.seqwater.com.au/sites/default/files/PDF%20Documents/Publications/20130107-Fluoride-information-sheet.pdf 2011.
-
MNCAHS, Mid North Coast Area Health Service Fluoride brochure, http://www.coffsharbour.nsw.gov.au/places-for-living/drinking-water-supply/water-treatment/Pages/fluoridation.aspx 2004.
- R. B. Johnston, M. Berg, C. A. Johnson, E. Tilley and J. G. Hering, Water and Sanitation in Developing Countries: Geochemical Aspects of Quality and Treatment, Elements, 2011, 7(3), 163–168 CrossRef CAS.
- S. Ayoob and A. K. Gupta, Fluoride in drinking water: A review on the status and stress effects, Crit. Rev. Environ. Sci. Technol., 2006, 36(6), 433–487 CrossRef CAS.
- A. Bretzler and C. A. Johnson, The Geogenic Contamination Handbook: Addressing arsenic and fluoride in drinking water, Appl. Geochem., 2015, 63, 642–646 CrossRef CAS.
- C. A. Johnson, M. Berg and D. Sabatini, Towards sustainable safe drinking water supply in low-and middle-income countries: The challenges of geogenic contaminants and mitigation measures Foreword, Sci. Total Environ., 2014, 488, 479–480 Search PubMed.
- F. Hammes, C. Berger, O. Koster and T. Egli, Assessing biological stability of drinking water without disinfectant residuals in a full-scale water supply system, J. Water Supply: Res. Technol.--AQUA, 2010, 59(1), 31–40 CrossRef CAS.
- J. El-Chakhtoura, E. Prest, P. Saikaly, M. van Loosdrecht, F. Hammes and H. Vrouwenvelder, Dynamics of bacterial
communities before and after distribution in a full-scale drinking water network, Water Res., 2015, 74, 180–190 CrossRef CAS PubMed.
- P. Lipphaus, F. Hammes, S. Kotzsch, J. Green, S. Gillespie and A. Nocker, Microbiological tap water profile of a medium-sized building and effect of water stagnation, Environ. Technol., 2014, 35(5), 620–628 CrossRef CAS PubMed.
- J. Reungoat, B. I. Escher, M. Macova and J. Keller, Biofiltration of wastewater treatment plant effluent: Effective removal of pharmaceuticals and personal care products and reduction of toxicity, Water Res., 2011, 45(9), 2751–2762 CrossRef CAS PubMed.
-
S. A. Atkinson, R. Costello and J. M. Donohue, Overview of global dietary calcium and magnesium intakes and allowances, Calcium and Magnesium in drinking-water: public health significance, WHO Library Cataloguing-in-Publication Data, 2009, pp. 17–36, ISBN 978 92 4 156355 0 (http://whqlibdoc.who.int/publications/2009/9789241563550_eng.pdf) Search PubMed.
-
C. N. Ong, A. C. Grandjean and R. P. Heaney, The mineral composition of water and its contribution to calcium and magnesium intake, Calcium and Magnesium in drinking-water: public health significance, WHO Library Cataloguing-in-Publication Data, 2009, pp. 37–58, ISBN 978 92 4 156355 0 (http://whqlibdoc.who.int/publications/2009/9789241563550_eng.pdf) Search PubMed.
- H. Zeng, W. Q. Shu, J. A. Chen, L. Liu, D. H. Wang, W. J. Fu, L. Q. Wang, J. H. Luo, L. Zhang, Y. Tan, Z. Q. Qiu and Y. J. Huang, Experimental comparison of the reproductive outcomes and early development of the offspring of rats given five common types of drinking water, PLoS One, 2014, 9(10), e108955 Search PubMed.
- K. Watson, M. J. Farre and N. Knight, Comparing a silver-impregnated activated carbon with an unmodified activated carbon for disinfection by-product minimisation and precursor removal, Sci. Total Environ., 2016, 542, 672–684 CrossRef CAS PubMed.
- K. Watson, M. J. Farré and N. Knight, Enhanced coagulation with powdered activated carbon or MIEX® secondary treatment: A comparison of disinfection by-product formation and precursor removal, Water Res., 2015, 68, 454–466 CrossRef CAS PubMed.
Footnote |
† Electronic supplementary information (ESI) available: Additional figures and tables are available in the ESI file. See DOI: 10.1039/c6ew00068a |
|
This journal is © The Royal Society of Chemistry 2016 |
Click here to see how this site uses Cookies. View our privacy policy here.