The intramolecular H-bonding effect on the growth and stability of Schiff-base surface covalent organic frameworks†
Received
8th October 2016
, Accepted 23rd November 2016
First published on 23rd November 2016
Abstract
The introduction of intramolecular H-bonding by adding –OH functionalities adjacent to the Schiff base centers is considered to be a useful strategy to enhance the stability and crystallinity of bulk covalent organic frameworks (COFs). However, the influence of intramolecular H-bonding on the synthesis of surface COFs (SCOFs) have been barely explored. Herein, SCOFs based on the Schiff-base reaction between 1,3,5-tris(4-aminophenyl)benzene (TAPB) and terephthalaldehydes with symmetry or asymmetrically substituted hydroxyl functional groups are designed. In the absence of a solvent, hydroxyl substituents can be easily oxidized; thus argon protection is required to obtain high-quality SCOFs. Besides, an extended network with uniform pores can be achieved in spite of the symmetry of substituents. Both experimental results and theoretical calculations show that the influence of intramolecular hydrogen bonding on surface synthesis is not as important as that in bulk phase synthesis because the substrate itself can lead to the complanation of adsorbed molecules. The existence of intramolecular H-bonding can enhance the stability of the network in both acid and alkali environments.
1 Introduction
Covalent organic frameworks (COFs) are a class of crystalline porous polymers with predesigned skeletons and nanopores, and are constructed with organic units via strong covalent bonds.1–3 Since Yaghi and co-workers achieved the first successful synthesis of COFs in 2005,4 the design and synthesis of COFs as promising materials for catalysis, gas adsorption and storage, sensing, electronic and photoelectric applications have attracted enormous interest.1,2 Synthesis of COFs with high quality and stability is crucial in order to realize their various functions, and the introduction of intramolecular H-bonding has been proved to be an efficient approach to influence the fabrication of COFs. By choosing aldehyde molecules containing hydroxyl substituents, the crystallinity of the material can be enhanced distinctly.5–7 It is believed that the intramolecular O–H⋯N
C hydrogen bond can suppress the torsion of the edge units and lock the 2D sheets in a planar conformation, leading to improved crystallinity. Furthermore, the presence of intramolecular H-bonding enhances the chemical stability of COFs as well, especially the hydrolytic stability in acid or base solution.5,7
Two-dimensional porous covalent networks synthesized through on-surface reactions are called surface covalent organic frameworks (SCOFs).8 SCOFs are ideal models to study the growth mechanism and properties of the COFs, since the structural details of SCOFs on the surface can be resolved at the molecular level by scanning tunnel microscopy (STM). In addition, unlike bulk COF materials, SCOFs are ideally one-atom thick, which are promising to display interesting dimension-related properties. Hence, a great deal of effort has been devoted to obtain SCOFs.9–26 However, the influence of intramolecular H-bonding on the synthesis of SCOFs is still poorly studied. Herein, we report the synthesis of SCOFs by the reaction between 1,3,5-tris(4-aminophenyl)benzene (TAPB) and 2,3-dihydroxyterephthalaldehyde (2,3-DHTA); 2,5-dihydroxyterephthalaldehyde (2,5-DHTA) (Scheme 1). We aim at exploring the influence of hydroxyl functional groups on the synthesis of SCOFs, especially the effect of intramolecular H-bonding interaction on the quality and the stability of the SCOFs. Highly ordered surface covalent organic frameworks are obtained under argon protection. Because of the complanation effect of the substrate itself, the influence of intramolecular hydrogen bonding on molecular planarity is not as important as that on bulk phase synthesis, but has a vital effect on the stability.
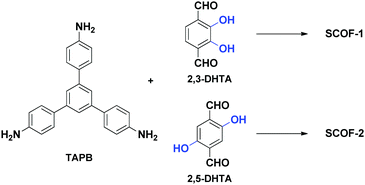 |
| Scheme 1 Molecular structures of monomers used in this study: 1,3,5-tris(4-aminophenyl)benzene (TAPB); 2,3-dihydroxyterephthalaldehyde (2,3-DHTA); and 2,5-dihydroxyterephthalaldehyde (2,5-DHTA). | |
2 Experimental section
2.1 Materials
1,3,5-Tris(4-aminophenyl)benzene was purchased from J&K. 2,3-Dihydroxyterephthalaldehyde and 2,5-dihydroxyterephthalaldehyde were synthesized according to the procedures reported previously. Their 1H NMR and 13C NMR spectra agree well with the spectra reported in the related literature.27,28 All chemicals used in this study were used without any further purification.
2.2 Preparation and characterization of samples
In a typical procedure of sample preparation, two building blocks (TAPB and 2,3-dihydroxyterephthalaldehyde/2,5-dihydroxyterephthalaldehyde) were mixed in THF with a molar ratio of about 2
:
3 and a molar concentration of about 10−5 mol L−1. Then a droplet (∼5 μL) of solution was deposited on the surface of freshly cleaved HOPG. After the evaporation of THF, HOPG was transferred into a 100 mL Teflon-sealed autoclave with CuSO4·5H2O (∼1.1 g) particles, which serve as thermodynamic regulation agents.15 The heating temperature for 2,3-dihydroxyterephthalaldehyde was 110 °C in an Ar atmosphere and 100 °C without Ar protection. While for 2,5-dihydroxyterephthalaldehyde, the optimal heating temperature is 110 °C with or without an Ar atmosphere. The designated reaction time was 3 h. STM images were recorded using the NanoscopeIIIa SPM (Bruker Nano) with mechanically cut Pt/Ir wires (90/10) under ambient conditions. All of the images were obtained in constant-current mode and are shown without further processing. FFT spectra of the STM images were recorded using Nanoscope software.
2.3 Stability experiment
A drop of HCl or KOH solution with specific pH (∼50 μL) was added onto the surface with the prepared SCOFs and the droplet covered the entire surface. After standing for 5 minutes, the droplet was removed and the sample surface was observed by STM.
2.4 Computational methods
The adsorption energy of conceivable networks obtained by the reaction between TAPB and 2,3-DHTPA due to the asymmetrical substitution of the hydroxyl functional group was calculated by Forcite using the Dreiding force field. The geometry optimizations of the models in Fig. 3 were performed by dispersion-corrected density functional theory (DFT-D) using the DMol3 code. Standard parameter set of LDA-PWC potential functionals was adopted without any parameter optimization.
3 Results and discussion
3.1 Synthesis of SCOF-1
We first investigated the Schiff-base reaction between 1,3,5-tris(4-aminophenyl)benzene (TAPB) and 2,3-dihydroxyterephthalaldehyde (2,3-DHTA). TAPB and 2,3-DHTA were mixed in THF with a molar ratio of 2
:
3 and then drop-casted onto the highly oriented pyrolytic graphite (HOPG) surface. The reaction was carried out at 100 °C for 3 h. Fig. 1a and b depict the STM images of SCOFs after the reaction. The surface is covered by a disordered 2D polymeric network composed of honeycomb networks of SCOF-1, polygon grids, unclosed pores and irregular oligomers. On further increasing the reaction temperature to 110 °C, the amount of ordered network decreased; when the temperature increases to 120 °C, we can hardly find six-membered rings on the surface (Fig. S1, ESI†), indicating that the higher reaction temperature is not conducive to the growth of the network. We ascribe this phenomenon to the oxidation of the hydroxyl substituents.29 In order to inhibit oxidation, the reaction was carried out under argon protection at 110 °C for 3 h. After cooling to ambient conditions, an extend honeycomb network with significantly improved quality is observed by STM (Fig. 1c). The fast Fourier transform (FFT) spectrum of the image in the inset shows well-defined six-fold symmetry. Fig. 1d displays a high-resolution STM image of SCOF-1. A network with uniform pores can be observed and the pore size is measured to be 3.8 ± 0.2 nm by structural analysis, which is in agreement with the expected size of 3.91 nm by structural modelling, confirming the covalent bond formation of imine-linked SCOF-1. Due to the asymmetrical substitution of the hydroxyl functional group, several conceivable networks can be formed by the reaction between TAPB and 2,3-DHTA. They have the same pore size but different hydroxyl orientations at the sides of six-membered rings (Table S1, ESI†). Since one can hardly distinguish the hydroxyl orientation of each side from STM images, theoretical calculations are implemented. Table S1 (ESI†) shows the structural models of conceivable networks and the corresponding adsorption energy. The energy differences among each structural model are within the range of 6.5 kcal mol−1 and Fig. 1e displays the structural model of SCOF-1 with the lowest adsorption energy. The details of the hydrogen bonding sites are shown in the inset of Fig. 1e. Both the hydroxyl groups in 2,3-DHTA form hydrogen bonds and the two imine bonds connected with 2,3-DHTA point to the same side with respect to the molecular axis (cis-conformation).
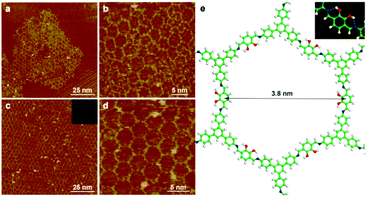 |
| Fig. 1 (a and b) Large scale and high resolution STM images of SCOF-1 on HOPG obtained by heating at 100 °C for 3 h without Ar protection. (c and d) Large scale and high resolution STM images of SCOF-1 on HOPG formed after heating at 110 °C for 3 h in an Ar atmosphere with the inset depicting the corresponding FFT spectrum of the STM image. (e) A structural model with the measured structural parameter for SCOF-1. The inset of (e) is partially magnified structural model depicting the hydrogen bonding sites of SCOF-1. Imaging conditions: Vbias = 700 mV, It = 500 pA. | |
3.2 Synthesis of SCOF-2
Similarly, we can obtain SCOF-2 by the condensation reaction of TAPB and 2,5-DHTA (the equation is shown in Scheme 1). Fig. 2a reveals a typical large-scale STM image obtained by heating SCOF-2 at 110 °C for 3 h without Ar protection. The high resolution STM image (Fig. 2b) discloses imperfection of the network. Apart from a few six-membered rings, the surface is covered by polygon grids, unclosed pores and irregular oligomers caused by incomplete condensation as well. By employing the argon gas protection, the synthesis of SCOF-2 with improved order is achieved successfully (Fig. 2c). The inset of Fig. 2c is the FFT spectrum of the image, which discloses the six-fold symmetry of the network. The structural details of SCOF-2 are illustrated in the high-resolution STM image shown in Fig. 2d. A uniform pore spacing of 3.8 nm and a small amount of unclosed pores are observed. The measured pore size agrees well with the expected size of 3.91 nm by structural modeling, which confirms covalent bond formation. The structural model of SCOF-2 is shown in Fig. 2e with the inset depicting the hydrogen bonding sites on one side of the network. In this case, both the hydroxyl groups in 2,5-DHTA form hydrogen bonds and the two imine bonds connected with molecular 2,5-DHTA keep trans-conformation.
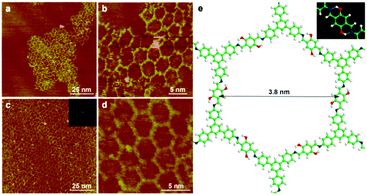 |
| Fig. 2 (a and b) Large scale and high resolution STM images of SCOF-2 on HOPG obtained by heating at 110 °C for 3 h without Ar protection. (c and d) Large scale and high resolution STM images of SCOF-2 on HOPG formed after heating at 110 °C for 3 h in an Ar atmosphere with the inset depicting the corresponding FFT spectrum of the STM image. (e) A structural model with the measured structural parameter for SCOF-2. The inset of (e) is partially magnified structural model depicting the hydrogen bonding sites of SCOF-2. Imaging conditions: Vbias = 700 mV, It = 500 pA. | |
3.3 Effect of intramolecular H-bonding
To further understand the role of intramolecular H-bonding in SCOFs synthesis, we conduct the control experiment with TAPB and terephthalaldehyde. Extended networks with good quality can be achieved without the hydroxyl substituents (Fig. S2, ESI†), which indicates that intramolecular H-bonding is not indispensable to obtain the highly ordered SCOFs. It is known that the intramolecular hydrogen bonds can enhance material crystallinity in solution phase synthesis of bulk COFs by influencing molecular planarity.5–7 To gain a deeper insight into the influence of intramolecular H-bonding on molecular planarity in the case of surface synthesis, we select a typical segment including a C
N bond as a model for the theoretical calculation. All of the geometry optimizations were carried out using DMol3. The distances (O–H⋯N) of 2.51 Å with the angle of 151.1° are measured in Fig. 3b and 2.51 Å; 151.5° in Fig. 3d, which demonstrate the existence of intramolecular hydrogen bonds.30–33Fig. 3a shows an optimized structural model of the reaction product of 5′-phenyl-[1,1′:3′,1′′-terphenyl]-4-amine and benzaldehyde in free space. The measured dihedral angle of C
N in the model compound is 148.61°. When introducing a hydroxyl substituent into the model, the measured dihedral angle value changes to 152.65° (Fig. 3b), which demonstrates that the existence of intramolecular hydrogen bonding can restrain the torsion of the molecular plane. This result is consistent with previous reports.5,6 As we synthesize COFs on the surface, the influence of the substrate must be taken into account. Next, the model compound is optimized with the presence of a fixed graphite substrate. The measured dihedral angle value on the substrate is 161.23° (Fig. 3c), which discloses that the influence of the substrate can markedly improve the molecular planarity as well. When the influence of both substrate and intramolecular H-bonding interaction is present, the measured dihedral angle in the corresponding model is 168.43° (Fig. 3d). The changes in the dihedral angle from Fig. 3a and b and Fig. 3c and d are 4.04°, 7.20°, respectively, which show the contribution of intramolecular H-bonding interaction on molecular planarity. Compared with that of the substrate (the differences from Fig. 3a–c and Fig. 3b–d are 12.62°, 15.78°), we believe that the influence of the substrate has a major contribution to the molecular planarity in the case of on-surface synthesis.
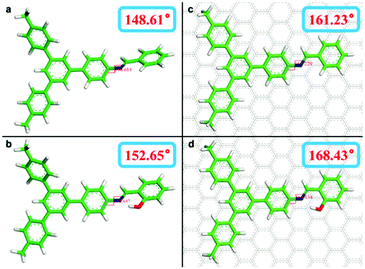 |
| Fig. 3 DMol3 optimized geometries with measured dihedral angle values. (a) A DMol3 optimized structural model in free space. (b) The influence of intramolecular H-bonding interaction on molecular planarity. (c) The influence of the substrate on molecular planarity. (d) The influence of both substrate and intramolecular H-bonding interaction on molecular planarity. The substrate is displayed as a single layer of graphite in the figure for clarity. | |
Then we focus on the influence of intramolecular H-bonding on SCOF stability. Nonfunctionalized SCOFs are obtained by the reaction between TAPB and terephthalaldehyde, which has the same skeleton but no intramolecular H-bonding interaction compared to SCOF-1. Fig. 4a and e reveal the STM images of pristine SCOFs. Both reactions have successfully synthesized a continuous network with high quality (high resolution images are shown in the blue boxes at the top-right corner). The measured pore size of regular networks obtained by the reaction between TAPB and terephthalaldehyde is 3.8 ± 0.2 nm, which is consistent with the value in our previous work.18 After 1 mol L−1 HCl treatment, the network of SCOF-1 is almost entirely destroyed by acid solution and only disorderly adsorption can be observed on the surface (Fig. 4b). After 0.01 mol L−1 HCl treatment, most of the network can be retained (Fig. 4c). From the high resolution STM images we find that the network after treatment still has a high degree of order. However, in the case of nonfunctionalized SCOFs, the network has been destroyed thoroughly after either 1 mol L−1 or 0.01 mol L−1 HCl treatment (Fig. 4f and g). Hence, the existence of intramolecular hydrogen bonding can enhance SCOF stability in acidic environment significantly. In an alkaline environment, although two kinds of SCOFs have been damaged to some extent after 0.01 mol L−1 KOH treatment, the destruction of the nonfunctionalized SCOF is more severe (Fig. 4d and h). Therefore, the SCOF containing intramolecular H-bonding is more stable in the alkaline environment as well. The stability experiment of SCOF-2 has also been implemented (Fig. S3, ESI†), with experimental results similar to those of SCOF-1. To summarize, the existence of intramolecular H-bonding can obviously enhance the generated network stability in both acid and alkali environments.
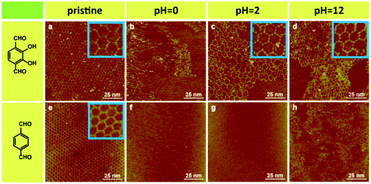 |
| Fig. 4 The stability study of SCOFs synthesized by TAPB and 2,3-DHTA or terephthalaldehyde. (a and e) The pristine STM images of the generated networks; (b and f) the STM images of networks after 1 mol L−1 HCl treatment; (c and g) the STM images of networks after 0.01 mol L−1 HCl treatment; and (d and h) the STM images of networks after 0.01 mol L−1 KOH treatment. The corresponding high resolution STM images are shown in the insets of Fig. a and c–e. Imaging conditions: (a) Vbias = 700 mV, It = 500 pA; (b) Vbias = 700 mV, It = 500 pA; (c) Vbias = 630 mV, It = 495 pA; (d) Vbias = 630 mV, It = 495 pA; (e) Vbias = 630 mV, It = 500 pA; (f) Vbias = 660 mV, It = 545 pA; (g) Vbias = 700 mV, It = 500 pA; (h) Vbias = 630 mV, It = 500 pA. | |
4 Conclusions
In summary, we have explored the influence of hydroxyl functional groups on the synthesis of covalent organic frameworks on the surface by the reaction between TAPB and terephthalaldehydes with symmetry or asymmetrically substituted hydroxyl functional groups. The easily oxidized properties of the hydroxyl substituents in the absence of a solvent make the synthesized SCOFs suffer from small domain sizes and large amounts of defects. Once the reaction was carried out under argon protection, high quality SCOFs were obtained. Besides, an extended network with uniform pores can be achieved in spite of the symmetry of substituents. DFT simulation results indicate that because of the complanation effect of the substrate itself, the influence of intramolecular hydrogen bonds on surface synthesis is not as important as that on bulk phase synthesis. However, the existence of intramolecular H-bonding can significantly enhance the stability of SCOFs in both acid and alkali environments.
Acknowledgements
This work is supported by the National Natural Science Foundation of China (Grants 21433011, 91527303, 21373236, 21573252, 21127901) and the Strategic Priority Research Program of the Chinese Academy of Sciences (Grant no. XDB12020100).
References
- X. Feng, X. Ding and D. Jiang, Chem. Soc. Rev., 2012, 41, 6010–6022 RSC.
- S. Y. Ding and W. Wang, Chem. Soc. Rev., 2013, 42, 548–568 RSC.
- P. J. Waller, F. Gandara and O. M. Yaghi, Acc. Chem. Res., 2015, 48, 3053–3063 CrossRef CAS PubMed.
- A. P. Cote, A. I. Benin, N. W. Ockwig, M. O'Keeffe, A. J. Matzger and O. M. Yaghi, Science, 2005, 310, 1166–1170 CrossRef CAS PubMed.
- S. Kandambeth, D. B. Shinde, M. K. Panda, B. Lukose, T. Heine and R. Banerjee, Angew. Chem., Int. Ed., 2013, 52, 13052–13056 CrossRef CAS PubMed.
- X. Chen, M. Addicoat, E. Jin, L. Zhai, H. Xu, N. Huang, Z. Guo, L. Liu, S. Irle and D. Jiang, J. Am. Chem. Soc., 2015, 137, 3241–3247 CrossRef CAS PubMed.
- S. Kandambeth, V. Venkatesh, D. B. Shinde, S. Kumari, A. Halder, S. Verma and R. Banerjee, Nat. Commun., 2015, 6, 6786–6795 CrossRef CAS PubMed.
- X. H. Liu, C. Z. Guan, D. Wang and L. J. Wan, Adv. Mater., 2014, 26, 6912–6920 CrossRef CAS PubMed.
- N. A. Zwaneveld, R. Pawlak, M. Abel, D. Catalin, D. Gigmes, D. Bertin and L. Porte, J. Am. Chem. Soc., 2008, 130, 6678–6679 CrossRef CAS PubMed.
- R. Gutzler, H. Walch, G. Eder, S. Kloft, W. M. Heckl and M. Lackinger, Chem. Commun., 2009, 4456–4458 RSC.
- M. O. Blunt, J. C. Russell, N. R. Champness and P. H. Beton, Chem. Commun., 2010, 46, 7157–7159 RSC.
- O. Ourdjini, R. Pawlak, M. Abel, S. Clair, L. Chen, N. Bergeon, M. Sassi, V. Oison, J.-M. Debierre, R. Coratger and L. Porte, Phys. Rev. B: Condens. Matter Mater. Phys., 2011, 84, 125421 CrossRef.
- J. R. F. Dienstmaier, D. D. Medina, M. Dogru, P. Knochel, T. Bein, W. M. Heckl and M. Lackinger, ACS Nano, 2012, 6, 7234–7242 CrossRef CAS PubMed.
- T. Faury, S. Clair, M. Abel, F. Dumur, D. Gigmes and L. Porte, J. Phys. Chem. C, 2012, 116, 4819–4823 CAS.
- C. Z. Guan, D. Wang and L. J. Wan, Chem. Commun., 2012, 48, 2943–2945 RSC.
- A. C. Marele, R. Mas-Balleste, L. Terracciano, J. Rodriguez-Fernandez, I. Berlanga, S. S. Alexandre, R. Otero, J. M. Gallego, F. Zamora and J. M. Gomez-Rodriguez, Chem. Commun., 2012, 48, 6779–6781 RSC.
- Y. Q. Zhang, N. Kepcija, M. Kleinschrodt, K. Diller, S. Fischer, A. C. Papageorgiou, F. Allegretti, J. Bjork, S. Klyatskaya, F. Klappenberger, M. Ruben and J. V. Barth, Nat. Commun., 2012, 3, 1286–1293 CrossRef PubMed.
- X. H. Liu, C. Z. Guan, S. Y. Ding, W. Wang, H. J. Yan, D. Wang and L. J. Wan, J. Am. Chem. Soc., 2013, 135, 10470–10474 CrossRef CAS PubMed.
- L. Xu, X. Zhou, Y. Yu, W. Q. Tian, J. Ma and S. Lei, ACS Nano, 2013, 7, 8066–8073 CrossRef CAS PubMed.
- X. H. Liu, Y. P. Mo, J. Y. Yue, Q. N. Zheng, H. J. Yan, D. Wang and L. J. Wan, Small, 2014, 10, 4934–4939 CrossRef CAS PubMed.
- L. Xu, X. Zhou, W. Q. Tian, T. Gao, Y. F. Zhang, S. Lei and Z. F. Liu, Angew. Chem., Int. Ed., 2014, 53, 9564–9568 CrossRef CAS PubMed.
- X. H. Liu, C. Z. Guan, Q. N. Zheng, D. Wang and L. J. Wan, J. Chem. Phys., 2015, 142, 101905 CrossRef PubMed.
- L. Xu, L. Cao, Z. Guo, Z. Zha and S. Lei, Chem. Commun., 2015, 51, 8664–8667 RSC.
- J.-Y. Yue, X.-H. Liu, B. Sun and D. Wang, Chem. Commun., 2015, 51, 14318–14321 RSC.
- Z. Gong, B. Yang, H. Lin, Y. Tang, Z. Tang, J. Zhang, H. Zhang, Y. Li, Y. Xie, Q. Li and L. Chi, ACS Nano, 2016, 10, 4228–4235 CrossRef CAS PubMed.
- X.-H. Liu, J.-Y. Yue, Y.-P. Mo, Y. Yao, C. Zeng, T. Chen, H.-J. Yan, Z.-H. Wang, D. Wang and L.-J. Wan, J. Phys. Chem. C, 2016, 120, 15753–15757 CAS.
- S. Akine, T. Taniguchi and T. Nabeshima, J. Am. Chem. Soc., 2006, 128, 15765–15774 CrossRef CAS PubMed.
- J.-I. Kadokawa, Y. Tanaka, Y. Yamashita and K. Yamamoto, Eur. Polym. J., 2012, 48, 549–559 CrossRef CAS.
- F. G. Bordwell and J. Cheng, J. Am. Chem. Soc., 1991, 113, 1736–1743 CrossRef CAS.
- A. Halder, S. Kandambeth, B. P. Biswal, G. Kaur, N. C. Roy, M. Addicoat, J. K. Salunke, S. Banerjee, K. Vanka, T. Heine, S. Verma and R. Banerjee, Angew. Chem., Int. Ed., 2016, 55, 7806–7810 CrossRef CAS PubMed.
- D. B. Shinde, S. Kandambeth, P. Pachfule, R. R. Kumar and R. Banerjee, Chem. Commun., 2015, 51, 310–313 RSC.
- H. Nazır, M. Yıldız, H. Yılmaz, M. Tahir and D. Ülkü, J. Mol. Struct., 2000, 524, 241–250 CrossRef.
- A. Simperler, H. Lampert and W. Mikenda, J. Mol. Struct., 1998, 448, 191–199 CrossRef CAS.
Footnote |
† Electronic supplementary information (ESI) available: Structural models of conceivable networks obtained by reaction between TAPB and 2,3-DHTPA as well as supplementary STM figures. See DOI: 10.1039/c6cp06894d |
|
This journal is © the Owner Societies 2017 |