DOI:
10.1039/C5BM00332F
(Review Article)
Biomater. Sci., 2016,
4, 40-54
Biomedical applications of nano-titania in theranostics and photodynamic therapy
Received
21st August 2015
, Accepted 21st September 2015
First published on 7th October 2015
Abstract
Titanium dioxide (TiO2) is one of the most abundantly used nanomaterials for human life. It is used in sunscreen, photovoltaic devices, biomedical applications and as a food additive and environmental scavenger. Nano-TiO2 in biomedical applications is well documented. It is used in endoprosthetic implants and early theranostics of neoplastic and non-neoplastic maladies as a photodynamic therapeutic agent and as vehicles in nano-drug delivery systems. Herein, we focus on the recent advancements and applications of nano-TiO2 in bio-nanotechnology, nanomedicine and photodynamic therapy (PDT).
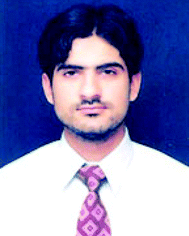 F. U. Rehman | Fawad Ur Rehman has been a Ph.D. candidate in the School of Biological Science and Medical Engineering, State Key Lab of Bioelectronics, Southeast University, Nanjing, China since 2013. Before this, he got his Masters degree from The University of Agriculture, Peshawar and his Bachelors degree (DVM) in Veterinary Medicine and Surgery from the University of Veterinary and Animal Sciences, Lahore, Pakistan. Currently, his interest is in bio-imaging and photodynamic therapy with nano-TiO2 and porphyrin derivatives for various disease theranostics, including rheumatism, cancer, and stem cell therapeutics. |
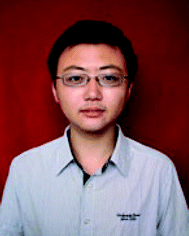 C. Zhao | Chunqiu Zhao was born in 1990. He obtained his B.E. degree from Central South University, China. He has been enrolled in the School of Biological Science and Medical Engineering, Southeast University, China for a doctoral degree since 2013. His research interests are the application of nano materials for bio-imaging and photodynamic therapy. |
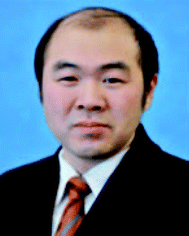 H. Jiang | Dr Hui Jiang is an associate professor of biomedical engineering at Southeast University. He obtained his Ph.D. (Chemistry) from the University of Science and Technology of China in 2005. After a postdoctoral fellowship at Nanjing University, he joined the State Key Laboratory of Bioelectronics, Southeast University in 2007. His research focuses on nano-based electro-chemiluminescent biosensors and the electron transfer behaviors of the micro–nano interface. |
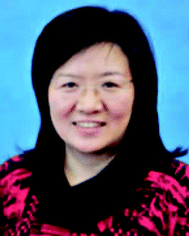 X. Wang | Dr Xuemei Wang is currently a full professor of biomedical engineering at Southeast University. She obtained her Ph.D. in Chemistry from Nanjing University, China, in 1994 and became a lecturer at Nanjing University in 1995. She was an Alexander von Humboldt Fellow in 1997 in the Chemistry Department, University of Saarland, Germany, and then she joined the State Key Laboratory of Bioelectronics, Southeast University in 1998. Her research focuses on bioelectronics and biosensors, nanomedicine, bio-imaging and highly sensitive diagnosis strategies for cancers and other related diseases. |
1. Introduction
The role of nanotechnology (NT) in our life is inevitable. Naturally, the existence of bio-nanotechnology (BNT) can be traced back to the initiation of first life on earth. The inter- and intra- cellular transfer of nanosized biomolecules (e.g. low density lipoproteins), neural transmission and memory storage in biological systems are the prototype examples of BNT.1,2 Similarly, biomolecules like proteins, DNA and RNA are on the nano-scale and are structural analogues to nanomaterials, which introduces the concept of integrating nanomaterials with biomolecules to understand the cellular signal pathways, functional mechanisms and cellular interactions within living cells and organisms.3 In nature, dust particles, smoke and ink are examples of non-biological NT.4 BNT existed from the beginning of life and its potential has been explored with the advancement in human civilization by realizing the great impact BNT has on our day to day life. Similarly, enzymes, antigens, antibodies and ligand receptors with 2–20 nm sizes in either direction, are structural analogues to nanomaterials, and thus they provide plenty of scope for BNT biomedical applications.5,6
The distinctive properties of fabricated inorganic-nanomaterials (INM), including their high reactivity, increased surface area, lower melting point, ductility and charge conductivity, make them suitable candidates for biomedical applications and BNT.7,8 Since these INM can readily interact with organic biomolecules by exhibiting excellent conductivity and site recognition within cellular gadgetry, they can be reintroduced to cells and biological systems. Mostly, the physico-chemical properties, including the shape,9 size10 and surface chemistry,11 decide the fate of the INM. Surface modification of INM leads to relevant changes in the physico-chemical properties that make them suitable for certain disease treatments, e.g. –COOH functionalized MWCNT nanowhiskers (NW) are more hydrophilic and less toxic than pristine or untreated nanoparticles and whiskers.12
Titanium is one of the most abundantly available elements in nature with the most common forms being the metallic state of T, TiCl4 and titanium dioxide (TiO2); the latter one exists with well-known crystalline shapes of rutile, brookite, TiO2-B and the most reactive – anatase.13–15 It has also been proven that mixed polymorphs of TiO2 are more efficient for biomedical applications than single crystals, e.g. anatase 80% and rutile 20% are more efficient than anatase 100%,13 similarly TiO2-B1 and anatase have been reported as being more reactive.16,17 These polymorph additive effects can be attributed to the surface interface among various polymorphs, reducing the recombination of photo-generated electron hole pairs and thus resulting in more ROS generation.13
Currently, more than thirteen hundred nanomaterials are globally available,18 among them TiO2 is the second most abundantly consumed material in our daily life.19 Food grade TiO2 is named as E171 in the European Union.20 In the US, the daily consumer statistics reveal that every child under the age of ten years is exposed to 1–2 mg of TiO2 per kgbody weight (bwt), whereas the exposure rate for other age groups is 0.2–0.7 mg per kgbwt.20 TiO2 was used as a white pigment in ancient times,21 and its long term exposure to humans and the environment guarantees its less toxic nature.
Despite the extensive use of TiO2 in other fields, the history of TiO2 in the biomedical field is relatively new. The first nano-TiO2 biomedical application can be traced back to the early nineties of the 20th century, when Japanese scientist Fujishima and his coworkers reported that TiO2 had photodynamic therapeutic properties by killing cancer cells (HeLa).22 At the same time another study reported the successful application of nano-TiO2 in medical implants by coating TiO2 as a redox agent on their surface.23 Soon after, these reports on TiO2 caused momentum in the biomedical applications field and the number of publications and citations exponentially increased by the start of the 21st century. In this review we will mainly focus on the most recent advancements of nano-TiO2 and its bio-applications in the biomedical realms.
2. Nanotechnology and bio-application of TiO2
Nanotechnology (NT) is based on engineering science at the molecular level and is used in biomedicine for therapeutics and diagnostics, which are collectively termed as “Theranostics”.24 Nano-drug delivery systems, bio-imaging, photodynamics, sonodynamics and photothermal therapy are among the few vital applications of BNT. The European Union defines a nanomaterial as a “manufactured, incidental or natural material containing particles as an aggregate, agglomerate or an unbound; where fifty percent exhibit 1–100 nm size in any dimension”.14 Generally, the size of the nanoparticle (NP) must be in the range of 1–100 nm in any dimension, as described by the National Nanotechnology Initiative; however the term “Nano” describes the size in several hundred nanometers. Their tiny nano-size is advantageous for crossing easily through various cell and tissue barriers with desired effects. NP lodging in desired sites depends upon the unique microenvironment, i.e. temperature, pH, ROS, metabolic rate, osmotic pressure, etc.25 In some cases NPs are conjugated to antibodies or active ligands, like peptides and folic acid, that are highly specific to achieve sufficient accumulation in the desired tissue. This approach is known as an active mechanism,26 similarly most of the neoplastic tissues bear enhanced vascular permeability and retention (EPR) effects that can be termed as a passive mechanism to achieve the desired nano-drug concentration27,28 (Fig. 1).
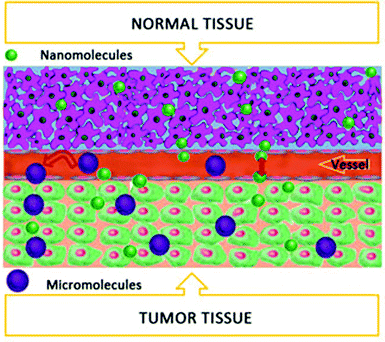 |
| Fig. 1 Schematic presentation of enhanced permeability and retention (EPR) effects in tumor tissue. The nanomolecules can easily cross the blood capillary endothelial cells whereas micromolecules can only stay in the tumor tissue due to the larger intercellular space compared to normal tissue. | |
As one of the most abundantly used nanomaterials, titanium dioxide (TiO2) has been widely utilized as a food additive and environmental scavenger, as well as in sunscreen, photovoltaic devices, and biomedical treatments. When TiO2 is irradiated with a certain light band (i.e. UV), it will generate electron–hole pairs that react with the surrounding oxygen to form various reactive oxygen species (ROS), i.e. OH− or O2− radicals, by redox reactions.29 Waste water purification via TiO2 by utilizing the natural sun light is the most valuable application in our day to day life.30,31 In fact, the photo-generated radicals are used as sanitizers for polluted water containing various dyes and phenols, or for other organic pollutants in the environment.29,32 It has been reported that due to the wide band gap, TiO2 photo absorption is limited to the UV light spectrum, i.e. 4 percent of the total sun light, however, metal ions and nonmetal doping can extend TiO2 photo absorption to the visible range.33 The aforementioned approaches are the key features of nano-TiO2 for biological applications and modern theranostics. Mostly, the nano-TiO2 applications depend upon the type of polymorph used (e.g. one dimensional or three dimensional particles), surface modification, size of the particle and the subject system, i.e. biological, photoelectrical, etc.34
2.1 Nano-TiO2 fabrication
Various techniques have been used so far for the fabrication of nanomaterials, including the sol–gel method, hydrothermal processes, chemical vapor deposition and electrospinning.35,36 The later method is more favorable for the fabrication of one dimensional TiO2 nanomaterials, e.g. nanowhiskers, nanofibers, nanobelts, necklace-like nanofibers and nanowires, etc.30,37 Moreover, the electrochemical anodization technique is considered more favorable for nanotube synthesis, in addition to the chemical template synthesis and alkaline hydrothermal treatment,38 and allows great control over the dimensions during the synthesis.39,40 The structure of TiO2 nanotubes is not clearly defined, however, it is assumed that hydrogen titanate is present where the TiO2 sheets are separated by H+ ions.41
TiO2 nanowhiskers are better than particles for photocatalytic activity42 and charge transportation, which makes them suitable candidates for solar cells and windscreens.43 Moreover, the better charge recombination rate and oxygen affinity of nano-TiO2 make it a suitable remedy for environmental recycling and an efficient catalyst.44
TiO2 nanowhiskers are also known for their synergistic effect, which is attributed to their photocatalytic activity, that makes TiO2 an excellent phototherapeutic agent. Moreover, its large surface area and reactivity provide scaffolds for excellent drug delivery systems. Recently, García-Valverde et al. reported the successful coating of carbon on TiO2 nanotubes for improved application in biomedical realms.45 Similarly, the synergistic effect for carrying anticancer drugs like daunorubicin (DNR) to cancer cells (i.e. hepatic carcinoma cells SMMC-7721) and the PD therapeutic effect when irradiated with UV light, mean that TiO2 nanowhiskers could play important roles in future cancer and other disease theranostics46 (Fig. 2).
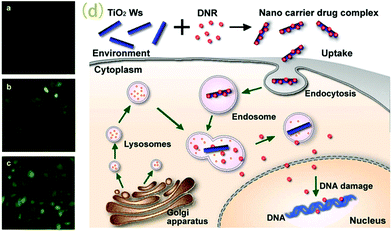 |
| Fig. 2 Fluorescence microscopic results showing SMMC-7721 cancer cells treated with DNR (a), DNR–TiO2 nanoparticles (b), and DNR–TiO2 nanowhiskers (c). (d) represents the possible mechanism for cellular uptake of TiO2 nanowhiskers in SMMC-7721 cancer cells. Adapted from ref. 46. Copyright 2009 Elsevier Ltd. | |
2.2 Nano-TiO2 pharmacodynamics
TiO2 nanoparticles are commonly used in industry as consumer products and for biomedical applications due to their strong catalytic activity and smaller size, which provides a large surface area per unit mass and a maximum number of electrons on the surface to enhance their reactivity.14 The safe dose for TiO2 nanoparticles reported by previous studies is 5 g per kgbwt in rat models, with no adverse effects and rapid distribution when administered systemically.47,48 TiO2 nanoparticles can be administered orally, as an aerosol or by systemic parenterals (intravenous, intramuscular or sub-cutaneous) without any adverse effects with the exception of less to none transdermal penetration when applied topically.49 It is evident that the liver, spleen, kidney and lungs are the main organs for TiO2 nanoparticle deposition, while blood cells, plasma and the brain are least affected.50 Nevertheless, recently it has been reported that 5 nm TiO2 nanoparticles as intraperitoneal injections for 14 days and 80–155 nm particles in the transnasal location can cross the blood–brain barrier51–54 (Fig. 3).
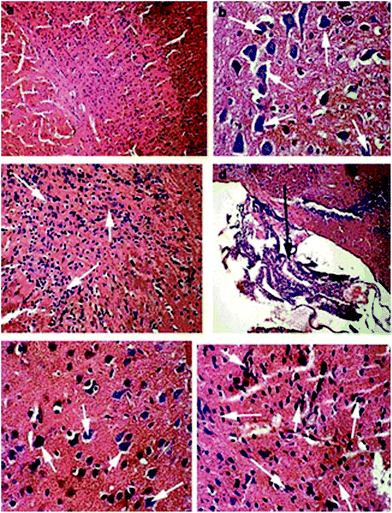 |
| Fig. 3 Brain tissue histopathology (H&E stain) after 60 days of TiO2 nanoparticle administration in ICR mice. Here (a) shows normal brain tissue at 100×; (b) shows 5 mg per kgbwt TiO2 nanoparticle administration resulting in higher calcium deposition in the brain tissue (400×); (c) shows 10 mg per kgbwt TiO2 nanoparticle administration resulting in spongiocyte proliferation indicating pathological lesions (200×); (d) shows 10 mg per kgbwt TiO2 nanoparticle administration indicating proliferating ependyma (100×); (e) shows 50 mg per kgbwt TiO2 nanoparticle administration indicating calcium deposition (400×) whereas (f) shows the 50 mg per kgbwt TiO2 administered group with spongiocyte proliferation. Adapted from ref. 52. Copyright 2010 Elsevier Ltd. | |
3. Biomedical applications of nano-TiO2
3.1 Photodynamic treatment
Photodynamic therapy (PDT) is a therapeutic technique with three main components, i.e. a photosensitizer (PS), a light source and an oxygen molecule. A nontoxic photosensitizer (i.e. a macromolecular compound55 or nano-sized organic56 or inorganic particle57) can be introduced into living tissues and cells. These PSs are excited by the absorption of photons from the visible light source and transfer energy to the oxygen molecule to produce singlet oxygen (1O2) and reactive oxygen species (ROS).58,59 PDT has more advantages over the conventional theranostics for cancer, i.e. it has a higher safety record, is minimally invasive, is cost effective, and is highly localized with low to no complications.60
PDT is most effective in delicate areas and natural cavities such as the bladder, pulmonary tissues, head and neck, brain, pancreas, prostate, mammary tissue and intraperitoneal cavity, where invasive surgery always carries a great deal of risk.61–63 PDT is still an ideal candidate and first choice for dermal neoplastic and non-neoplastic maladies. Moreover, the first ever therapeutic effect of PDT was for dermal malignancies.64 PDT is more effective for the treatment of tiny and superficial tumors, e.g. on internal body organ linings, above and just below the skin and exposed body cavities (i.e. oral, vagina, rectum, urethra, bladder, etc.). In deep seated tumors, its efficacy is low due to less penetration of the excitation light to generate an ample therapeutic quantity of singlet oxygen (1O2) and other reactive oxygen species (ROS). PDT’s potential against dermal and oral microbial infections (e.g. halitosis) has been proven to be more efficient.65
The early diagnosis of benign and malignant tumors is still a challenge for modern therapeutics and the only available treatment is invasive surgery, chemotherapy or radiotherapy. Hence, fluorescence imaging provides a suitable alternative for early diagnosis and PDT for cancer66,67 as well as for other infectious diseases.68,69 This fluorescence imaging and therapy as a combination can also be termed as “photodynamic therapy” (PDT).70 At the beginning of the 20th century, PDT was rediscovered by Oskar Raaband Hermannvon Tappeiner, although in ancient China, Egypt and the subcontinent PDT was already well established for dermal infections and as a natural sanitizer for households.65,71 The role of PDT is not only vital in onco-therapy but also in non-neoplastic cardiovascular,72–74 pulmonary,75,76 dermal,77,78 ophthalmic,79 oral59,80 and inflammatory diseases along with rheumatoid arthritis as an autoimmune reaction suppressant.81–83 Mostly, photosensitizers and drug delivery systems are sensitive to UV light in the range of less than 400 nm wavelength,84 however, this range has limited penetration (because of the short wavelength) and can damage the DNA as an adverse effect.85,86 For deep tissue penetration, the near infrared (NIR) light range (620–850 nm) is more favored because of the less harmful and maximum penetration properties.87 The NIR light falls in the phototherapeutic window that ranges from 600 to 1000 nm.88
Photosensitive nanocomposites like TiO2 nanoparticles could be administered systemically to the living tissue and upon attaining a certain concentration in the desired location, visible light or NIR light is passed through the subject tissue. These NPs (PSs) absorb the light in the form of a photon and transfer it to local oxygen containing molecules that release various kinds of ROS and 1O2. When light energy is passed through the PS, the ground state oxygen is excited to 1O2 and then to the triplet oxygen (3O2) energy state (Fig. 4).86 The half-life of singlet oxygen within the living tissue is estimated to be ∼3 μseconds.89–91 Moreover, once the PS is photoactivated it produces ROS for 18 hours, which is sufficiently long enough to induce apoptosis within the target cell and adverse effects in the surrounding environment.92
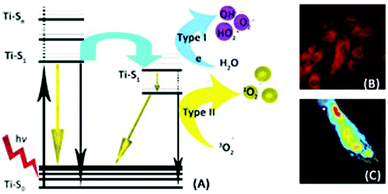 |
| Fig. 4 Schematic presentation of ROS and singlet oxygen generation from TiO2 when photoactivated with UV range light (A). Fluorescence generated from nano-TiO2 after photoactivation in fibroblast cells, in vitro (B) and in vivo in a rheumatoid arthritis foot in a murine model (C). | |
Pristine nano-TiO2 is susceptible to photoactivation under UV range light (400 nm).93 The WHO approved therapeutic window starts at a relatively higher wavelength (more than 600 nm) due to the fact that proteins, haemoglobin and melanin are also excited under UV range light, which could cause severe adverse effects.94 To make pristine TiO2 amenable for PDT desired effects, it should be either coupled with up-converting surface modifiers or coated with materials of the desired range. Synthetic or naturally occurring agents that upon excitation with certain light produce reactive oxygen species (ROS) are termed as “photosensitizers” (PSs).95,96 PSs are the main and most important components of PDT.83,97 Porphyrin derivatives are the most common and prototype PSs98,99 that are composed of macromolecules with excellent potential for singlet oxygen generation and the least known toxicity.100,101 Porfimer sodium is an example of an approved PS in the US by the Food and Drug Administration (FDA) for clinical applications, whereas zinc(II) phthalocyanine is an example of a second generation of clinically approved PSs, with high tumor cell selectivity and higher reactivity to generate −O2.60
Most of the PSs are hydrophobic in nature, which impedes their parental administration. This bottleneck develops the concept for hydrophilic nano-PSs, such as micelles, liposomes, low density lipoproteins,102–105etc. Nevertheless, these PSs’ hypersensitivity reactions and accumulation in the skin and eyes are the leading factors for severe phototoxicity.106 Therefore, to overcome these snags, the concept of nano-drug delivery systems is promising due to the hydrophilic nature and the safe delivery to the target tissue. Most of the drug delivery systems are nano-silica based, organically modified57 or selected metallic and non-metallic PSs, e.g. gold,107,108 silver,109 zinc,110,111 carbon fibers and complexes,112,113 platinum,114,115 titanium,116 iron,117,118 cerium,119 ruthenium,120etc., and/or their hybrid clusters.121–124
Recently NT combined inorganic materials with organic macromolecules as PSs to achieve their synergistic effects in PDT.125,126 Over the last few decades, the potential of nano-TiO2 as an inorganic PS for PDT has been extensively explored.127 Nano-TiO2 as a PD-therapeutic agent has become popular for various disease therapies, including for cancer128,129 and microbial infections,130 and in food safety and sanitation.131 The reason for using TiO2 as a PS in PDT is that it becomes super hydrophilic when exposed to UV light and this functionality can be reversed, depending upon the light it is exposed to.132
Hou et al. designed NIR light up-converting TiO2 nanoparticles to explore their potential in PDT for cancer amelioration.133 They used NaYF4:Yb3+,Tm3+@NaGdF4:Yb3+ as up-conversion nanoparticles (UNCP) to form UNCP@TiO2 for the photoactivation of TiO2 nanoparticles by NIR light. When these UNCP@TiO2 were introduced into cancer cells in vitro and photoactivated by NIR light, the TiO2-generated ROS induced apoptosis by decreasing the mitochondrial membrane potential, which releases cytochrome C to activate caspase3 for apoptosis initiation in cancer cells. Furthermore, in vitro, these UNCP@TiO2 also suppressed tumor growth in murine models (Fig. 5).
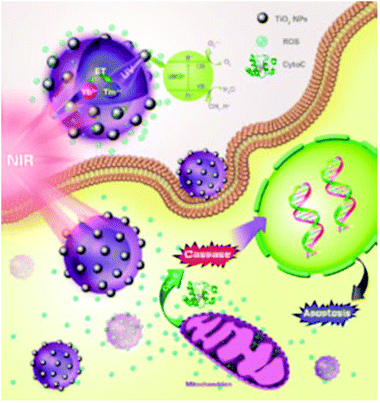 |
| Fig. 5 Near infrared light activated TiO2 nanoparticles damage mitochondria to release the cyto C enzyme for caspase activation to induce apoptosis in target cells during photodynamic therapy. Reprinted with permission from ref. 133. Copyright 2015 American Chemical Society. | |
Nano-TiO2 has also been used in combination with other nanomaterials and macromolecules where it exhibits excellent synergistic effects, e.g. Zheng et al. used TiO2–Fe3O4 nano-composites for neoplastic disease theranostics, where nano-TiO2 is used as a PS for PDT and Fe3O4 is used as a contrast agent for Magnetic Resonance Imaging (MRI).127 Tokuoka et al. used chlorine e6 in combination with TiO2 nanoparticles for the murine thymic lymphoma cancer cell line (EL-4). The nano-TiO2 combined with chlorine e6 had a significant effect on the cell line after photoactivation in the visible range compared with separate treatment with TiO2 and chlorine e6.134In vitro these results could be achieved, but in vivo biomedical applications may not be promising due to the weak electrostatic adsorption force of nano-TiO2 that systemically interacts with the plasma proteins in circulation. Although this concept is challenging, our group recently successfully delivered tetra sulphonatophenyl porphyrin (TSPP) to inflamed joints by TiO2 nanowhiskers in photodynamic therapy for rheumatoid arthritis early diagnosis by fluorescence bio-imaging and treatment.135
3.2 Sonodynamic therapy with nano-TiO2
Recently, ultrasound activated sensitizers received attention and gained popularity for the remedy of deep seated tumors and other infectious diseases, due to the deep penetration ability of ultrasound radiation. In 1989, Umemura et al. were the first to investigate the concept of sonodynamic therapy by ultrasonically activating hematoporphyrin, which was a well known photosensitizer back then.136
Most of the sonosensitizers used are organic macromolecules (porphyrin derivatives, phthalocyanines, 5-aminolevulinic acid, etc.) that produce ROS upon ultrasonic activation.137–139 Harada and his co-workers found that TiO2 nanoparticles activation by ultrasound radiation could be utilized to treat neoplastic melanoma C32 in vitro as well in vivo.140 They activated nano-TiO2 by 1 MHz, 1.0 W cm−2 for two minutes and found a significant decrease in the volume and growth suppression of neoplastic tissue (Fig. 6).
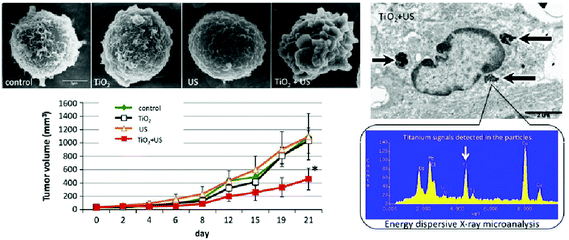 |
| Fig. 6 Sonodynamic therapy with nano-TiO2 to induce apoptosis in cancer cells. Reprinted with permission from ref. 140. Copyright 2011 Elsevier B.V. | |
Shen et al. combined Fe3O4@TiO2 (core@shell) nanoparticles and a chemotherapeutic agent, i.e. doxorubicin (DOX), to evaluate its potential for the successful delivery to neoplastic tissue and this is termed “chemo-sonodynamic therapy”.141 They found that TiO2 irradiation with ultrasound generated the ROS and ensured the safe delivery and retention of the chemotherapeutic agent post intravenous injections in murine models. Shen et al. also used Fe3O4@TiO2 nanoparticles for sonodynamic therapy of cancer cells and found more than 50% reduction in cancer cell viability.142 The synergistic effect in chemo-sonodynamic therapy of NaYF4–Fe3O4@TiO2 nanocomposites with a hyaluronic acid coating for cancer was explored by Shen et al.143 They reported the successful uptake of DOX loaded nanocomposites by MCF-7 and KB cells in vitro. In xenograft models, the combined synergistic inhibitory effect of chemo-sonodynamic therapy was 88.36% as compared to 38.91% and 28.36% for separate sonodynamic and chemotherapy, respectively.
Yamaguchi et al. reported TiO2–PEG nanoparticles and explored their sonodynamic and photodynamic therapeutic effects on human glioblastoma cells U251. They activated the TiO2–PEG sonosensitizers at 5.0 mW cm−2 and evaluated the effect by MTT assay.144 Their results demonstrate the promising role of TiO2–PEG sonosensitizers since they significantly decrease the viability and membrane integrity in the subject tumor cell line.
Ninomiya et al. reported S1/S2 protein mobilized TiO2 nanoparticles for sonodynamic therapy of HepG2 cancer cells in vitro145 and xenograft tumor murine models in vivo.146 Ultrasonic activated TiO2 nanoparticles activated at 0.5 MHz and 1 MHz for 60 seconds induced apoptosis and reduced viability by 46% in the HepG2 cells. Similarly, when 0.1 mg of TiO2 nanoparticles were directly injected into tumor tissue, five times in 13 days, the tumor was reduced within 28 days.
1O2 singlet oxygen generation by TiO2 nanoparticles is well known after photoactivation. Recently, Harada et al. also reported 1O2 oxygen generation by TiO2 nanoparticles with core–shell type polyallylamine micelles. When these TiO2 containing micelles were introduced into HeLa cancer cells, they generated 1O2 along with other ROS after sonoactivation.147 These findings are very important and can revolutionize sonodynamic therapeutics in terms of bio-imaging and theranostics, especially for neoplastic maladies.
3.3 Nano-TiO2 for medical implants
Medical implants have revolutionized orthopedic surgery, especially endoprosthetic surgeries. However, autoimmune reactions against the orthopaedic implants and cardiovascular stents hamper their liberal application. Various metallic combinations have been used so far, but Ti and its various alloys, especially nano-TiO2 surface coated implants, are reported to be the most promising for reducing autoimmune reactions. The thin passivation layer of nano-sized TiO2 minimizes the autoimmune reaction between the underlying bone tissue surfaces and the implant.148
TiO2 nanotube implants are used in prosthetic articular surgery of orthopedic patients with tibia-tarsal joints (knee) or acetabulum in hip joint fractures, however, their success rate is not very good due to degradation of the materials or promotion of the generation of chronic inflammation within the implanted tissues.149,150 Nevertheless, some studies suggest that nano-TiO2 provides more safe scaffolds for tissue recognition in endo-prosthetic surgery.151,152 Many studies have reported that TiO2 nanotubes enhance the bone mineralization, osteoblast adhesion in vitro, and strong bone adhesion in vivo.153–155
In biomedical applications, especially in orthopaedic plating156,157 and stenting in the cardiovascular system (CVS), it was found that when the surface was coated with nano-TiO2 or submicron particles, the macrophage chemotaxis was appreciably reduced and the chances of thrombosis as a post-operative complication were delayed (one of the leading causes of stenosis and complications in CVS stenting).158–160 Ti-6Al-4V (Ti-Alloy) is the most common alloy used in medical implants161 due to its excellent anti-corrosion properties caused by the spontaneous formation of a 2–3 nm oxide film on the alloy surface.162–164 Nevertheless, these corrosion properties are limited by native oxides that jeopardize the strength of the titanium alloy.165 Benea et al. increased the surface thickness of the Ti-Alloy from 23.06 nm to 123.35 nm by using the anodic oxidation technique for depositing TiO2 nanoparticles, which resulted in safer and better tribo-corrosion than the untreated Ti-Alloy.166 Jin and his coworkers found that TiO2 nanotubes (<100 nm diameter) had excellent osteoblast adhesion properties with elongated nuclei and up-regulated alkaline phosphatase enzyme, which suggests excellent biocompatibility and interaction with bone tissue for orthopedic implants.167
The concept of antibiotic loading on prosthetic implants as a prophylactic therapeutic agent is gaining popularity in biomedical realms. Recently, Chennell et al. used various types of TiO2 nanofibers, nanotubes and smooth surfaces for loading and eluting cefuroxime as an antibiotic on orthopedic implants.168 They found the peak drug release at a two minute time interval from the TiO2 nanotubes with the highest concentration release when compared to other nanostructures. Some studies also revealed successful loading and eluting of gentamycin, a broad spectrum antibiotic, on the surface of TiO2 prosthetic implants and found an inhibitory effect up to six days for Staphylococcus aureus during the in vitro culture sensitivity trial.169 Kumeria et al. and Popat et al. loaded gentamycin on the TiO2 nanotubes array by electrochemically anodizing the compound on the titanium surface to inhibit the Staphylococcus epidermis (Gram positive) bacteria and cause adhesion to osteoblast cells in vitro.170,171 They found excellent osteoblast adhesion differentiation and proliferation, which should be a key feature for any orthopedic prosthetic implant and should keep check on postoperative bacterial infections. TiO2 prosthetic implants for hip replacements were coated with hydroxyapatite and poly(cyclodextrin) to load two different antibiotics, i.e. tobramycin and rifampicin, to control postoperative Staphylococcus aureus and Enterobacter cloacae.172 This dual drug loading synergistic effect on the TiO2 implants was found to be much more efficient at keeping check on infective post-surgical complications. These findings provide new approaches for prophylaxis of prosthetic surgery post-operative infections, which are common complications, by using TiO2 as a drug delivery vehicle and a core prosthetic implant.
Zhao et al. used chitosan hydrogel mesoporous silica nanoparticles on the titanium surface as a pH and electro responsive component to release ibuprofen, i.e. a non-steroidal anti-inflammatory drug (NSAID).173 This approach is amenable to suppress post-operative inflammation. Moreover, the combined antibiotic and NSAID loading on the same TiO2 prosthetic implant can efficiently decrease the postoperative complications of infection and inflammation due to prosthetic surgery.
3.4 Nano-TiO2 for theranostics
The therapeutic effect of TiO2 is solely dependent upon the generation of ROS, including
, O2˙−, OH˙− and 1O2, irrespective of its shape or crystalline form. However, the various isomorphs can affect the extent of the ROS generation due to their reactivity variation, i.e. anatase has been reported to be more reactive than rutile. Successful 1O2 generation has been reported from nano-TiO2 with a size of more than 10 nm by NIR phosphorescence, whereas nano-TiO2 with a size of less than 10 nm is unable to generate 1O2 due to the inability of the smaller size particles to retain charge separation.174 Certain short half-life ROS, i.e.1O2 and OH˙, can interfere with intracellular signal pathways and induce local membrane lipid peroxidation (attributed to weaker diffusion), while the relatively long half-life ROS, i.e. H2O2, O2˙− and
, can strongly diffuse intercellular with a potential to induce apoptosis by interfering with neighbouring cell signal pathways.175
In some studies, the potential of nano-TiO2 as a drug delivery vehicle has also been explored because it is easy to adsorb the therapeutic agent and the presence of an ample number of surface electrons provides scaffolds for covalent or ionic bonding to ensure successful delivery and release in the target tissue via a special microenvironment trigger, i.e. pH, osmotic pressure, blood flow, EPR, etc. The highly reactive uncoordinated surface electrons have a strong affinity to the OH− group in oxygen rich molecules,176 which can be an excellent approach to target specific cell receptors in drug delivery systems.
Recently, our group used TiO2 nanowhiskers to mitigate the adverse effects of TSPP on circulatory and excretory systems, both in vitro and in vivo.177 Porphyrin derivatives’ well-known adverse effects (e.g. neuro-toxicity, photo-toxicity, accumulation in tissue other than the target tissue, etc.) have limited their potential use in biomedical applications. However, TiO2 nanowhiskers’ porous nature and excellent vehicle properties have reintroduced porphyrin derivatives to biomedical realms, especially for neoplastic malady theranostics.
Veerachandra tested various commercially available combinations of TiO2 isomorphs on Escherichia Coli samples for food sanitation and found that Degusa (P25) was more efficient than the other combinations. Moreover, they also observed that the germicidal effect and sanitation properties of P25 were increased with an increase in light intensity.131
Titanate, another form of TiO2 micro- and nano-particles, has gained popularity in recent years. Monosodium titanate (MST) particles are efficient for biomedical applications, especially as a suppressor of human squamous cell carcinoma by holding 30% mitochondrial activity.178 However, non-lethal effects of MST were reported for oral bacterial infections,179 and MST is least effective against murine fibroblasts L929 and human monocytes THP1.180,181 However, Wataha et al. reported the successful delivery of MST metal ions to monocytes and fibroblast cells, and affected the cellular functionality.182 Originally MST was designed for the decontamination of nuclear waste materials as it is a good ion exchanger. However, later on it was found that it can be efficiently bound to biologically active metals.183 Recently Drury et al. demonstrated that nano-MST is more efficient in biological activities than macro-MST.34
Sandoval et al. used europium-III (Eu3+) doped TiO2 nanoshells coated with polyethyleneimine for in vitro cancer cell (HeLa) photo diagnosis.184 This system could provide scaffolds for cancer theranostics if the anticancer agent is coupled with the TiO2 nanoshells.
Nano-TiO2 as a vehicle in a drug delivery system is more sensitive to pH change. Zhang et al. reported that the fabricated TiO2 nanocomposites were pH sensitive and therefore they could selectively deliver DNR to the tumor cells. It is well documented that due to a higher metabolic rate, the pH is lower in neoplastic tissue than in normal tissue (7.4).185
In stem cell biology, nano-TiO2 is also gaining popularity. Recently Lavenus et al. reported the successful tailoring of nano-TiO2 on the surface of glass slides with different angles to investigate its effect on human mesenchymal stem cells. It was observed that a subtle deviation in the coating angle of nano-TiO2 influenced the cell behavior, which was evaluated by cellular pathways viz. differentiation, proliferation, migration and cellular morphology alteration186 (Fig. 7). Similarly, in neural stem cells, the endocytosis and exocytosis of nano-TiO2 irrespective of its shape and size were investigated by Wang et al.. They found nano-TiO2 exocytosis within 72 hours. These findings vouch for the safety of nano-TiO2 and can be used as a landmark for future stem cell investigations.187
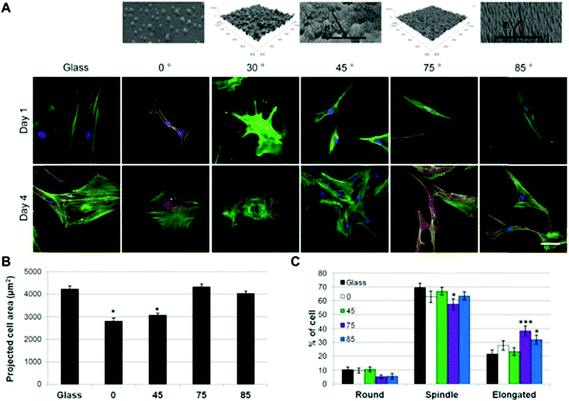 |
| Fig. 7 Cell morphology of hMSCs on the different substrates. A) Staining of the characteristic cell morphology observed on the surface (actin in green, nucleus in blue, scale bar: 25 μm). B) Projected cell area as a function of the surface deposition angle. C) Distribution of the cell morphology on the different substrates (mean ± SEM, *p < 0.05/***p < 0.001 compared to TCPS). Reprinted with permission from ref. 186. Copyright 2015 Elsevier Ltd. | |
3.5 The role of TiO2 in nano-drug delivery systems
A nano-drug delivery system is tailored to be highly specific to achieve the desired concentration and safe drug release in the subject system or tissue. Targeted therapy not only provides scaffolds for safe delivery but also allows combinations of various therapies, e.g. the combination of a photothermal therapeutic agent with a chemotherapeutic agent (doxorubicin, daunorubicin, etc.) or others.99 Currently, gold and silver nanoclusters, MWCNTs, platinum, iridium and titanium are in vogue for nano inorganic drug delivery systems (NI-DDSs) with multiple therapies. Among the various NI-DDSs, titanium has been recently reported as a promising sonodynamic, photodynamic therapeutic and delivery agent for cancer and other infectious diseases146 (Fig. 8).
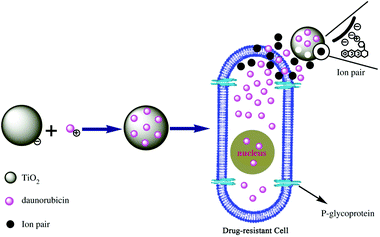 |
| Fig. 8 Chemotherapeutic agent intake by the drug resistant leukemia cells (K562) in the presence of nano-TiO2. Reprinted with permission from ref. 29. Copyright 2006 Elsevier Ltd. | |
Recently, Yin et al. conjugated mesoporous TiO2 up-converting nanoparticles with hyaluronic acid and doxorubicin for cancer therapy.188 These up-converting nanoparticles convert the NIR light to UV light for PDT with mesoporous TiO2 in the tissue and the hyaluronic acid ensures the controlled drug release of the chemotherapeutic agent (doxorubicin) within the neoplastic tissue. Kawashita et al. formed TiO2 microspheres including SiO2 nanoparticles and loaded them with Rhodamine B as a model hydrophilic drug. They found rapid elution of Rhodamine B for the first six hours.189 They exploited TiO2 (anatase/rutile polymorphs) in drug delivery systems, however, for clinical applications, the burst release of the loaded drug may be a problem that needs to be addressed accordingly.190 TiO2 nanoparticles have also been used as a photoprotectant in sunscreens and as a vehicle in drug delivery systems to release terpinen-4-ol during topical applications.
The porous nature of nano-TiO2 has been widely investigated as a vehicle for drug delivery systems. Recently Wang et al. modified porous TiO2 nanoparticles with polyethylineimine to deliver an anticancer drug (paclitaxel) to neoplastic tissue by additionally conjugating with folic acid for site specificity.191 The TiO2 was photoactivated by UV light as a trigger for drug release by producing super oxides and polyethyleneimine was used to prevent premature drug release until its concentration was attained in the desired tumor. The porous TiO2 nanoparticles not only produced the ROS but also released the chemotherapeutic agent upon photoactivation. This property can be efficiently explored for cancer and other malady treatments.
Yuan et al. developed a protocol to ensure the safe delivery of photoactivatable nanoparticles to the cytoplasm of HeLa cancer cells.192 They specially designed Fe3O4@TiO2 nanoparticles to attach to the epidermal growth factor receptor (EGFR), which ensured the safe delivery of the nanocomposites to cellular nucleus without interfering and compromising the EGFR and karyopherin-β interaction, a key protein factor for nucleus translocation. After photoactivation, they found more DNA double strand breakage due to the effect of those photosensitizers, which remained in the cytoplasm.
4. Adverse effects of nano-TiO2
Most nanomaterials that are known to be inert perform totally differently when injected into the body.193 Exposure to nanomaterials may be oral, transdermal, or via inhalation, which exert adverse effects on our various body systems7 and vital organs, including the respiratory system,194,195 gastrointestinal tract,19,196,197 reproductive system,7 excretory system (kidney, liver), circulatory and nervous system,198etc. The length and rigidity of the nanomaterials hamper their clearance from the pulmonary tissue, provoking the release of inflammatory mediators, e.g. multi-walled carbon nanotubes (MWCNT), nano-titania, platinum, etc.44,199
TiO2 nanomaterials are one of the most commonly studied nanomaterials for pulmonary toxicity. Nano-TiO2 was considered as the safest among all the nanomaterials in vogue, until it was reported as a potential carcinogen when murine models were exposed in vivo for prolonged durations200 and then it was ranked as a 2B human potential carcinogen.201,202 It has been reported that chronic and sub-chronic exposure of nano-TiO2 leads to inflammation, epithelial hyperplasia and pulmonary carcinoma in murine models.193 Moreover, TiO2 nanoparticles are considered more toxic than nanowhiskers, similarly larger size TiO2 is reported as being less toxic than smaller sized TiO2.203
4.1 Sexual health
The widespread use of TiO2 nanoparticles has led to severe adverse post-therapeutic complications in animals in general and in advanced primates, including humans in particular.204 Notably, complications have been reported in the reproductive system, such as the decreasing viability and proliferation of laydig cells,205,206 the decreasing follicle survival rate, less griffin follicles and an altered morphology of the follicle and oocyte in murine models.207 When TiO2 nanoparticles were injected into pregnant mice, then their offspring became infertile with abnormal development of seminiferous tubules, decreased sertoli cell numbers and increased spermatozoa primary abnormalities.208,209
TiO2 nanoparticles can cross the blood–testis barrier and damage the testicular interstitial tissue or sertoli cells by up-regulating caspase 3 to induce apoptosis and down-regulating the anti-oxidative enzymes, i.e. Superoxide Dismutase (SOD), Glutathione Peroxidase (GPX), Catalase (CAT), Glutathione S-Transferase (GST), etc., leading to an increase in ROS production.7 Super oxides (O˙−) are formed when oxygen acquires an additional electron. These O˙− are scavenged by the SOD, converting them to H2O2 and −O2.210,211 DNA oxidative damage has also been reported by many studies, suggesting that the smaller sized TiO2 nanoparticles (<20 nm) can cause damage to the DNA double helix along with micronuclei formation and lipid peroxidation of membranes within the cell either in the presence or absence of photoactivation.212,213
4.2 Nervous system
In Central Nervous System (CNS) studies, it has been reported that intravenous nano-TiO2 induces oxidative stress to the brain by increasing the ROS production and decreasing the anti-oxidative enzymes and melatonin level. Moreover, it also activates caspase 3, which initiates apoptosis in the brain tissue.214 TiO2 nanoparticles can translocate in the CNS via olfactory pathways and cause lesions in the brain, including neuronal vacuoles and fatty degeneration of the hippocampus in murine models in vivo.47,52 In recent studies, Younes et al. also reported that intraperitoneal 20 mg per kgbwt of TiO2 nanoparticles on alternate days could alter the neurobehavioral performance and elevate the serum enzymes (ALT, AST, LDH), indicating the additional hepatic malfunction in murine models.50
4.3 Musculoskeletal system
In the musculoskeletal system, the role of nano-TiO2 is very vital because in many orthopedic implants and plates, nano-TiO2 has been used to suppress the autoimmune reactions and inflammatory response in the bone tissues. Nevertheless, there are some studies suggesting the toxic role of TiO2 nanoparticles on the osteoblast cells by generating more ROS, hampering the potential use of TiO2 in prosthetic surgeries. Recently it was reported that nano-TiO2 damages the double helix DNA structure of bone marrow cells post-oral intake and also promotes the proinflammatory genes in the pro-osteoblast cells (MC3T3-E1 cell line).17,215 TiO2 nanoparticles (15 nm) cause impairment of SOD1, SOD2 (Mn SOD) and the sirtuin 3 protein that is present in mitochondria and is responsible for increased catalytic activity and homeostasis in human osteoblast cells (hFOB 1.19). Moreover, the study also revealed ultrastructure alterations in the osteoblast cells216,217 (Fig. 9).
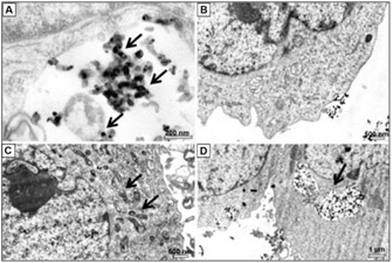 |
| Fig. 9 TiO2 nanoparticles in various organelles of hFOB1.19 cells. Reprinted with permission from ref. 217. Copyright 2015 Dovepress. | |
Cardiac arrhythmias and compromised myocardial function have been reported after administration of TiO2 nanoparticles to rat models.198 Earlier, it was also reported that TiO2 nanoparticles smaller than 100 nm cause endothelial cell leakiness after 60 minutes of exposure, whereas the relatively larger size (more than 600 nm) remained inert.218 This approach can be ideal for studies where cancer amelioration is targeted by normalizing the tumor vasculature;219 however, the consequences can be more drastic on the surrounding healthy tissue if TiO2 accumulates in tissue other than the target tissue.
In another study where TiO2 nanoparticles were used for orthodontic applications, it was found that the nanoparticles accumulated in vacuoles of gingival fibroblast cells without compromising the sensitivity of the human oral squamous cells line (HSC-2) and cytotoxic effects on the surrounding tissue. However, nano-TiO2 increased the prostaglandin E2 production in synergy to IL-1B as a proinflammatory action of the TiO2 nanoparticles.220
5. Conclusions
In summary, from the aforementioned properties of TiO2 nanoparticles and nanowhiskers, it is evident that TiO2 can be an excellent photosensitizer and oxidizing agent for biomedical applications and could be a vital part of nano-biotechnology and photodynamic therapy. The relatively lower toxic effects, the existence in various polymorphs, the economical advantages, the high availability and the excellent biocompatibility towards biological systems declare its potential as a vital component in nanomedicine and biomedical realms. The important role of nano-TiO2 and the relevant nano-biotechnology in early theranostics of neoplastic and non-neoplastic maladies and prosthetic surgeries is exponentially increasing every day. Nevertheless, for the best treatment efficiency, we need to realize the surface functionalization of nano-TiO2 and bring the current photoactivation energy transfer band (400 nm) to the therapeutic window, which is greater than 600 nm excitation wavelength, as approved by the WHO.
Acknowledgements
This work was supported by the National Natural Science Foundation of China (81325011), National High Technology Research & Development Program of China (2015AA020502, 2012AA022703), and the Major Science & Technology Project of Suzhou (ZXY2012028).
Notes and references
- N. L. Rosi and C. A. Mirkin, Chem. Rev., 2005, 105, 1547–1562 CrossRef CAS PubMed.
- D. Narducci, Vet. Res. Commun., 2007, 31, 131–137 CrossRef PubMed.
- T. A. Taton, Trends Biotechnol., 2002, 20, 277–279 CrossRef CAS.
- P. A. Schulte and F. Salamanca-Buentello, Ciênc. Saúde Coletiva, 2007, 12, 1319–1332 Search PubMed.
- E. Katz and I. Willner, Angew. Chem., Int. Ed., 2004, 43, 6042–6108 CrossRef CAS PubMed.
- A. N. Shipway, E. Katz and I. Willner, ChemPhysChem, 2000, 1, 18–52 CrossRef CAS.
- X. Zhao, L. Sheng, L. Wang, J. Hong, X. Yu, X. Sang, Q. Sun, Y. Ze and F. Hong, Part. Fibre Toxicol., 2014, 11, 47 CrossRef PubMed.
- C.-Y. Jin, B.-S. Zhu, X.-F. Wang and Q.-H. Lu, Chem. Res. Toxicol., 2008, 21, 1871–1877 CrossRef CAS PubMed.
- M. Li, S. K. Cushing, Q. Wang, X. Shi, L. A. Hornak, Z. Hong and N. Wu, J. Phys. Chem. Lett., 2011, 2, 2125–2129 CrossRef CAS.
- M. Zhi, A. Koneru, F. Yang, A. Manivannan, J. Li and N. Wu, Proc. Soc. Photo-Opt. Instrum. Eng., 2012, 23, 305501 Search PubMed.
- C. Xiang, F. Yang, M. Li, M. Jaridi and N. Wu, J. Nanopart. Res., 2013, 15, 1–12 Search PubMed.
- R. F. Hamilton, N. Wu, C. Xiang, M. Li, F. Yang, M. Wolfarth, D. W. Porter and A. Holian, Part. Fibre Toxicol., 2014, 11, 43 CrossRef PubMed.
- S. Zhang, D. Yang, D. Jing, H. Liu, L. Liu, Y. Jia, M. Gao, L. Guo and Z. Huo, Nano Res., 2014, 7, 1659–1669 CrossRef CAS.
- H. Shi, R. Magaye, V. Castranova and J. Zhao, Part. Fibre Toxicol., 2013, 10, 15 CrossRef CAS PubMed.
- D. B. Warheit, T. R. Webb, K. L. Reed, S. Frerichs and C. M. Sayes, Toxicology, 2007, 230, 90–104 CrossRef CAS PubMed.
- D. Yang, J. Zhao, H. Liu, Z. Zheng, M. O. Adebajo, H. Wang, X. Liu, H. Zhang, J. c. Zhao and J. Bell, Chem. – Eur. J., 2013, 19, 5113–5119 CrossRef CAS PubMed.
- Y. Zhang, W. Yu, X. Jiang, K. Lv, S. Sun and F. Zhang, J. Mater. Sci.: Mater. Med., 2011, 22, 1933–1945 CrossRef CAS PubMed.
-
D. M. Kahan and D. Rejeski, Project on emerging nanotechnologies, 2009 Search PubMed.
- E. Brun, F. Barreau, G. Veronesi, B. Fayard, S. Sorieul, C. Chanéac, C. Carapito, T. Rabilloud, A. Mabondzo and N. Herlin-Boime, Part. Fibre Toxicol., 2014, 11, 13 CrossRef PubMed.
- A. Weir, P. Westerhoff, L. Fabricius, K. Hristovski and N. von Goetz, Environ. Sci. Technol., 2012, 46, 2242–2250 CrossRef CAS PubMed.
- K. Hashimoto, H. Irie and A. Fujishima, Jpn. J. Appl. Phys., 2005, 44, 8269 CrossRef CAS.
- R. Cai, K. Hashimoto, K. Itoh, Y. Kubota and A. Fujishima, Bull. Chem. Soc. Jpn., 1991, 64, 1268–1273 CrossRef CAS.
- J. E. Ellingsen, Biomaterials, 1991, 12, 593–596 CrossRef CAS.
- J. V. Jokerst and S. S. Gambhir, Acc. Chem. Res., 2011, 44, 1050–1060 CrossRef CAS PubMed.
- J. L. Wang, G. Zhang, Q. W. Li, H. Jiang, C. Y. Liu, C. Amatore and X. M. Wang, Sci. Rep., 2013, 3, 6 Search PubMed.
- A. Z. Wilczewska, K. Niemirowicz, K. H. Markiewicz and H. Car, Pharmacol. Rep., 2012, 64, 1020–1037 CrossRef CAS.
- Y. Matsumura and H. Maeda, Cancer Res., 1986, 46, 6387–6392 CAS.
- O. C. Farokhzad and R. Langer, ACS Nano, 2009, 3, 16–20 CrossRef CAS PubMed.
- M. Song, R. Zhang, Y. Dai, F. Gao, H. Chi, G. Lv, B. Chen and X. Wang, Biomaterials, 2006, 27, 4230–4238 CrossRef CAS PubMed.
- J. Wei, H. Shi, M. Zhou, D. Song, Y. Zhang, X. Pan, J. Zhou and T. Wang, Appl. Catal., A, 2015, 499, 101–108 CrossRef CAS PubMed.
- N. Kinsinger, R. Honda, V. Keene and S. L. Walker, Environ. Eng. Sci., 2015, 32, 292–300 CrossRef CAS.
- E. A. Rozhkova, I. Ulasov, B. Lai, N. M. Dimitrijevic, M. S. Lesniak and T. Rajh, Nano Lett., 2009, 9, 3337–3342 CrossRef CAS PubMed.
- L. Liu, S. Ouyang and J. Ye, Angew. Chem., 2013, 125, 6821–6825 CrossRef PubMed.
- J. L. Drury, Y. Jang, K. M. L. Taylor-Pashow, M. Elvington, D. T. Hobbs and J. C. Wataha, J. Biomed. Mater. Res., Part B, 2015, 103, 254–260 CrossRef PubMed.
- X. Chen, L. Liu, Y. Y. Peter and S. S. Mao, Science, 2011, 331, 746–750 CrossRef CAS PubMed.
- A. Michailowski, D. AlMawlawi, G. Cheng and M. Moskovits, Chem. Phys. Lett., 2001, 349, 1–5 CrossRef CAS.
- D. Li and Y. Xia, Nano Lett., 2004, 4, 933–938 CrossRef CAS.
- P. Hoyer, Langmuir, 1996, 12, 1411–1413 CrossRef CAS.
- I. Gurrappa and L. Binder, Sci. Technol. Adv. Mater., 2008, 9, 043001 CrossRef.
- I. Yashwanth and I. Gurrappa, Mater. Lett., 2015, 142, 328–331 CrossRef CAS PubMed.
-
T. H. Miyauchi Masahiro, in Inorganic and Metallic Nanotubular Materials, Springer, 2010, pp. 45–57 Search PubMed.
- N. Wu, J. Wang, D. N. Tafen, H. Wang, J.-G. Zheng, J. P. Lewis, X. Liu, S. S. Leonard and A. Manivannan, J. Am. Chem. Soc., 2010, 132, 6679–6685 CrossRef CAS PubMed.
- D. Wang, H. Zhao, N. Wu, M. A. El Khakani and D. Ma, J. Phys. Chem. Lett., 2010, 1, 1030–1035 CrossRef CAS.
- R. F. Hamilton jr., Z. Wu, S. Mitra, P. K. Shaw and A. Holian, Part Fibre Toxicol., 2013, 10, 57 CrossRef PubMed.
- M. García-Valverde, R. Lucena, F. Galán-Cano, S. Cárdenas and M. Valcárcel, J. Chromatogr. A, 2014, 1343, 26–32 CrossRef PubMed.
- Q. Li, X. Wang, X. Lu, H. Tian, H. Jiang, G. Lv, D. Guo, C. Wu and B. Chen, Biomaterials, 2009, 30, 4708–4715 CrossRef CAS PubMed.
- J. Wang, G. Zhou, C. Chen, H. Yu, T. Wang, Y. Ma, G. Jia, Y. Gao, B. Li and J. Sun, Toxicol. Lett., 2007, 168, 176–185 CrossRef CAS PubMed.
- E. Fabian, R. Landsiedel, L. Ma-Hock, K. Wiench, W. Wohlleben and B. Van Ravenzwaay, Arch. Toxicol., 2008, 82, 151–157 CrossRef CAS PubMed.
- K. Adachi, N. Yamada, Y. Yoshida and O. Yamamoto, Exp. Dermatol., 2013, 22, 278–283 CrossRef CAS PubMed.
- N. R. B. Younes, S. Amara, I. Mrad, I. Ben-Slama, M. Jeljeli, K. Omri, J. El Ghoul, L. El Mir, K. B. Rhouma and H. Abdelmelek, Environ. Sci. Pollut. Res., 2015, 1–10 Search PubMed.
- L. Ma, J. Liu, N. Li, J. Wang, Y. Duan, J. Yan, H. Liu, H. Wang and F. Hong, Biomaterials, 2010, 31, 99–105 CrossRef CAS PubMed.
- R. Hu, X. Gong, Y. Duan, N. Li, Y. Che, Y. Cui, M. Zhou, C. Liu, H. Wang and F. Hong, Biomaterials, 2010, 31, 8043–8050 CrossRef CAS PubMed.
- J. Wang, Y. Liu, F. Jiao, F. Lao, W. Li, Y. Gu, Y. Li, C. Ge, G. Zhou and B. Li, Toxicology, 2008, 254, 82–90 CrossRef CAS PubMed.
- J. Wang, C. Chen, Y. Liu, F. Jiao, W. Li, F. Lao, Y. Li, B. Li, C. Ge and G. Zhou, Toxicol. Lett., 2008, 183, 72–80 CrossRef CAS PubMed.
- Z. Lu, X. Zhang, Y. Zhao, Y. Xue, T. Zhai, Z. Wu and C. Li, Polym. Chem., 2015, 6, 302–310 RSC.
- C. S. Shemesh, C. W. Hardy, S. Y. David, B. Fernandez and H. Zhang, Photodiagn. Photodyn. Ther., 2014, 11, 193–203 CrossRef CAS PubMed.
- S. P. Singh, M. Sharma, H. Patel and P. K. Gupta, Photodiagn. Photodyn. Ther., 2014, 11, 156–164 CrossRef CAS PubMed.
- T. A. Heinrich, A. C. Tedesco, J. M. Fukuto and R. S. da Silva, Dalton Trans., 2014, 43, 4021–4025 RSC.
-
M. A. Biel, in Biofilm-based Healthcare-associated Infections, Springer, 2015, pp. 119–136 Search PubMed.
- H. S. Qian, H. C. Guo, P. C. L. Ho, R. Mahendran and Y. Zhang, Small, 2009, 5, 2285–2290 CrossRef CAS PubMed.
- R. J. Skyrme, A. J. French, S. N. Datta, R. Allman, M. D. Mason and P. N. Matthews, BJU Int., 2005, 95, 1206–1210 CrossRef CAS PubMed.
- L. E. Rhodes, M. de
Rie, Y. Enström, R. Groves, T. Morken, V. Goulden, G. A. Wong, J.-J. Grob, S. Varma and P. Wolf, Arch. Dermatol., 2004, 140, 17–23 CAS.
- H.-I. Hung, J. M. Schwartz, E. N. Maldonado, J. J. Lemasters and A.-L. Nieminen, J. Biol. Chem., 2013, 288, 677–686 CrossRef CAS PubMed.
- D. Ferguson, A. Perry, M. E. Wood, P. G. Winyard and M. Whiteman, Free Radical Biol. Med., 2014, 76, S135 CrossRef PubMed.
- R. G. Lopes, M. E. S. O. Santi, B. E. Franco, A. M. Deana, R. A. Prates, C. M. França, K. P. S. Fernandes, R. A. M. Ferrari and S. K. Bussadori, Lasers Med. Sci., 2014, 5, 146–152 Search PubMed.
- C. W. Lai, Y. H. Wang, C. H. Lai, M. J. Yang, C. Y. Chen, P. T. Chou, C. S. Chan, Y. Chi, Y. C. Chen and J. K. Hsiao, Small, 2008, 4, 218–224 CrossRef CAS PubMed.
- S.-R. Tsai, R. Yin, Y.-Y. Huang, B.-C. Sheu, S.-C. Lee and M. R. Hamblin, Photodiagn. Photodyn. Ther., 2014, 12, 123–130 CrossRef PubMed.
- M. G. Alvarez, M. N. M. de Oca, M. E. Milanesio, C. S. Ortiz and E. N. Durantini, Photodiagn. Photodyn. Ther., 2014, 11, 148–155 CrossRef CAS PubMed.
- M. Kossakowska, J. Nakonieczna, A. Kawiak, J. Kurlenda, K. P. Bielawski and M. Grinholc, Photodiagn. Photodyn. Ther., 2013, 10, 348–355 CrossRef CAS PubMed.
- H. Guo, H. Qian, N. M. Idris and Y. Zhang, J. Nanomed. Nanotechnol., 2010, 6, 486–495 CrossRef CAS PubMed.
-
M. H. Abdel-Kader, Photodynamic Therapy: From Theory to Application, Springer, Berlin Heidelberg, 2014 Search PubMed.
- S. G. Rockson, D. P. Lorenz, W.-F. Cheong and K. W. Woodburn, Circulation, 2000, 102, 591–596 CrossRef CAS PubMed.
- J. Heckenkamp, T. Luebke, T. Theis, L. Schumacher, M. Gawenda, R. Thul, J. W. Fries and J. Brunkwall, Interact. Cardiovasc. Thorac. Surg., 2012, ivs124 Search PubMed.
-
S. Hossainy, D. Davalian, M. Trollsas, J. Stankus, Y. M. Khong and J. Wan, US Pat., 20100331819 A20100331811, 2012 Search PubMed.
- M. A. Biel, J. W. Jones, L. Pedigo, A. Gibbs and N. Loebel, Laryngoscope, 2012, 122, 2628–2631 CrossRef CAS PubMed.
- J. S. Friedberg, M. J. Culligan, R. Mick, J. Stevenson, S. M. Hahn, D. Sterman, S. Punekar, E. Glatstein and K. Cengel, Ann. Thorac. Surg., 2012, 93, 1658–1667 CrossRef PubMed.
- Y.-M. Jeon, H.-S. Lee, D. Jeong, H.-K. Oh, K.-H. Ra and M.-Y. Lee, Life Sci., 2015, 124, 56–63 CrossRef CAS PubMed.
- M. L. Dimaano, C. Rozario, M. M. Nerandzic, C. J. Donskey, M. Lam and E. D. Baron, Int. J. Mol. Sci., 2015, 16, 7851–7860 CrossRef CAS PubMed.
- F.-Y. Tsai, L.-I. Lau, S.-J. Chen, F.-L. Lee and S.-M. Lee, J. Chin. Med. Assoc., 2014, 77, 52–56 CrossRef CAS PubMed.
- V. F. Carvalho, P. V. Andrade, M. F. Rodrigues, M. H. Hirata, R. D. Hirata, C. M. Pannuti, G. De Micheli and M. C. Conde, J. Clin. Periodontol., 2015, 42, 440–447 CrossRef PubMed.
- I. Roy, T. Y. Ohulchanskyy, H. E. Pudavar, E. J. Bergey, A. R. Oseroff, J. Morgan, T. J. Dougherty and P. N. Prasad, J. Am. Chem. Soc., 2003, 125, 7860–7865 CrossRef CAS PubMed.
- B. S. Zink, L. Valente, B. Ortiz, A. Caldas, T. Jeunon and J. Marques-da-Costa, Photodiagn. Photodyn. Ther., 2013, 10, 535–537 CrossRef PubMed.
- S. Marchal, G. Dolivet, H.-P. Lassalle, F. Guillemin and L. Bezdetnaya, Lasers Med. Sci., 2015, 1–7 Search PubMed.
- J. L. Vivero-Escoto, I. I. Slowing, C.-W. Wu and V. S. Y. Lin, J. Am. Chem. Soc., 2009, 131, 3462–3463 CrossRef CAS PubMed.
- L. Paasonen, T. Laaksonen, C. Johans, M. Yliperttula, K. Kontturi and A. Urth, J. Controlled Release, 2007, 122, 86–93 CrossRef CAS PubMed.
- C. Sudhamani, H. B. Naik, K. S. Gowda, M. Giridhar, D. Girija and P. P. Kumar, Spectrochim. Acta, Part A, 2015, 138, 780–788 CrossRef CAS PubMed.
- A. Yuan, X. Tang, X. Qiu, K. Jiang, J. Wu and Y. Hu, Chem. Commun., 2015, 51, 3340–3342 RSC.
- Y. Cheng, T. L. Doane, C. H. Chuang, A. Ziady and C. Burda, Small, 2014, 10, 1799–1804 CrossRef CAS PubMed.
- M. K. Kuimova, G. Yahioglu and P. R. Ogilby, J. Am. Chem. Soc., 2008, 131, 332–340 CrossRef PubMed.
- K. Piwowar, A. Blacha-Grzechnik and J. Żak, Electrochem. Commun., 2015, 55, 10–13 CrossRef CAS PubMed.
- M. Potasek, E. Parilov and K. Beeson, Proc. SPIE, 2013, 85865 Search PubMed , 85681D-85613.
- D. Daicoviciu, A. Filip, R. M. Ion, S. Clichici, N. Decea and A. Muresan, Folia Biol., 2011, 57, 12–19 CAS.
- M. Pacia, P. Warszyński and W. Macyk, Dalton Trans., 2014, 43, 12480–12485 RSC.
- F. Scholkmann, S. Kleiser, A. J. Metz, R. Zimmermann, J. M. Pavia, U. Wolf and M. Wolf, NeuroImage, 2014, 85, 6–27 CrossRef PubMed.
- Y.-j. Yan, M.-z. Zheng, Z.-l. Chen, X.-h. Yu, X.-x. Yang, Z.-l. Ding and L. Xu, Bioorg. Med. Chem., 2010, 18, 6282–6291 CrossRef CAS PubMed.
- R. R. Allison, G. H. Downie, R. Cuenca, X.-H. Hu, C. J. Childs and C. H. Sibata, Photodiagn. Photodyn. Ther., 2004, 1, 27–42 CrossRef CAS.
- Y. Ye, L.-X. Wang, D.-P. Zhang, Y.-J. Yan and Z.-L. Chen, J. Innovative Opt. Health Sci., 2015, 8 CAS.
- K. Ravindra and G. Zheng, Porphyrins as photosensitizers in photodynamic therapy, 1999, 6, 157–161 Search PubMed.
- D. Chen, C. Zhao, J. Ye, Q. Li, X. Liu, M. Su, H. Jiang, C. Amatore, M. Selke and X. Wang, ACS Appl. Mater. Interfaces, 2015, 7, 18163 CAS.
- L. M. Moreira, F. V. dos Santos, J. P. Lyon, M. Maftoum-Costa, C. Pacheco-Soares and N. S. da Silva, Aust. J. Chem., 2008, 61, 741–754 CrossRef CAS.
- A. B. Ormond and H. S. Freeman, Dyes Pigm., 2013, 96, 440–448 CrossRef CAS PubMed.
- H. Dong, C. Dong, T. Ren, Y. Li and D. Shi, J. Biomed. Nanotechnol., 2015, 10, 2086–2106 CrossRef PubMed.
- Y. Cheng, J. D. Meyers, A.-M. Broome, M. E. Kenney, J. P. Basilion and C. Burda, J. Am. Chem. Soc., 2011, 133, 2583–2591 CrossRef CAS PubMed.
- C. V. Synatschke, T. Nomoto, H. Cabral, M. Förtsch, K. Toh, Y. Matsumoto, K. Miyazaki, A. Hanisch, F. H. Schacher and A. Kishimura, ACS Nano, 2014, 8, 1161–1172 CrossRef CAS PubMed.
- G. I. Harisa and F. K. Alanazi, Saudi Pharm. J., 2014, 22, 504–515 CrossRef PubMed.
- P. Mroz and M. R. Hamblin, Photochem. Photobiol. Sci., 2011, 10, 751–758 CAS.
- J. Wang, J. Ye, H. Jiang, S. Gao, W. Ge, Y. Chen, C. Liu, C. Amatore and X. Wang, RSC Adv., 2014, 4, 37790–37795 RSC.
- H. Jiang, Y. Zhang and X. Wang, Nanoscale, 2014, 6, 10355–10362 RSC.
- Y. Zhang, H. Jiang, W. Ge, Q. Li and X. Wang, Langmuir, 2014, 30, 10910–10917 CrossRef CAS PubMed.
- Y. Zhang, H. Wang, H. Jiang and X. Wang, Nanoscale, 2012, 4, 3530–3535 RSC.
- C. Yang, C. Xu, X. Wang, Z. Xiao and B. Gu, Electrochim. Acta, 2012, 60, 50–54 CrossRef CAS PubMed.
- S. Li, C. Wu, X. Tang, S. Gao, X. Zhao, H. Yan and X. Wang, Sci. China, Ser. B: Chem., 2013, 56, 595–603 CrossRef CAS.
- D. Guo, C. Wu, H. Jiang, Q. Li, X. Wang and B. Chen, J. Photochem. Photobiol., B, 2008, 93, 119–126 CrossRef CAS PubMed.
- D. Chen, S. Gao, W. Ge, Q. Li, H. Jiang and X. Wang, RSC Adv., 2014, 4, 40141–40145 RSC.
- Y. Zhang, X. Bai, X. Wang, K.-K. Shiu, Y. Zhu and H. Jiang, Anal. Chem., 2014, 86, 9459–9465 CrossRef CAS PubMed.
- J. Li, X. Wang, Y. Shao, X. Lu and B. Chen, Materials, 2014, 7, 6865–6878 CrossRef CAS PubMed.
- B.-A. Chen, W.-w. Wu, J. Cheng, J. Wang, F. Gao, W.-l. Xu, C. Gao, J.-h. Ding, Y.-y. Sun and H.-h. Song, Blood, 2010, 116, 1619–1619 Search PubMed.
- L. Wang, H. Zhang, B. Chen, G. Xia, S. Wang, J. Cheng, Z. Shao, C. Gao, W. Bao and L. Tian, Int. J. Nanomedicine, 2012, 7, 789–798 CrossRef CAS.
- L. Zhang, H. Jiang, M. Selke and X. Wang, J. Biomed. Nanotechnol., 2014, 10, 278–286 CrossRef CAS PubMed.
- G. Zhang, C. Wu, H. Ye, H. Yan and X. Wang, J. Nanobiotechnol., 2011, 9, 6 CrossRef CAS PubMed.
- D. Chen, S. Gao, F. U. Rehman, H. Jiang and X. Wang, Sci. China, Ser. B: Chem., 2014, 57, 1532–1537 CrossRef CAS.
- S. Gao, C. Wu, H. Jiang, D. Chen, Q. Li, X. Liu and X. Wang, RSC Adv., 2014, 4, 20841–20846 RSC.
- Y. Wu, X. Zhang, S. Li, X. Lv, Y. Cheng and X. Wang, Electrochim. Acta, 2013, 109, 328–332 CrossRef CAS PubMed.
- W. Ge, Y. Zhang, J. Ye, D. Chen, F. U. Rehman, Q. Li, Y. Chen, H. Jiang and X. Wang, J. Nanobiotechnol., 2015, 13, 8 CrossRef PubMed.
- A. Shimoyama, H. Watase, Y. Liu, S.-I. Ogura, Y. Hagiya, K. Takahashi, K. Inoue, T. Tanaka, Y. Murayama and E. Otsuji, Photodiagn. Photodyn. Ther., 2013, 10, 607–614 CrossRef CAS PubMed.
- Y. Li, T.-y. Lin, Y. Luo, Q. Liu, W. Xiao, W. Guo, D. Lac, H. Zhang, C. Feng and S. Wachsmann-Hogiu, Nat. Commun., 2014, 5, 4712 CrossRef CAS PubMed.
- L. Zeng, W. Ren, L. Xiang, J. Zheng, B. Chen and A. Wu, Nanoscale, 2013, 5, 2107–2113 RSC.
- H. E. Townley, J. Kim and P. J. Dobson, Nanoscale, 2012, 4, 5043–5050 RSC.
- J. Li, X. Wang, H. Jiang, X. Lu, Y. Zhu and B. Chen, Nanoscale, 2011, 3, 3115–3122 RSC.
- M. Montazer, A. Behzadnia, E. Pakdel, M. K. Rahimi and M. B. Moghadam, J. Photochem. Photobiol., B, 2011, 103, 207–214 CrossRef CAS PubMed.
- V. K. Yemmireddy and Y.-C. Hung, LWT–Food Sci. Technol., 2015, 61, 1–6 CrossRef CAS PubMed.
- X. Chen and S. S. Mao, Chem. Rev., 2007, 107, 2891–2959 CrossRef CAS PubMed.
- Z. Hou, Y. Zhang, K. Deng, Y. Cheng, X. Li, X. Deng, Z. Cheng, H. Lian, C. Li and J. Lin, ACS Nano, 2015, 9, 2584–2599 CrossRef CAS PubMed.
- Y. Tokuoka, M. Yamada, N. Kawashima and T. Miyasaka, Chem. Lett., 2006, 35, 496–497 CrossRef CAS.
- C. Zhao, F. U. Rehman, Y. Yang, X. Li, D. Zhang, H. Jiang, M. Selke, X. Wang and C. Liu, Sci. Rep., 2015, 5, 11518 CrossRef PubMed.
- N. Yumita, R. Nishigaki, K. Umemura and S. I. Umemura, Cancer Sci., 1989, 80, 219–222 CrossRef CAS PubMed.
- T. Ohmura, T. Fukushima, H. Shibaguchi, S. Yoshizawa, T. Inoue, M. Kuroki, K. Sasaki and S.-I. Umemura, Anticancer Res., 2011, 31, 2527–2533 CAS.
- P. Wang, L. Xiao, X. Wang, X. Li and Q. Liu, Ultrasonics, 2010, 50, 634–638 CrossRef CAS PubMed.
- N. Yumita, Y. Iwase, K. Nishi, H. Komatsu, K. Takeda, K. Onodera, T. Fukai, T. Ikeda, S.-i. Umemura and K. Okudaira, Theranostics, 2012, 2, 880 CrossRef CAS PubMed.
- Y. Harada, K. Ogawa, Y. Irie, H. Endo, L. B. Feril, T. Uemura and K. Tachibana, J. Controlled Release, 2011, 149, 190–195 CrossRef CAS PubMed.
- S. Shen, L. Wu, J. Liu, M. Xie, H. Shen, X. Qi, Y. Yan, Y. Ge and Y. Jin, Int. J. Pharm., 2015, 486, 380–388 CrossRef CAS PubMed.
- S. Shen, B. Ding, L. Wu, X. Y. Qi and Y. R. Ge, Key Eng. Mater., 2014, 636, 129–132 CrossRef.
- S. Shen, X. Guo, L. Wu, M. Wang, X. Wang, F. Kong, H. Shen, M. Xie, Y. Ge and Y. Jin, J. Mater. Chem. B, 2014, 2, 5775–5784 RSC.
- S. Yamaguchi, H. Kobayashi, T. Narita, K. Kanehira, S. Sonezaki, N. Kudo, Y. Kubota, S. Terasaka and K. Houkin, Ultrason. Sonochem., 2011, 18, 1197–1204 CrossRef CAS PubMed.
- K. Ninomiya, K. Noda, C. Ogino, S.-i. Kuroda and N. Shimizu, Ultrason. Sonochem., 2014, 21, 289–294 CrossRef CAS PubMed.
- K. Ninomiya, C. Ogino, S. Oshima, S. Sonoke, S.-i. Kuroda and N. Shimizu, Ultrason. Sonochem., 2012, 19, 607–614 CrossRef CAS PubMed.
- A. Harada, M. Ono, E. Yuba and K. Kono, Biomater. Sci., 2013, 1, 65–73 RSC.
- B. Feng, J. Weng, B. Yang, S. Qu and X. Zhang, Biomaterials, 2003, 24, 4663–4670 CrossRef CAS.
- Y.-T. Sul, Int. J. Nanomed., 2010, 5, 87 CrossRef CAS.
- A. Patri, T. Umbreit, J. Zheng, K. Nagashima, P. Goering, S. Francke-Carroll, E. Gordon, J. Weaver, T. Miller and N. Sadrieh, J. Appl. Toxicol., 2009, 29, 662–672 CrossRef CAS PubMed.
- S. Jain, A. P. Jain, S. Jain, O. N. Gupta and A. Vaidya, J. Dent. Sci., 2013 DOI:10.1016/j.jds.2013.08.004.
- A. Tautzenberger, A. Kovtun and A. Ignatius, Int. J. Nanomed., 2012, 7, 4545 CrossRef CAS PubMed.
- S.-H. Oh, R. R. Finones, C. Daraio, L.-H. Chen and S. Jin, Biomaterials, 2005, 26, 4938–4943 CrossRef CAS PubMed.
- S. Oh, C. Daraio, L. H. Chen, T. R. Pisanic, R. R. Finones and S. Jin, J. Biomed. Mater. Res., Part A, 2006, 78, 97–103 CrossRef PubMed.
- L. M. Bjursten, L. Rasmusson, S. Oh, G. C. Smith, K. S. Brammer and S. Jin, J. Biomed. Mater. Res., Part A, 2010, 92, 1218–1224 Search PubMed.
- M. J. Santillan, N. E. Quaranta and A. R. Boccaccini, Surf. Coat. Technol., 2010, 205, 2562–2571 CrossRef CAS PubMed.
- H. S. Kim, Y. Z. Yang, J. T. Koh, K. K. Lee, D. J. Lee, K. M. Lee and S. W. Park, J. Biomed. Mater. Res., Part B, 2009, 88, 427–435 CrossRef PubMed.
- J. Lu and T. J. Webster, Acta Biomater., 2015, 16, 223–231 CrossRef CAS PubMed.
- A. Palmquist, O. M. Omar, M. Esposito, J. Lausmaa and P. Thomsen, J. R. Soc., Interface, 2010, 5, S515–S527 CrossRef PubMed.
- A. Biesiekierski, J. Wang, M. A.-H. Gepreel and C. Wen, Acta Biomater., 2012, 8, 1661–1669 CrossRef CAS PubMed.
- L. Mohan, C. Anandan and N. Rajendran, Electrochim. Acta, 2015, 155, 411–420 CrossRef CAS PubMed.
- C. E. Marino, E. M. de Oliveira, R. C. Rocha-Filho and S. R. Biaggio, Corros. Sci., 2001, 43, 1465–1476 CrossRef CAS.
- C. Elias, J. Lima, R. Valiev and M. Meyers, JOM, 2008, 60, 46–49 CrossRef CAS.
- V. Alves, R. Reis, I. Santos, D. Souza, T. d. F. Gonçalves, M. Pereira-da-Silva, A. Rossi and L. Da Silva, Corros. Sci., 2009, 51, 2473–2482 CrossRef CAS PubMed.
- M. Sarraf, E. Zalnezhad, A. Bushroa, A. Hamouda, A. Rafieerad and B. Nasiri-Tabrizi, Ceram. Int., 2015, 41, 7952–7962 CrossRef CAS PubMed.
- L. Benea, E. Danaila and P. Ponthiaux, Corros. Sci., 2015, 91, 262–271 CrossRef CAS PubMed.
- K. S. Brammer, S. Oh, C. J. Cobb, L. M. Bjursten, H. van der Heyde and S. Jin, Acta Biomater., 2009, 5, 3215–3223 CrossRef CAS PubMed.
- P. Chennell, E. Feschet-Chassot, T. Devers, K. Awitor, S. Descamps and V. Sautou, Int. J. Pharm., 2013, 455, 298–305 CrossRef CAS PubMed.
- Van Den Berghe, E. Courcot, J. Sobocinski, N. Tabary, F. Chai, J.-F. Blach and A. Addad, ACS Appl. Mater. Interfaces, 2015, 7, 12882–12893 Search PubMed.
- T. Kumeria, H. Mon, M. S. Aw, K. Gulati, A. Santos, H. J. Griesser and D. Losic, Colloids Surf., B, 2015, 130, 255–263 CrossRef CAS PubMed.
- K. C. Popat, M. Eltgroth, T. J. LaTempa, C. A. Grimes and T. A. Desai, Biomaterials, 2007, 28, 4880–4888 CrossRef CAS PubMed.
- M. Taha, F. Chai, N. Blanchemain, C. Neut, M. Goube, M. Maton, B. Martel and H. F. Hildebrand, Int. J. Pharm., 2014, 477, 380–389 CrossRef CAS PubMed.
- P. Zhao, H. Liu, H. Deng, L. Xiao, C. Qin, Y. Du and X. Shi, Colloids Surf., B, 2014, 123, 657–663 CrossRef CAS PubMed.
- T. Daimon and Y. Nosaka, J. Phys. Chem. C, 2007, 111, 4420–4424 CAS.
- P. C. Panus, R. Radi, P. H. Chumley, R. H. Lillard and B. A. Freeman, Free Radical Biol. Med., 1993, 14, 217–223 CrossRef CAS.
- T. Rajh, L. Chen, K. Lukas, T. Liu, M. Thurnauer and D. Tiede, J. Phys. Chem. B, 2002, 106, 10543–10552 CrossRef CAS.
- F. U. Rehman, C. Zhao, H. Jiang, M. Selke and X. Wang, Photodiagn. Photodyn. Ther., 2015 DOI:10.1016/j.pdpdt.2015.08.005.
- J. L. Drury, Y.-W. Chen, J. J. Wong, M. C. Elvington, R. B. Rutherford, D. T. Hobbs and J. C. Wataha, J. Exp. Clin. Med., 2014, 6, 21–27 CrossRef CAS PubMed.
- W. O. Chung, J. C. Wataha, D. T. Hobbs, J. An, J. J. Wong, C. H. Park, S. Dogan, M. C. Elvington and R. B. Rutherford, J. Biomed. Mater. Res., Part A, 2011, 97, 348–354 CrossRef PubMed.
- R. Davis, P. Lockwood, D. Hobbs, R. Messer, R. Price, J. Lewis and J. Wataha, J. Biomed. Mater. Res., Part B, 2007, 83, 505–511 CrossRef CAS PubMed.
- R. R. Davis, D. T. Hobbs, R. Khashaba, P. Sehkar, F. N. Seta, R. L. Messer, J. B. Lewis and J. C. Wataha, J. Biomed. Mater. Res., Part A, 2010, 93, 864–869 Search PubMed.
- J. C. Wataha, D. T. Hobbs, J. J. Wong, S. Dogan, H. Zhang, K. H. Chung and M. C. Elvington, J. Mater. Sci.: Mater. Med., 2010, 21, 1289–1295 CrossRef CAS PubMed.
- D. Hobbs, R. Messer, J. Lewis, D. Click, P. Lockwood and J. C. Wataha, J. Biomed. Mater. Res., Part B, 2006, 78, 296–301 CrossRef CAS PubMed.
- S. Sandoval, J. Yang, J. G. Alfaro, A. Liberman, M. Makale, C. E. Chiang, I. K. Schuller, A. C. Kummel and W. C. Trogler, Chem. Mater., 2012, 24, 4222–4230 CrossRef CAS PubMed.
- H. Zhang, C. Wang, B. Chen and X. Wang, Int. J. Nanomed., 2012, 7, 235 CAS.
- S. Lavenus, D. J. Poxson, N. Ogievetsky, J. S. Dordick and R. W. Siegel, Biomaterials, 2015, 55, 96–109 CrossRef CAS PubMed.
- Y. Wang, Q. Wu, K. Sui, X.-X. Chen, J. Fang, X. Hu, M. Wu and Y. Liu, Nanoscale, 2013, 5, 4737–4743 RSC.
- M. Yin, E. Ju, Z. Chen, Z. Li, J. Ren and X. Qu, Chem. – Eur. J., 2014, 20, 14012–14017 CrossRef CAS PubMed.
- E. B. Manaia, R. C. K. Kaminski, A. G. de Oliveira, M. A. Corrêa and L. A. Chiavacci, Int. J. Nanomed., 2015, 10, 811 CAS.
- M. Kawashita, Y. Tanaka, S. Ueno, G. Liu, Z. Li and T. Miyazaki, Mater. Sci. Eng., C, 2015, 50, 317–323 CrossRef CAS PubMed.
- T. Wang, H. Jiang, L. Wan, Q. Zhao, T. Jiang, B. Wang and S. Wang, Acta Biomater., 2015, 13, 354–363 CrossRef CAS PubMed.
- Y. Yuan, S. Chen, T. Paunesku, S. C. Gleber, W. C. Liu, C. B. Doty, R. Mak, J. Deng, Q. Jin and B. Lai, ACS Nano, 2013, 7, 10502–10517 CrossRef CAS PubMed.
- G. Oberdörster, E. Oberdörster and J. Oberdörster, Environ. Health Perspect., 2005, 113, 823–839 CrossRef.
- B. L. Baisch, N. M. Corson, P. Wade-Mercer, R. Gelein, A. J. Kennell, G. Oberdorster and A. Elder, Part. Fibre Toxicol., 2014, 11, 5 CrossRef PubMed.
- W. McKinney, M. Jackson, T. Sager, J. Reynolds, B. Chen, A. Afshari, K. Krajnak, S. Waugh, C. Johnson and R. Mercer, Inhalation Toxicol., 2012, 24, 447–457 CrossRef CAS PubMed.
- C. M. Nogueira, W. M. de Azevedo, M. L. Z. Dagli, S. H. Toma, A. Z. de Arruda Leite, M. L. Lordello, I. Nishitokukado, C. L. Ortiz-Agostinho, M. I. S. Duarte and M. A. Ferreira, World J. Gastroenterol., 2012, 18, 4729 CrossRef CAS PubMed.
- E. Fröhlich, B. J. Teubl and E. Roblegg, BioNanoMaterials, 2013, 14, 25–35 CrossRef.
- M. Savi, S. Rossi, L. Bocchi, L. Gennaccaro, F. Cacciani, A. Perotti, D. Amidani, R. Alinovi, M. Goldoni and I. Aliatis, Part. Fibre Toxicol., 2014, 11, 63 CrossRef PubMed.
- L. M. Sargent, D. W. Porter, L. M. Staska, A. F. Hubbs, D. T. Lowry, L. Battelli, K. J. Siegrist, M. L. Kashon, R. R. Mercer and A. K. Bauer, Part. Fibre Toxicol., 2014, 11, 3 CrossRef PubMed.
- K. Lee, H. Trochimowicz and C. Reinhardt, Toxicol. Appl. Pharmacol., 1985, 79, 179–192 CrossRef CAS.
- I. A. f. R. o. Cancer, 2006.
- A. D. Maynard, Nature, 2011, 475, 31–31 CrossRef CAS PubMed.
- J. Zhao, L. Bowman, X. Zhang, V. Vallyathan, S.-H. Young, V. Castranova and M. Ding, J. Toxicol. Environ. Health, Part A, 2009, 72, 1141–1149 CrossRef CAS PubMed.
- G. Oberdörster, V. Stone and K. Donaldson, Nanotoxicology, 2007, 1, 2–25 CrossRef PubMed.
- Z. Lan and W.-X. Yang, Nanomedicine, 2012, 7, 579–596 CrossRef CAS PubMed.
- T. Komatsu, M. Tabata, M. Kubo-Irie, T. Shimizu, K.-i. Suzuki, Y. Nihei and K. Takeda, Toxicol. in Vitro, 2008, 22, 1825–1831 CrossRef CAS PubMed.
- H. Juan, W. XuYing, W. Fei, X. GuiFeng, L. Zhen and Z. TianBao, Ti-erh Chun I Ta Hsueh Hsueh Pao, 2009, 30, 869–873 Search PubMed.
- M. Ema, N. Kobayashi, M. Naya, S. Hanai and J. Nakanishi, Adv. Exp. Med. Biol., 2010, 30, 343–352 CAS.
- K. Takeda, K.-i. Suzuki, A. Ishihara, M. Kubo-Irie, R. Fujimoto, M. Tabata, S. Oshio, Y. Nihei, T. Ihara and M. Sugamata, J Health Sci., 2009, 55, 95–102 CrossRef CAS.
- K. Bhattacharya, G. Alink and E. Dopp, Int. J. Hum. Genet., 2007, 7, 1 CAS.
- P. I. Merksamer, Y. Liu, W. He, M. D. Hirschey, D. Chen and E. Verdin, Aging, 2013, 5, 144 CAS.
- J.-R. Gurr, A. S. Wang, C.-H. Chen and K.-Y. Jan, Toxicology, 2005, 213, 66–73 CrossRef CAS PubMed.
- B. Trouiller, R. Reliene, A. Westbrook, P. Solaimani and R. H. Schiestl, Cancer Res., 2009, 69, 8784–8789 CrossRef CAS PubMed.
- R. Meena, S. Kumar and R. Paulraj, J. Nanopart. Res., 2015, 17, 1–14 CrossRef CAS.
- Z. Chen, Y. Wang, T. Ba, Y. Li, J. Pu, T. Chen, Y. Song, Y. Gu, Q. Qian and J. Yang, Toxicol. Lett., 2014, 226, 314–319 CrossRef CAS PubMed.
- R. Nogueiras, K. M. Habegger, N. Chaudhary, B. Finan, A. S. Banks, M. O. Dietrich, T. L. Horvath, D. A. Sinclair, P. T. Pfluger and M. H. Tschöp, Physiol. Rev., 2012, 92, 1479–1514 CrossRef CAS PubMed.
- K. Niska, K. Pyszka, C. Tukaj, M. Wozniak, M. W. Radomski and I. Inkielewicz-Stepniak, Int. J. Nanomed., 2015, 10, 1095 CAS.
- M. Setyawati, C. Y. Tay, S. Chia, S. Goh, W. Fang, M. Neo, H. C. Chong, S. Tan, S. C. J. Loo and K. Ng, Nat. Commun., 2013, 4, 1673 CrossRef CAS PubMed.
- R.-L. Huang, Z. Teo, H. C. Chong, P. Zhu, M. J. Tan, C. K. Tan, C. R. I. Lam, M. K. Sng, D. T. W. Leong and S. M. Tan, Blood, 2011, 118, 3990–4002 CrossRef CAS PubMed.
- R. Garcia-Contreras, M. Sugimoto, N. Umemura, M. Kaneko, Y. Hatakeyama, T. Soga, M. Tomita, R. J. Scougall-Vilchis, R. Contreras-Bulnes and H. Nakajima, Biomaterials, 2015, 57, 33–40 CrossRef CAS PubMed.
|
This journal is © The Royal Society of Chemistry 2016 |