DOI:
10.1039/C5BM00163C
(Review Article)
Biomater. Sci., 2016,
4, 25-39
Composite biopolymers for bone regeneration enhancement in bony defects
Received
28th May 2015
, Accepted 11th August 2015
First published on 28th August 2015
Abstract
For the past century, various biomaterials have been used in the treatment of bone defects and fractures. Their role as potential substitutes for human bone grafts increases as donors become scarce. Metals, ceramics and polymers are all materials that confer different advantages to bone scaffold development. For instance, biocompatibility is a highly desirable property for which naturally-derived polymers are renowned. While generally applied separately, the use of biomaterials, in particular natural polymers, is likely to change, as biomaterial research moves towards mixing different types of materials in order to maximize their individual strengths. This review focuses on osteoconductive biocomposite scaffolds which are constructed around natural polymers and their performance at the in vitro/in vivo stages and in clinical trials.
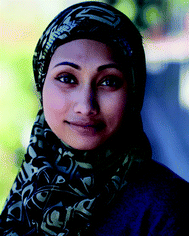 K. Jahan | Kaushar Jahan completed her Bachelor's degree in Anatomy and Cellular Biology at McGill University. During her undergraduate studies she acquired extensive skills in molecular and cell biology. She then started her Master's degree exploring 2D scaffolds for bone regeneration with Dr Maryam Tabrizian and fast-tracked into the PhD program to further develop an injectable 3D scaffold for bone repair in critical-sized defect models. |
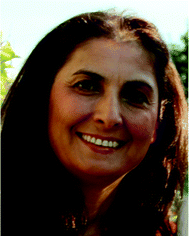 M. Tabrizian | Maryam Tabrizian is full professor in the Biological and Biomedical Engineering Department at McGill University. She is a Guggenheim Fellow in Biomedical Sciences and a Fellow of the Biomaterials Science & Engineering. She has established expertise in designing advanced biomaterials and bioactive biointerface for application in nanomedicine, regenerative medicine and Lab-on-a-chip devices. |
Introduction
Non-union or mal-union fractures resulting in bone defects remain a big challenge in the field of bone tissue engineering. A bone defect is defined as the smallest size defect that will not repair without medical intervention.1 Depending on the bone involved, the “critical size” of a non-union defect may vary: forearm (3 cm), femur and tibia (5 cm) and humerus (6 cm).2 Autologous bone grafts are currently the gold standard in bone repair, followed by allografts (from another individual) and xenografts (from an animal). However, donor shortages and donor site morbidity are amongst these treatments’ several shortcomings.3 This has led to ample research on alternative bone graft materials such as polymers, metals and ceramics. Their role is not only to fill the bone fissure but also to provide both structural and mechanical support. An increasing number of natural and synthetic graft materials are under development with composites being favoured as they combine all the advantageous properties of the individual monolithic biomaterials. An investigation of the literature has shown that even though synthetic polymers have been widely used in bone research over the past ten years, the same cannot be said about naturally-derived polymers (Fig. 1). While the use of synthetic polymers has increased substantially over time, the use of naturally-derived polymers has remained steady, despite their abundance in nature and their biocompatibility. In this review, we aim to cover naturally-derived polymer-based biocomposites reported in the past 10 years and to present their potential as a material of choice for bone.
 |
| Fig. 1 Graph displaying the total number of publications on polymers used in bone tissue engineering vs. naturally-derived polymers used in the same field. Source: Web of Science, 2015. | |
Biology of bone
Bone composition
Bone is a specialized connective tissue made of calcified matter called the bone matrix, and three dominant types of cells. Osteoblasts are the cells that synthesize and release the organic material of bone. Osteocytes, cells deriving from mature osteoblasts, are found within calcified bone in cavities called lacunae. Lastly, osteoclasts are large multinucleated cells with projections that play a key role in bone resorption to maintain bone homeostasis. The bone matrix is composed of both organic and inorganic material. The inorganic material includes calcium hydroxyapatite, bicarbonate, potassium and magnesium ions among others. The organic part is made of proteins such as type I collagen, as well as proteoglycans and glycoproteins. The organic components are embedded within the calcified matrix. Anatomically, bone tissue has two distinct structures: compact (80%) and cancellous (20%) bones. Compact bone is a denser structure found at the surface whereas cancellous bone is a cavity-filled structure found in the deeper areas of the bone. For a more extensive review of bone composition, please refer to Junqueira's Basic Histology.4
Bone formation
Bone development starts in the first few weeks of embryogenesis through two stages: osteogenesis which is the bone formation, and ossification which is the mineralization process.6,7 Two types of ossification take place via the condensation of mesenchymal stem cells (MSCs).8 The first is an intra-membranous ossification for the development of flat bones, and the second is an endochondral ossification (Fig. 2) for other types of bone.5 During intra-membranous ossification the MSCs will differentiate into pre-osteoblasts and osteoblasts, which will in turn produce extracellular matrix proteins that become mineralized.9 These bone deposits, also known as spicules, fuse together to form a trabecular bone. This will form a periosteum on the outer surface. Mature trabeculae will replace the immature trabeculae during the secondary bone formation.10 The endochondral ossification forms through a structure called the cartilage template.
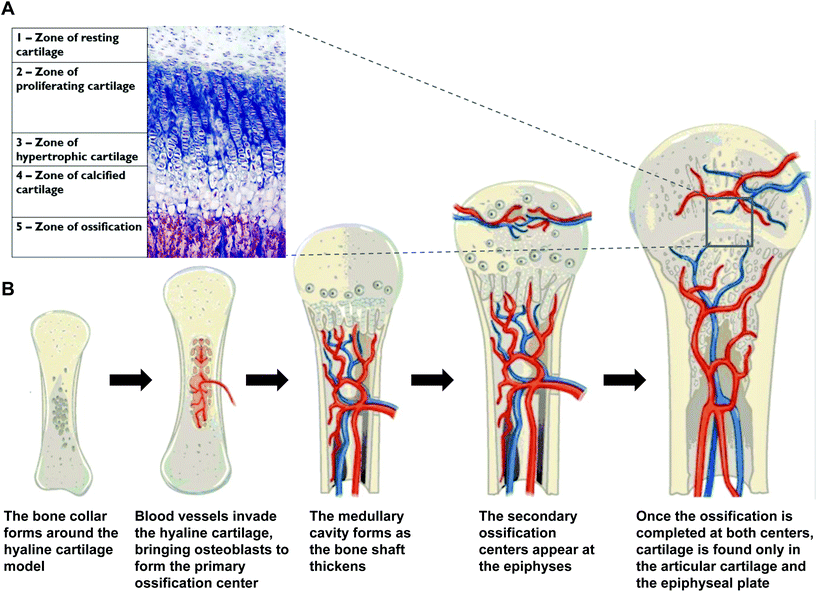 |
| Fig. 2 (A) A histological stained image of a growth plate.4 (B) The different stages of endochondral ossification as it occurs in long bones (adapted from Biology-OpenStax College, download for free at http://cnx.org/contents/185cbf87-c72e-48f5-b51e-f14f21b5eabd@9.83).5 | |
MSCs condense and differentiate into chondrocytes. The latter will deposit hyaline cartilage that will act as a template for bone formation. This cartilage will undergo interstitial growth to lengthen and appositional growth to widen. The matrix will begin to calcify and large chondrocytes amidst the matrix will die, leaving holes. This progression is regulated via several signaling pathways such as Hedgehog, bone morphogenic proteins (BMPs), transforming growth factor beta (TGF-β), Wnt and parathyroid hormone (PTH).7 Meanwhile blood vessels start growing closer to the cartilage, and osteoblast differentiation is induced within the outer layer of the hyaline cartilage, the perichondrium. The osteoblasts create a bone collar that grows to become compact bone. At this point, the perichondrium is referred to as the periosteum.11,12 The blood vessels now invade the cartilage in the central region and introduce mesenchymal and hematopoietic stem cells. Both these cells differentiate into osteoblasts which form the trabecular bone; this is the primary ossification center. The cartilage gets replaced by the bone along the shaft. This is followed by the formation of the marrow cavity via bone remodelling. The blood vessels bring in monocytes that will differentiate into osteoclasts, which are bone resorbing cells.9 The marrow cavity is enlarged by the resorption of the trabecular bone and is eventually replaced by red bone marrow. The diaphysis elongates while the cortical bone thickens. The bone also replaces the cartilage at the metaphysis. Next, the blood vessels invade the epiphyses at each end of the bone and introduce osteoblasts. Ossification ensues just like in the primary ossification center, except that no medullary cavity is formed this time. The cartilage at the epiphyses is mostly replaced by the trabecular bone except at two distinct locations: (i) articular cartilage covering the bone at the joint and (ii) the growth plate that separates the epiphysis from the diaphysis.13,14
Bone regeneration
Fracture healing involves both types of ossification. Immediately following a fracture, there is a haematoma formation due to the torn blood vessels. A mass of clotted blood will rise at the fracture site while bone cells will die. The surrounding fibroblasts survive and start dividing. Platelets then release platelet-derived growth factors (PDGF) TGF-β causing local inflammation and swelling.15 Other secreted cytokines such as tumor necrosis factor-alpha (TNF-α), and interleukin-1, -6, -11 and -18 (IL-1, -6, -11 and -18) will lead to the recruitment of inflammatory cells which will in turn recruit MSCs at the site of injury.16 Receptors TNFR1 and TNFR2 are activated with TNFR2 being specific to bone repair. This leads to the formation of a soft callus. The MSCs then divide and differentiate into osteogenic cells. Intra-membranous ossification takes place at the edge of the fracture where there is sufficient oxygen, whereas endochondral ossification occurs close to the fracture where the environment is hypoxic.17 A fibrocartilaginous callus forms to stabilize the bone injury. An external callus consisting of hyaline cartilage surrounds the fracture while an internal callus made of cartilage and collagen forms in the bone marrow cavity. Revascularization follows and phagocytic cells clean up the debris. The bony callus starts forming when new spongy bone trabeculae appear in the fibrocartilaginous callus. Mineralization of the matrix begins 3–4 weeks after the injury and extends up to 2–3 months, until union of the fracture occurs. However, in order for the bone to restore its biomechanical properties, the excess material needs to be removed through a remodelling process. The exterior of the bone and the interior of the medullary canal are resorbed by the osteoclasts for up to a year in some cases.15 And in the occurrence of an open fracture or bone excision due to a tumor, it is very difficult for the bone to repair itself without any external aid. This results in impaired chondrogenesis due to insufficient blood supply and the inability to maintain mechanical stability. The development of an atrophic fibrous non-union or a cartilaginous callus with a hypertrophic non-union can be seen as the healing processes take place.17
Critical-sized defects
Although the bone is a self-healing tissue, in many cases when the bone loss exceeds the critical size, and the osteogenesis is compromised. This is due to many factors like the reduced expression of certain factors involved chondrocyte maturation and osteoblasts differentiation such as COX-2, BMP2 and various Wnts. Unsuccessful bone repair may also be due to impaired revascularization in gaps that are too large, thus not providing enough nutrients and oxygen.15 Currently, in the literature, the term “critical-sized defect” (CSD) generally refers to an animal model with an introduced bony defect that is most suitable for studying bone repair mechanisms.18 The bone defect must affect both cancellous and cortical bone, be stable and minimize fracture.19 The size of the induced defect in a rat must be between 6–8 mm.1,18 The defect is defined as “the smallest size intraosseous wound in a particular bone and species of animal that will not heal spontaneously during the lifetime of the animal”.20 According to Cacchioli et al., the CSD healing is usually evaluated by both the quantity and the quality of the newly regenerated bone; the best tissue quality being formed in a relatively short time.16 The evaluation of the newly formed bone relies on several imaging and immunocytochemistry techniques, such as microcomputed tomography, radiography, histology, and immunocytochemistry, among others.21–24
Currently the rodent model is the most studied with its well-established calvarial, mandibular and long bone models (Fig. 3).1 Rats make excellent models for CSD with 8 mm defects in the calvaria, 4 mm in the mandible and 12 mm in the long bones. Rabbit models have a 5–20 mm calvarial defect, 5 mm mandible defect and 6 mm long bone defect. Cats’ long bones do not heal at 1.5 times the diameter of the shaft. Dogs’ calvaria has a 20 mm gap, their mandibles have a 3 cm defect and their long bones have a defect 2 times the shaft diameter. Pigs were also used in some studies with femoral defects of 6–12 cm. Horses and guinea pigs were also used as CSD models but to a lesser extent. Among non-human primates, baboons were tested with a 15 mm calvarial defect and rhesus monkeys with a 4 cm mandible defect.25 Numerous bone repair approaches are currently under study; however, they necessitate intensive investigation before they can be applied to clinical trials. Such an approach makes use of biomaterial constructs, which are progressively gaining interest as state-of-the-art bone graft materials.
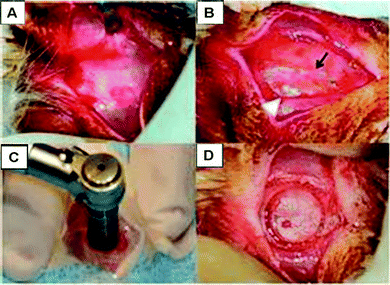 |
| Fig. 3 Creation of the defect in a rodent. Removal of (A) skin and (B) periosteum. (C) A trephine is used to cut the calvarial bone resulting in a (D) scored calvarium (adapted from Spicer et al., 2012).1 | |
Ideal bone regeneration according to the “diamond concept”
Bone repair research currently focuses on five general therapeutic targets: osteogenesis, vascularization, growth factors, mechanical environment and osteoconductive scaffolds (Fig. 4). However, using each element on its own does not always yield consistent results. This calls for a more efficient approach that tackles numerous aspects of bone repair. The “diamond concept” established by Giannoudis et al.26,27 states that the five major elements for successful bone regeneration should ideally be studied in a combination of at least three. This strategy, termed “polytherapy”, usually focuses on investigating the osteogenic cells, the growth factors and the scaffolds, with the assumption that the mechanical environment is provided and that angiogenesis will ensue.28 The cell types that have shown most osteogenic potential for implantation in bone defects are MSCs, embryonic stem cells (ESCs), induced pluripotent stem cells (iPSCs), adipose derived stem cells (ADSCs) and exfoliated deciduous teeth stem cells (SHED). These cells, which can progress from progenitors to fully matured bone cells, are employed at different stages of bone repair.29
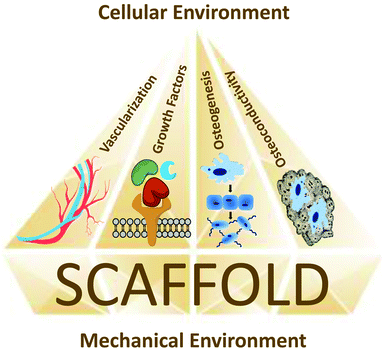 |
| Fig. 4 Diamond concept for the design of scaffolds in bone regeneration. | |
The cellular models of choice to investigate cytocompatibility are usually MSCs, pre-osteoblasts (MC3T3), and fibroblasts (NIH3T3), among many others. This multifaceted approach can be applied to both animal and human bone defect models. Ideally, a bone substitute should have properties adapted to its specific clinical situation. Properties such as geometrical form, internal structure (pore size and distribution, crystallinity), surface topography, biocompatibility and resorbability are generally studied for scaffolds.30 Adding growth factors and MSCs to the scaffolds can improve osteoinductivity and osteoconductivity, respectively. For instance, bone morphogenetic proteins (BMPs) from the TGF-β superfamily are already being used in clinical treatments for bone repair.31 Stem cells, whether directly transplanted or combined with scaffolds, have also shown potential for bone regeneration both in vitro32,33 and in vivo.34 MSCs are the gold standard in bone repair as they have great osteogenic potency and are found in adult tissues.
Biomaterial scaffolds in bone regeneration
Three major classes of biomaterials are used to fabricate scaffolds: metals, ceramics and polymers.35 Their different properties serve different purposes, such as biodegradability which is ideal in bone regenerating scaffolds but not as much in load-bearing devices.36 Metals and alloys can be classified into three groups: titanium- and titanium alloy-based, stainless steels and cobalt-based alloys, all of which are biocompatible, relatively cheap in cost and possess good mechanical properties.37 The second class of biomaterials, ceramics, regroups inorganic, non-metallic compounds, which also include carbon, bioglass and glass-ceramics. The two most common bioceramics used are hydroxyapatite and tricalcium phosphate. Another example of ceramic is pure coral which has been used as a preformed matrix with bone MSCs, loaded with BMP-2 and VEGF.38 Upon implant, different interactions can occur with the surrounding tissue, giving rise to four types of ceramics: (i) bioinert which has minimal interaction with the body, (ii) bioactive which develops a specific biological response at the interface between the scaffold and fractured bone, (iii) porous which allows for cellular migration and infiltration, and (iv) resorbable which is biodegradable and eventually removed from the body.39
Lastly, polymers are widely used in tissue engineering and are generally classified as natural or synthetic. Many of them have demonstrated good mechanical properties, biocompatibility and biodegradability that are generally required for tissue engineering applications.40 Despite all these biomaterials being suitable in these applications, on their own, they can only mimic biological tissues to a certain extent, especially in the case of bone. For example, even though a ceramic can substitute the inorganic component of bone, it lacks the organic counterpart, for instance, collagen that creates the adequate microenvironment for osteogenesis to take place. This limitation makes way for the field of biocomposites.
As defined by Ramakrishna, a composite is a heterogeneous combination of two or more materials, which differ in morphology or composition on a micro- or nanoscale.35 At least one material is the reinforcing phase which is embedded in another material, forming the matrix phase. Composite biomaterials can be grouped according to the shape of their reinforcing materials: (i) fibrous where fibers are incorporated in the matrix and (ii) particulate, whereby particles are introduced instead.41 The structure of a scaffold directly influences tissue formation by affecting cellular functions. The material as mentioned above is of crucial importance, but the geometry of the scaffold, its macro- and microstructures, as well as its physicochemical properties are also greatly considered during the selection and fabrication process. Porosity and interconnectivity between the pores are important for the distribution of nutrients, especially in a microenvironment that lacks neo-vascularisation. Additionally, the size of the pores determines the types of molecules that can navigate through or that can be entrapped for subsequent release. There is no consensus on the optimal porosity and pore size. Small pores (<200 μm) have shown proliferation and differentiation of osteoblasts limited to the periphery of the scaffold, whereas larger pores (>300 μm) have demonstrated bone regeneration throughout the entire scaffold. However, it was observed that the larger the pores the weaker the mechanical strength of the scaffold.29 The surface-to-volume ratio is a characteristic that assesses the quantity of cells that can attach to the scaffold.42 Compression is another very important mechanical property that measures the effects of implantation on the scaffold. The measure for compression is the elastic or Young's modulus, which computes the necessary force to compress a material, otherwise known as the stiffness of the material.43 Different biopolymers have different Young's moduli and the values cover a wider range for composites made of these biopolymers (Table 1). Degradation rate and resorption are assessed in scaffolds in order to find a controllable rate that will match the cell and tissue growth in vitro and in vivo.44
Table 1 Biopolymers’ young modulus
Furthermore, cellular behavior is of the upmost importance when designing a scaffold. The cell–biomaterial interactions are related to the molecular exchanges that take place at the interface between the biomaterial and the surrounding cells. The elemental composition and release from the biomaterial, the van der Waals forces at work, the wettability of the surface, and topography come into play to influence cell adhesion and spreading, cell proliferation and differentiation, as well as protein adhesion and adsorption.42,59 Once more, where monolithic biomaterials may be lacking in stiffness, roughness or biological mimicry, the biocomposites can help to improve the regenerating performance at the cell–biomaterial interface.
Fabrication of scaffolds can be grouped by their various techniques. Textile methods, like electrospinning that make nonwoven fibers have been one of the earlier used to make scaffolds. Particulate-leaching is a technique that can control pore size and once the salts introduced they are leached away. Phase separation is another technique that creates porous scaffolds either by solid–liquid phase separation or by liquid–liquid phase separation. More recently, 3D printing a technology that deposits polymer powders in sequential layers has been reported. Self-assembly is another up-and-coming technique which requires no human intervention to form patterned scaffolds. For a more extensive review on the fabrication methods of scaffolds, please refer to Ma's articles.60,61
Composites can incorporate two or more biomaterials from all three groups: metal, ceramics and polymers including both natural and synthetics ones, depending on the properties sought. When a composite is a mixture only consisting of two or more polymers, it is generally referred to as polymer blend. And while there are many reviews62–64 covering various biocomposites including synthetic polymer blends, little is reported on biocomposites made out of natural polymers as a matrix for bone substitute or scaffolding in the context of osteogenesis. In this article, we aim to provide direction with a comprehensive review of the state-of-the-art of using natural polymers in the fabrication of biocomposite for bone repair (Table 2).
Table 2 Summary of biopolymers in bone regeneration
Biopolymers |
Properties |
Materials added |
In vitro
|
In vivo
|
Clinical trials |
Chitosan (CH) |
• Deacetylated form of chitin |
• GE/silicon dioxide66 |
• Human osteosarcoma (MG63)66 |
• Male KM mice (leg)116 |
|
• Biocompatible |
• nHA67 |
• MG63, Vero, NIH3T3, and nHDF cells67 |
|
|
• Biodegradable |
• Bioactive glass68 |
• MG63, human periosteal-derived precursor cells (PCs)68 |
|
|
• Cellular-binding |
• AL/fucoidan69 |
• MG6369 |
|
|
• Wound-healing |
• CS coated Ti-6Al-4V70 |
• Mouse osteoblast 7F270 |
|
|
• Anti-bacterial |
• GE/rhBMP2116 |
• hMSCs116 |
|
|
• Anti-fungal |
|
|
|
|
Collagen (CO) |
• Fibrous protein |
• Octacalcium phosphate22,23 |
• MC3T3-E124 |
• Male Wistar rats (crania)22 |
• 21 patients (mandibular non-union)119 |
• Most abundant protein in the body |
• PLA/PEG/silica nanospheres loaded with aFGFs24 |
• MC3T3-E173 |
• Male Wistar rats (calvaria)23 |
• 32 patients (periodontal defect)110 |
• Biocompatible |
• Apatite72 |
• MSCs74 |
• Male Sprague-Dawley rats24 |
|
• Biodegradable |
• AL/silica73 |
• MC3T3-E1103 |
• Transgenic mice carrying the pOBCol3.6GFP transgene (calvaria)72 |
|
• Highly elastic |
• Glycosaminoglycan/porous titanium117 |
|
• Male beagle (femur)74 |
|
|
• Non-crystalline HA/ASC/PRP99 |
|
• Sheep (tibia)99 |
|
|
• Calcium sulfate hemihydrate/nHA/loaded with rhBMP2118 |
|
• Male New Zealand White rabbits (condoyle)101 |
|
|
• HA/loaded with bisphosphonates and BMPs103 |
|
• Male Wistar rats (femur)103 |
|
|
• Teicolplanin119 |
|
|
|
|
• Deprotonized cancellous bone particles110 |
|
|
|
Gelatin (GE) |
• Denatured collagen |
• Bioactive glass76 |
• hMSCs75 |
• Male Sprague-Dawley rats (crania)78 |
|
• Thermoreversible gelation |
• Porous HA75 |
• Human osteoblasts77 |
• Mice79 |
|
• Biocompatible |
• PEG/PPG77 |
• MG6378 |
• Male Sprague-Dawley rats (crania)102 |
|
• Biodegradable |
• PVA/BCP78 |
• hBM-MSCs, MC3T379 |
• Male Fischer-344 rats (crania)107 |
|
|
• Hyaluronan/AL79 |
• MSCs102 |
• Male Fischer-344 rats (crania)108 |
|
|
• PCL/HA102 |
|
|
|
|
• PPF/VEGF/BMP2107,108 |
|
|
|
Alginate (AL) |
• Water-soluble copolymers |
• β-TCP80 |
• Human osteoblasts (CRL-11372)80 |
• ICR mice (calvaria)81 |
|
• Extracted from brown seaweed |
• Octacalcium phosphate81 |
• Mouse bone marrow stromal cells (ST-2)81 |
• Male Sprague-Dawley rats (spine)109 |
|
• Biocompatible |
• Fibrin82 |
• hBM-MSCs82 |
|
|
• Biodegradable |
• GE/agarose w/calcium salt of polyphosphate83 |
• Human osteosarcoma cells (SaOS-2)83 |
|
|
|
• Strontium84 |
• BM-MSCs84 |
|
|
|
• Ce(III)85 |
• MG6385 |
|
|
|
• TiO2/simvastatin100 |
• Primary human osteoblasts (hOBs)100 |
|
|
|
• Heparin/BMP2109 |
• C2C12109 |
|
|
Silk fibroin (SF) |
• Linear structure with β-sheet structure |
• nHA86 |
MC3T387 |
• Male New Zealand white rabbits (back and knee)89 |
|
• Biocompatible |
• nHA/HY–dopamine conjugate87 |
• Human gingiva-derived MSCs88 |
• Male New Zealand white rabbits (calvaria)90 |
|
• Physical and mechanical properties |
• Tetracycline88 |
• Rabbit BM-MSCs89 |
• Female nude mice91 |
|
• Easy processability |
• Nano calcium phosphate89 |
• hMSCs90 |
|
|
|
• PCL90 |
• hMSCs91 |
|
|
|
• CS/nHA91 |
|
|
|
Hyaluronan (HY) |
• Nonsulfated glycosaminoglycan |
• Sodium alginate/nano-bioactive glass92 |
• MG6392 |
• Male Wistar rats (femur)96 |
|
• Abundant in the extracellular matrix |
• Poly(dimethylsiloxane)93 |
• MC3T393 |
• New Zealand white rabbit (femur) |
|
• Biocompatible |
• CS/CO-1120 |
• hMSCs120 |
|
|
• Biodegradable |
• Poly(vinyl phosphonic acid)94 |
• HepG294 |
|
|
• Physico-chemical properties |
• Inorganic polyphosphate95 |
• MC3T3-E195 |
|
|
|
• Calcium phosphate NP96 |
• BMSCs97 |
|
|
|
• GE/BCP97 |
|
|
|
Chitosan-based scaffolds
Chitosan is the deacetylated form of chitin, an abundant natural polymer found in the exoskeleton of crustaceans. It is recognized for its biodegradability and biocompatibility.65 Chitosan and ceramics are often combined to compensate for the lack of strength of polymers and the brittleness of ceramics. Some recent examples include the fabrication of a 3D nanocomposite consisting of chitosan, gelatin and nano-silica. This scaffold was obtained by lyophilisation and compared with pure chitosan/gelatin for bone repair. The cellular response to scaffold was studied on an osteosarcoma cell line where the composite biomaterial presented superior qualities for cell attachment/proliferation and mineralization than either chitosan or chitosan/gelatin scaffolds, proving that composite biomaterials offer more than their monolithic compounds.66 A similar scaffold was developed by freeze-drying a hydrogel made from α-chitin and nano-hydroxyapatite (nHA). Cell attachment and viability studies, as well as biomineralization assays, indicated that these scaffolds would be promising in bone repair applications (Fig. 5).67 Gentile et al. investigated a chitosan/gelatin blend containing CEL2, a bioactive glass. The composite was cross-linked with genipin for enhanced mechanical strength.68 Two cell lines were used to test cytocompatibility and proliferation, revealing that osteogenic properties of the scaffolds can be calibrated by controlling the pore size through bioglass content to obtain optimal performance for both soft and hard skeletal tissues. In other cases, composites are made by mixing synthetic and/or natural materials with chitosan, which helps to balance the properties of the polymers used. For example, a chitosan-alginate biocomposite with fucoidan was developed for bone repair applications, in which a MG-63 cell line was used for the in vitro studies. It was found that, when compared to the controls of chitosan and chitosan-alginate, the scaffold increased cellular proliferation as well as enhanced alkaline phosphotase secretion, a mineralization marker.69 Porosity and water retention were also superior in the composite scaffolds, which is an indication of adequate cell adhesion and infiltration as well as good protein adsorption efficiency. Lastly, chitosan and chitosan-based biocomposites are also employed as coatings on metallic implants. For instance, chitosan, alginate-cross-linked chitosan and pectin-cross-linked chitosan were used to coat Ti-6Al-4V surfaces and were tested for cytocompatibility using osteoblasts.70 The titanium–aluminum alloy was found to have higher proliferative properties after coating, especially with cross-linked chitosan.
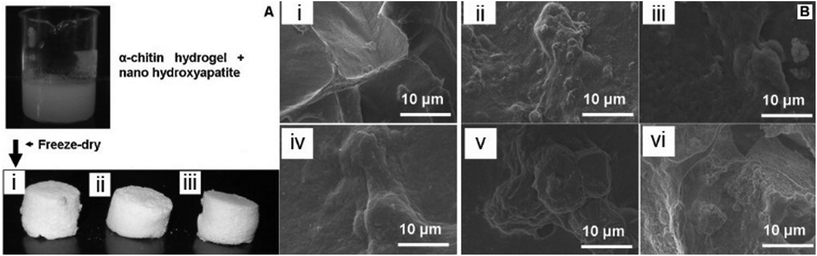 |
| Fig. 5 (A) Representation of composite scaffolds: (i) α-chitin control, (ii) α-chitin + 0.5% nHA composite scaffold and (iii) α-chitin + 1% nHA composite scaffold. (B) (i–iii) Are scanning electron microscopy (SEM) images of cell attachment of α-chitin control, α-chitin + 0.5% nHA and α-chitin + 1% nHA scaffolds respectively at 12 h and (iv–vi) are SEM images of cell attachment of α-chitin control, α-chitin + 0.5% nHA and α-chitin + 1% nHA scaffolds respectively at 72 h (adapted from Kumar et al., 2011).67 | |
Collagen-based scaffolds
Collagen is the most abundant protein in the animal kingdom. It is a highly insoluble fibre that makes up the extracellular matrix and connective tissues.71 Kamakura et al. recently studied the implantation of an octacalcium phosphate (OCP)/collagen sponge in a rat cranial bone defect over a period of 4–12 weeks. A newly formed bone was observed and very few granules from the implants remained, indicating good osteogenesis and resorbability.22 A very similar study using OCP/collagen scaffolds also demonstrated the osteoconductive effects of a polymer–ceramic composite.23 Scaffolds made of shelled silica nanospheres electrosprayed into a 3D foam were implanted in rats for 2 weeks, which showed a favourable tissue response. The constructs were composed of polylactic acid (PLA) and polyethylene glycol (PEG) nanospheres combined with collagen and loaded with acidic FGF.24 Simpler forms of calcium phosphate are also used in combination with polymers to create efficient biocomposite scaffolds. nHA is recognized for its great biocompatibility and osteoconductivity, for which it is often used pre-clinically and clinically for treating bone defects. Xia et al. fabricated a biomimetic collagen–apatite (Col–Ap) by a controllable freeze-casting method.72 The Col–Ap suspensions were prepared by first extracting collagen from rat tails and then freeze-drying it. The collagen was then dissolved under acidic conditions and mixed with simulated body fluid in order to get mineralization (apatite crystals). The Col–Ap solutions were freeze dried and then cross-linked before a subsequent freeze-drying step. It yielded a 3D scaffold that was subsequently tested in mice calvaria bone defects. After 4 weeks, the results showed dynamic new bone formation throughout the entire defect area (Fig. 6). Among the mixed polymer composites, a biomineralized collagen/alginate/silica scaffold was fabricated by Lee et al. and the cellular activities were assessed with pre-osteoblasts.73
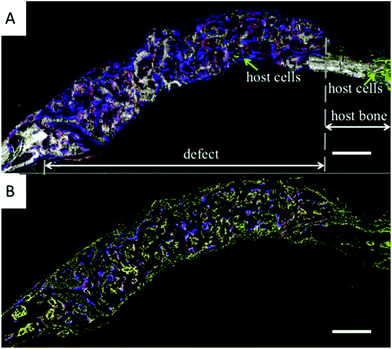 |
| Fig. 6 Green Fluorescent Protein (GFP) images showing bone formation at the cross-section of the calvarial defect implanted with Col–Ap loaded with mCOB cells (A) 10× scanning image with cyan + TRITC + tpz + DF channel. (B) 10× scanning image with cyan + TRITC + tpz + DAPI – 4 weeks. Scale bar: 500 μm (adapted from Xia et al., 2013).72 | |
The results showed that cell proliferation and osteogenic gene expression levels were higher on the composite scaffold versus the chitosan and chitosan/alginate controls. As for the collagen–metal composites being tested in vivo, there is a biphasic type I collagen/glycosaminoglycans/porous titanium (CGT) composite.74 Titanium powder was mixed with hydrogen peroxide, methylcellulose and PEG to form a slurry. The slurry went through a foaming and drying process to give porous titanium shaped cylinders. Type I collagen was mixed with glycosaminoglycans to make a slurry that was subsequently poured over the titanium cylinders and freeze-dried. Those were implanted in a beagle with osteochondral defects, and abundant subchondral bone formation was observed 3 months after the surgery.
Gelatin-based scaffolds
Gelatin is a natural protein with low toxicity that is derived by denaturing collagen. It is ubiquitously used as a gelling agent. In bone tissue repair, a study showed that a porous gelatin-covered HA scaffold had a 163% increase in proliferation when cultured with human MSCs (hMSCs).75 A gelatin-bioactive glass composite scaffold was recently developed by Lacroix et al. and its controlled porosity shows promising potential for bone formation after incubation in simulated body fluid (SBF). After 5 days of immersion into SBF, the formation of calcium phosphate was visible on both the surface of the bioglass particles and the gelatin walls.76 Another study has shown that a poly(ethylene glycol)–poly(propyleneglycol)–poly(ethylene glycol) (PEG–PPG–PEG)/pregelatinized starch composite can be used as a bone wax substitute; cytotoxic assays have revealed that it is harmless to osteoblasts.77 Linh et al. developed fiber mats composed of polyvinyl alcohol (PVA)/gelatin (GE) loaded with various amounts of biphasic calcium phosphate (BCP) nanoparticles.78 Studies in rats showed an increase in bone formation with the 50% PVA/GE-50% BCP blend, indicating its potential use in bone regeneration. Singh et al. presented a 3D gelatin/hyaluronan/alginate (GHA) blend that was freeze-dried and then cross-linked with calcium chloride (CaCl2) (Fig. 7). The implantation of this scaffold in mice demonstrated pronounced osseointegration, recruitment of cells and reduced inflammatory response compared to controls.79
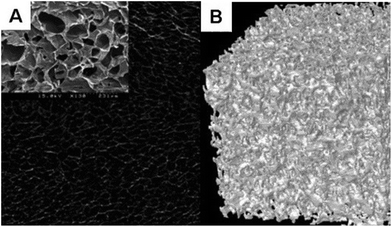 |
| Fig. 7 (A) SEM image shows the open pore architecture and smooth pore walls of the GHA matrix. (B) Micro-CT image shows the presence of interconnected pores which enhances cell recruitment (adapted from Singh et al., 2014).79 | |
Alginate-based scaffolds
Alginate is an anionic linear co-polymer derived from marine seaweed. Like chitosan, it is often used as a biomaterial due to its biocompatibility and biodegradability. Beta-tricalcium phosphate (β-TCP)/alginate scaffolds were recently fabricated via 3D printing and presented promising in vitro results toward bone regeneration applications.80 A mouse calvarial CSD was used to investigate the bone repair capacities of an OCP/alginate scaffold over a period of three weeks. Results showed that the sporadic bone formation around the defect was dependent on the pore size of the scaffold.81 Furthermore, a fibrin and alginate hydrogel appeared to support bone marrow derived MSCs (BM-MSCs) chondrogenesis in vitro.82
Very recently, another hydrogel made of a sodium alginate matrix stabilized with gelatin was tested with human osteoblast-like SaOS-2 cells and was shown to increase cellular mineralization on the surface of the matrix (Fig. 8).83 Lastly, Tu et al. recently studied the biocompatibility of an injectable alginate-strontium hydrogel with BM-MSCs as a precursor for bone tissue repair.84 Morais et al. developed several alginate-based hydrogels with cerium ions, which showed improved MG63 cell activity compared with the control with no Ce(III) ions.85
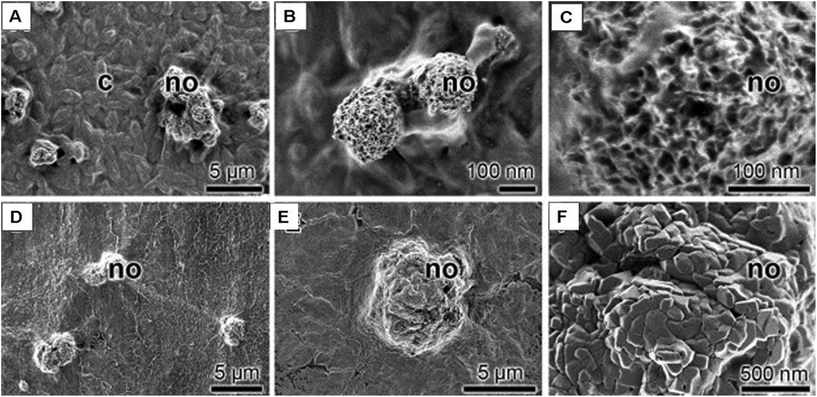 |
| Fig. 8 SEM images show the mineral deposits on SaOS-2 cell surfaces. (A–C) Mineral deposits, nodules (no), formed onto cells, (D–F) nodules (no) formed onto SaOS-2 cells that had been encapsulated into alginate/gelatin hydrogel (adapted from Neufurth et al., 2014).83 | |
Silk-based scaffolds
Silk fibroin (SF) is a linear polypeptide with a β-sheet conformation. It is commonly used in tissue engineering due to its low immunogenicity. It was recently shown that biomineralization could be regulated by the nano-sized features in SF proteins. This occurred due to the repulsive forces resulting from the double layering of SF. With increasing SF concentration, these forces gradually changed the nano-HA morphology. This allowed the SF to function as a template for biomineralization.86 Kim et al. prepared a SF hydrogel with nHA nanoparticles on its surface, and when tested on MC-3T3 cells, the surface-modified hydrogel was shown to have significantly higher proliferation abilities than the pure SF hydrogel group.87 A similar study done with gingiva-derived MSCs (GMSCs) demonstrated excellent cell adhesion and proliferative potential with tetracycline-loaded silk fibroin membranes (TC-SFMs) (Fig. 9).88 Yan et al. recently tested a bilayered silk/silk-nano calcium phosphate scaffold in a rabbit knee CSD model. Firm osseointegration and bone growth was observed in the host tissue.89 A SF/polycaprolactone (PCL) composite was implanted in a calvarial rabbit CSD. Quantitative analysis showed that the composite scaffold generated a larger amount of newly formed bone than both the empty control group and the PCL scaffold group.90 A new composite developed by Lai et al. demonstrated excellent osteogenic properties both in vitro and in vivo. The SF/CS/nHA scaffold in which the nHA was embedded by preelectrospinning in situ promoted proliferation and osteogenic differentiation, as well as ectopic bone formation in nude mice.91
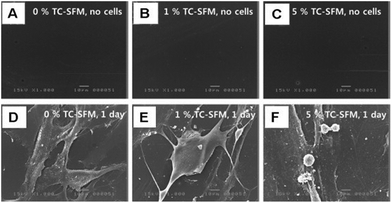 |
| Fig. 9 Cell attachment analysis. (A–C) Negative control groups were observed by SEM (no cells, PBS) at day 1. (D–F) GMSCs attached to the TC-SFMs (0, 1, 5, and 10%) were observed by SEM at day 1. Scale bar in the figures indicates 10 μm (adapted from Jin et al., 2014).88 | |
Hyaluronan-based scaffolds
Hyaluronan (HY) is a linear polysaccharide with repetitive D-glucuronic acid and N-acetyl-D-glucosamine monosaccharide. The adjustable structure and texture of HY makes it a great candidate for bone tissue engineering applications. Sohrabi et al. tested an injectable visco-elastic paste composed of HY/sodium alginate/nano-bioactive glasses on osteoblastic cells. The release of Ca and Si ions, from the bioactive glasses, into the culture media enhanced the alkaline phosphatase activity of the cells.92 Micro-patterning of a HY hydrogel on poly(dimethylsiloxane) (PDMS) was found to enhance adhesion and induce proliferation of MC-3T3, as well as showing excellent biocompatibility.93 A biomineralized hydrogel comprised of HY cross-linked with vinyl phosphonic acid was created to mimic the extracellular matrix for improved bone repair. It was tested on human hepatoma HepG2 cells for viability and did not show any adverse effects.94
The enhancement of osteogenic differentiation was also observed in an investigation by Wu et al. The group conjugated inorganic polyphosphate chains onto HY macromers to make a bioactive hydrogel. When incubated with MC-3T3, osteoconductivity was much higher in the HY/polyphosphate group than the control stimulated with free polyphosphates.95 An injectable nanocomposite scaffold consisting of bisphosphonated (BP) HY and calcium phosphate (CP) nanoparticles was developed by Nejadnik et al. for the repair of rat bone defects. The performance after 1 and 4 weeks was evaluated and it was found that the non-covalent interactions of the bisphosphonate groups and the CP nanoparticles allowed for better cellular infiltration and degradation of the scaffold. Abundant bone formation was observed throughout the material in the bone defect (Fig. 10).96 A HY/GE hydrogel was loaded through a dropwise method into a spongy porous BCP matrix. Implantation of the HY/GE/BCP scaffold in rabbit femurs demonstrated rapid bone formation and collagen mineralization, as well as the positive staining of bone-forming proteins: osteopontin, osteocalcin and collagen type I.97
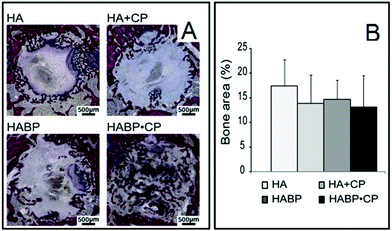 |
| Fig. 10 (A) Histology of covalently (HA, HABP, HA + CP) and non-covalently (HABPCP) cross-linked formulations after 4 weeks of implantation in bone tissue. (B) Bone regeneration after 4 weeks of implantation; error bars indicate the standard deviation (adapted from Nejadnik et al., 2014).96 | |
Composite scaffolds as delivery systems
Scaffolds that act as a delivery system are extremely useful. Not only do they provide a mechanical structure for the new tissue to grow on, they can also enhance bone repair by releasing drugs or growth factors locally. Among the biomolecules used to augment bone-cell response, there is TGF-β, BMP, insulin-like growth factors (IGF), PDGF, and fibroblast growth factors (FGF),3 as well as bisphosphonates and antiresorptive drugs such as Denosumad.98 Biocomposites offer many advantages as delivery scaffolds. For instance, their different materials degrade at different rates, allowing for a controlled release of molecules. Also, the hydrophobic and hydrophilic interactions between the biocomposite and delivery factor can be manipulated for improved molecule entrapment.
The delivery modes can be divided into three categories: (i) molecules released by seeded cells; (ii) molecules entrapped in the scaffold or attached to the surface, and (iii) molecules encapsulated in micro- or nanoparticles within the scaffold. Platelet-rich plasma (PRP), a source of growth factors, has also been integrated into some scaffolds to accelerate bone growth. PRP was used in combination with adipose tissue derived stem cells (ASCs) or BM-MSCs on mineralized collagen sponges.99 The nHA-coated construct was introduced in a sheep tibia CSD model for 26 weeks. The results showed that the BM-MSCs had the greatest osteogenic potential when paired with PRP. Even though the results did not show a significant difference, there was a trend for larger amount of bone formation for the PRP-group. Many molecules are now being directly integrated into the scaffold during synthesis or attached on the surface. For instance, an alginate-coated TiO2 hydrogel containing simvastatin (SIM) was tested in vitro for bone regeneration.100 SIM, known for enhancing bone formation, was mixed in the alginate solution prior to coating. The release was found to be sustained for a period of 15–17 days and to induce osteoblast differentiation. The cumulative release indicated that the drug remained entrapped in the scaffold even after 19 days of incubation.
Another injectable composite was made of nHA/collagen and calcium sulfate hemihydrate, subsequently loaded with rhBMP-2.101 The loaded scaffold was implanted in a rabbit femoral condyle defect and as suspected, was proven to be more bioactive than the scaffold alone. Lee et al. functionalized the interface of an electrospun gelatin/PCL scaffold by attaching proteins (fusion of fibronectin and osteocalcin (FN-OCN)) via a nHA coating.102In vivo testing in a rat calvarial defect model showed a higher amount of new bone formation in the FN-OCN-functionalized biocomposite compared to the control. Another study evaluated the efficacy of a porous composite collagen–nHA scaffold for delivering recombinant BMPs and bisphosphonates.103 The molecules were loaded into the scaffold after a 24-hour incubation at 37 °C following its synthesis. The results showed that a composite construct was ideal for dual delivery systems. Similarly, Quinlan et al. developed a collagen–HA scaffold that delivered rhBMP-2 via PLGA and alginate microparticles. The construct with the PLGA microparticles showed higher pro-osteogenic effect compared to the alginate microparticles; this was demonstrated both in vitro and in vivo (after 8 weeks of implantation in calvarial defects in rats).104 More recently, Ronca et al. tested a PLLA/PCL/hyaluronan derivative functionalized with BMP-7.105 The 3D tube-shaped scaffold was made via a combination of phase inversion/salt leaching and filament-winding technology. The entrapment and sustained release of the protein were successful and led to positive outcomes in the in vivo testing. The composite was found to be osteoinductive due to the scaffold and osteoconductive due to the BMP-7 release.
In another study, a gel scaffold made from cellulose and heparin showed osteogenesis via the targeted delivery of BMP-7 in vitro.106
To increase the efficacy of a delivery system, researchers opt for encapsulating molecules in particles. This ensures a controlled release profile that can be altered by modifying the particles’ properties. A dual delivery system of BMP-2 and vascular endothelial growth factor (VEGF) encapsulated in gelatin microparticles and embedded in a porous poly(propylene fumarate) (PPF) scaffold was studied by Patel et al.107 and Young et al.108 The dual release achieved full bone union in two-thirds of the CSD rats as opposed to only a third of the rats in the control groups which were only implanted with the unloaded PPF scaffold. The effectiveness of alginate microbeads in a poly-epsilon caprolactone TCP scaffold (mPCL-TCP) was investigated by Abbah et al.109 The microbeads, containing heparin and BMP-2, were loaded into the pores right before implantation into rats. Compared to a collagen scaffold, the composite dual delivery system demonstrated increased de novo bone formation. The above reported studies support the efficacy of composite biomaterials over monolithic biomaterials as drug delivery systems.
Composite biomaterials in clinical trials
There are currently clinical trials that use commercially available osteoconductive composite biomaterials as bone substitutes. For instance a study on periodontal defects used a composite bovine-derived xenograft with a collagen membrane (BDX Coll + GTR) to treat deep intra-bony defects.110 The scaffold is formulated by embedding deprotonized cancellous bone particles in the collagen matrix. The results of 32 patients were evaluated one year after the treatment, and they demonstrated a much higher, significant improvement over the control group, which was treated with access flap surgery. In an early clinical trial on patients with post-operative tumor lesions, a composite calcium sulfate/calcium phosphate injectable scaffold was used to improve bone repair as shown by the gradual resorption of the scaffold and new bone growth in 27 out of the 29 patients included in the trial.111 In 11 out of 13 patients, the application of a composite biomaterial containing calcium sulphate and HA for the treatment of osteolytic bone tumours was also reported to consolidate bone formation with great integration of the graft material into the host bone.112 More recently, studies have shown that combining scaffolds with growth factors help improve periodontal infrabony defects. An example is a biphasic HA/β-TCP composite with an enamel matrix derivative113 which clearly demonstrated that the composite materials in combination with biological molecules perform better than a monolithic unblended material. TricOs, macroporous biphasic ceramic phosphate granules, was recently used in association with fibrin to fill the bony defects of seven patients’ tibia. However, the results were still at a preliminary stage and the healing processes were analysed by X-ray, showing some new bone formation.114
Summary and perspectives
To date, the review of the literature evidently demonstrates that composite biomaterials significantly improve bone regeneration. Incorporating metals and ceramics into readily available polymers appears to be the new trend to follow for scaffolds mimicking the skeletal microenvironment (Table 1). Hence, composite biomaterials could be used to provide both strength and improved biological properties, such as cellular adhesion. Various material combinations show potential applications as bone substitutes. However, two shortcomings were revealed through the study of the current literature: (i) the lack of adequate in vivo data and clinical trials and (ii) the limited testing of different material blends.
There are presently numerous in vitro assays that assess the cellular behaviour of scaffolds. Even though these preliminary studies are necessary, they are unfortunately not sufficient to evaluate the compatibility and efficiency of scaffolds in the body. Animal studies will however demonstrate how different the requirements for the composite scaffold are from that of in vitro studies. For example, mechanical loads on the scaffold cannot be determined via cellular assays, nor can the immune system reaction. And with the lack of in vitro studies comes the scarcity of clinical trials; the few that were reported above sum up the most recent studies. To counter the second limitation, more combinations of biomaterials need to be tested.
This will allow the finding of appropriate scaffolds for different models of bone defects. An area that is particularly unexplored is the combination of polymers, ceramics and biological elements. Polymers can provide the right amount of elasticity; ceramics can provide the structural backbone, while growth factors can accelerate the regeneration processes. It is highly recommended that future studies focus on different combinations of bone graft substitutes as was also suggested by Schindler et al.112 Other parameters that can also be optimised for clinical applications are the mode of admission of the scaffold, such as injectability, the integration of a delivery system and the rate of gelling of a hydrogel.115 The present review demonstrates the significance of combining different materials with the aim of accelerating bone regeneration following a bone defect, notably reinforcing natural polymers. Among the various blends that can be made, natural polymer/ceramics composites seem to be the most prevalent. The efficacy of these scaffolds can be improved by entrapping growth factors and cytokines inside. Recent findings involving the entrapment of drugs inside composite scaffolds have shown increased and faster bone formation; this area of biomaterials should without a doubt be investigated thoroughly.
Acknowledgements
The authors acknowledge the CIHR and NSERC contribution through CIHR-POP, CHRP, NSERC-Discovery grants. K. Jahan expresses her gratitude to the Faculty of Dentistry for salary support through the Excellence Award, as well as Dr Mina Mekhail and Dr Laila Benameur for their guidance and Dr Amir Foudeh and Dr Feriel Melaine for their assistance with Lightroom, Inkscape and ChemBioUltra.
Notes and references
- P. P. Spicer, J. D. Kretlow, S. Young, J. A. Jansen, F. K. Kasper and A. G. Mikos, Nat. Protoc., 2012, 7, 1918–1929 CrossRef CAS PubMed.
- G. M. Calori, E. Mazza, M. Colombo and C. Ripamonti, Injury, 2011, 42(Supplement 2), S56–S63 CrossRef PubMed.
-
M. Ulrich and W. Hans Peter, Bone and Cartilage Engineering, Springer, Berlin, Heidelberg, 2006, ch. 3, pp. 47–63 Search PubMed.
-
L. C. U. a. Junqueira, J. Carneiro and A. N. Contopoulos, in A Concise medical library for practitioner and student, Lange Medical Publications, Appleton & Lange, Lange Medical Books/McGraw Hill, McGraw Hill, Los Altos, Calif., Norwalk, Conn., New York, 13th edn, 2013 Search PubMed.
-
A. Yael, C. Jung, D. Jean, J. Vladimir, W. Robert and R. Connie, OpenStax College, Biology, OpenStax CNX, 2013 Search PubMed.
-
U. Meyer and H. P. Wiesmann, in Bone and Cartilage Engineering, Springer, Berlin, Heidelberg, 2006, ch. 2, pp. 7–46 Search PubMed.
-
T. Yang, M. Grover, K. S. Joeng and B. Lee, in Primer on the Metabolic Bone Diseases and Disorders of Mineral Metabolism, John Wiley & Sons, Inc., 2013, pp. 119–126 Search PubMed.
-
C. Colnot and T. Alliston, in Bone and Development, ed. F. Bronner, M. C. Farach-Carson and H. I. Roach, Springer, London, 2010, vol. 6, ch. 2, pp. 25–37 Search PubMed.
-
U. Meyer and H. P. Wiesmann, in Bone and Cartilage Engineering, Springer, Berlin, Heidelberg, 2006, ch. 4, pp. 65–72 Search PubMed.
- E. M. Bueno and J. Glowacki, Synth. Lect. Tissue Eng., 2011, 3, 1–220 CrossRef.
-
D. J. J. de Gorter and P. ten Dijke, in Primer on the Metabolic Bone Diseases and Disorders of Mineral Metabolism, John Wiley & Sons, Inc., 2013, pp. 15–24 Search PubMed.
-
Q. Wang and E. Seeman, in Primer on the Metabolic Bone Diseases and Disorders of Mineral Metabolism, John Wiley & Sons, Inc., 2013, pp. 127–134 Search PubMed.
-
C. Qian, W. Lei, W. Zhengke, S. Xiaojuan, L. Junming and Y. Xu, Current Topics in Bone Biology, World Scientific Publishing Co., River Edge, NJ, USA, 2005 Search PubMed.
-
H. C. Anderson and I. Shapiro, in Bone and Development, ed. F. Bronner, M. C. Farach-Carson and H. I. Roach, Springer, London, 2010, vol. 6, ch. 3, pp. 39–64 Search PubMed.
-
M. J. Zuscik, in Primer on the Metabolic Bone Diseases and Disorders of Mineral Metabolism, John Wiley & Sons, Inc., 2013, pp. 90–98 Search PubMed.
- C. Antonio, S. Brunella, R. Francesca, M. Filippo Maria, B. Paolo and G. Carlo, Ann. Fac. Med. Vet.-Univ. Parma, 2006, 26, 97–110 Search PubMed.
- R. Marsell and T. A. Einhorn, Injury, 2011, 42, 551–555 CrossRef PubMed.
- I. Drosse, E. Volkmer, S. Seitz, H. Seitz, R. Penzkofer, K. Zahn, U. Matis, W. Mutschler, P. Augat and M. Schieker, Tissue Eng., Part C, 2008, 14, 79–88 CrossRef PubMed.
- C. Bosch, B. Melsen and K. Vargervik, J. Craniofac. Surg., 1998, 9, 310–316 CrossRef CAS PubMed.
- J. P. Schmitz and J. O. Hollinger, Clin. Orthop. Relat. Res., 1986, 299–308 CAS.
- P. Niemeyer, K. Fechner, S. Milz, W. Richter, N. P. Suedkamp, A. T. Mehlhorn, S. Pearce and P. Kasten, Biomaterials, 2010, 31, 3572–3579 CrossRef CAS PubMed.
- S. Kamakura, K. Sasaki, T. Homma, Y. Honda, T. Anada, S. Echigo and O. Suzuki, J. Biomed. Mater. Res., Part A, 2007, 83, 725–733 CrossRef PubMed.
- T. Kawai, T. Anada, Y. Honda, S. Kamakura, K. Matsui, A. Matsui, K. Sasaki, S. Morimoto, S. Echigo and O. Suzuki, Tissue Eng., Part A, 2009, 15, 23–32 CrossRef CAS PubMed.
- T. H. Kim, M. Eltohamy, M. Kim, R. A. Perez, J. H. Kim, Y. R. Yun, J. H. Jang, E. J. Lee, J. C. Knowles and H. W. Kim, Acta Biomater., 2014, 10, 2612–2621 CrossRef CAS PubMed.
- J. O. Hollinger and J. C. Kleinschmidt, J. Craniofac. Surg., 1990, 1, 60–68 CrossRef CAS PubMed.
- P. V. Giannoudis, T. A. Einhorn and D. Marsh, Injury, 2007, 38(Suppl 4), S3–S6 CrossRef PubMed.
- P. V. Giannoudis, T. A. Einhorn, G. Schmidmaier and D. Marsh, Injury, 2008, 39(Suppl 2), S5–S8 CrossRef PubMed.
- G. M. Calori and P. V. Giannoudis, Injury, 2011, 42, 1191–1193 CrossRef PubMed.
- A. R. Amini, C. T. Laurencin and S. P. Nukavarapu, Crit. Rev. Biomed. Eng., 2012, 40, 363–408 CrossRef PubMed.
- P. Janicki and G. Schmidmaier, Injury, 2011, 42(Supplement 2), S77–S81 CrossRef PubMed.
- G. Pelled, A. Ben-Arav, C. Hock, D. G. Reynolds, C. Yazici, Y. Zilberman, Z. Gazit, H. Awad, D. Gazit and E. M. Schwarz, Tissue Eng., Part B, 2010, 16, 13–20 CrossRef CAS PubMed.
- Y. H. Liao, Y. H. Chang, L. Y. Sung, K. C. Li, C. L. Yeh, T. C. Yen, S. M. Hwang, K. J. Lin and Y. C. Hu, Biomaterials, 2014, 35, 4901–4910 CrossRef CAS PubMed.
- W. TheinHan, J. Liu, M. Tang, W. Chen, L. Cheng and H. H. Xu, Bone Res., 2013, 4, 371–384 CrossRef PubMed.
- H. Ding, Y. S. Gao, Y. Wang, C. Hu, Y. Sun and C. Zhang, Stem Cells Dev., 2014, 23, 990–1000 CrossRef CAS PubMed.
-
M. R. Seeram Ramakrishna, T. S. Sampath Kumar and W. O. Soboyejo, in Biomaterials, CRC Press, 2010, pp. 1–33 Search PubMed.
- L. Horstink, H. T. Faber, M. J. F. de Wolf, C. A. J. Dun, C. W. R. J. Cremers and M. K. S. Hol, Otol. Neurotol., 2012, 33, 1013–1017, DOI:1010.1097/MAO.1010b1013e318259b318236c.
-
M. R. Seeram Ramakrishna, T. S. Sampath Kumar and W O. Soboyejo, in Biomaterials, CRC Press, 2010, pp. 161–186 Search PubMed.
- C. Xiao, H. Zhou, G. Liu, P. Zhang, Y. Fu, P. Gu, H. Hou, T. Tang and X. Fan, Biomed. Mater., 2011, 6, 015013 CrossRef PubMed.
-
M. R. Seeram Ramakrishna, T. S. Sampath Kumar and W. O. Soboyejo, in Biomaterials, CRC Press, 2010, pp. 187–215 Search PubMed.
-
M. R. Seeram Ramakrishna, T. S. Sampath Kumar and W. O. Soboyejo, in Biomaterials, CRC Press, 2010, pp. 217–262 Search PubMed.
-
M. R. Seeram Ramakrishna, T. S. Sampath Kumar and W. O. Soboyejo, in Biomaterials, CRC Press, 2010, pp. 263–298 Search PubMed.
-
U. Meyer and H. P. Wiesmann, Bone and cartilage engineering, Springer, Berlin, New York, 2006 Search PubMed.
- J. R. Woodard, A. J. Hilldore, S. K. Lan, C. J. Park, A. W. Morgan, J. A. Eurell, S. G. Clark, M. B. Wheeler, R. D. Jamison and A. J. Wagoner Johnson, Biomaterials, 2007, 28, 45–54 CrossRef CAS PubMed.
- D. W. Hutmacher, Biomaterials, 2000, 21, 2529–2543 CrossRef CAS PubMed.
- T. Nishino, R. Matsui and K. Nakamae, J. Polym. Sci., Part B: Polym. Phys., 1999, 37, 1191–1196 CrossRef CAS.
- H. L. Kim, G. Y. Jung, J. H. Yoon, J. S. Han, Y. J. Park, D. G. Kim, M. Zhang and D. J. Kim, Mater. Sci. Eng., C, 2015, 54, 20–25 CrossRef CAS PubMed.
- J. Zhang, A. Deng, A. Zhou, Y. Yang, L. Gao, Z. Zhong and S. Yang, J. Biomater. Sci., Polym. Ed., 2015, 26, 585–599 CrossRef CAS PubMed.
- N. Sasaki and S. Odajima, J. Biomech., 1996, 29, 655–658 CrossRef CAS PubMed.
- S. Ramalingam, A. Al-Rasheed, A. ArRejaie, N. Nooh, M. Al-Kindi and K. Al-Hezaimi, Odontology, 2015, 1–12 Search PubMed.
- C. Amador, M. W. Urban, S. Chen, Q. Chen, K.-N. An and J. F. Greenleaf, IEEE Trans. Biomed. Eng., 2011, 58, 1706–1714 CrossRef PubMed.
- K. Siimon, H. Siimon and M. Jarvekulg, J. Mater. Sci.: Mater. Med., 2015, 26, 5375 CrossRef PubMed.
- A. Sharma, S. Bhat, V. Nayak and A. Kumar, Mater. Sci. Eng., C, 2015, 47, 298–312 CrossRef CAS PubMed.
- C. D. Markert, X. Guo, A. Skardal, Z. Wang, S. Bharadwaj, Y. Zhang, K. Bonin and M. Guthold, J. Mech. Behav. Biomed. Mater., 2013, 27, 115–127 CrossRef CAS PubMed.
- M. S. Kim and G. Kim, Carbohydr. Polym., 2014, 114, 213–221 CrossRef CAS PubMed.
- H. S. Kim, M. Song, E. J. Lee and U. S. Shin, Mater. Sci. Eng., C, 2015, 51, 139–147 CrossRef CAS PubMed.
- H. J. Lee, A. Sen, S. Bae, J. S. Lee and K. Webb, Acta Biomater., 2015, 14, 43–52 CrossRef CAS PubMed.
- S. C. Choi, M. A. Yoo, S. Y. Lee, H. J. Lee, D. H. Son, J. Jung, I. Noh and C. W. Kim, J. Biomed. Mater. Res., Part A, 2015, 103, 3072–3080 CrossRef CAS PubMed.
-
C. Jiang, X. Wang, R. Gunawidjaja, Y.-H. Lin, M. K. Gupta, D. L. Kaplan, R. R. Naik and V. V. Tsukruk, Mechanical properties of robust ultrathin silk fibroin films, DTIC Document, 2007.
- S. G. Piperni, E. R. Takamori, S. Sartoretto, K. B. Paiva, J. M. Granjeiro, R. C. de Oliveira and W. F. Zambuzzi, Arch. Biochem. Biophys., 2014, 561, 88–98 CrossRef PubMed.
- P. X. Ma, Mater. Today, 2004, 7, 30–40 CrossRef CAS.
- P. X. Ma, Adv. Drug Delivery Rev., 2008, 60, 184–198 CrossRef CAS PubMed.
- G. H. Billström, A. W. Blom, S. Larsson and A. D. Beswick, Injury, 2013, 44(Supplement 1), S28–S33 CrossRef.
- D. Ozdil and H. M. Aydin, J. Chem. Technol. Biotechnol., 2014, 89, 1793–1810 CrossRef CAS.
- T. Garg and A. K. Goyal, Expert Opin. Drug Deliv., 2014, 11, 767–789 CrossRef CAS PubMed.
- M. Fan, Q. Hu and K. Shen, Carbohydr. Polym., 2009, 78, 66–71 CrossRef CAS.
- K. C. Kavya, R. Jayakumar, S. Nair and K. P. Chennazhi, Int. J. Biol. Macromol., 2013, 59, 255–263 CrossRef CAS PubMed.
- P. T. S. Kumar, S. Srinivasan, V.-K. Lakshmanan, H. Tamura, S. V. Nair and R. Jayakumar, Int. J. Biol. Macromol., 2011, 49, 20–31 CrossRef CAS PubMed.
- P. Gentile, M. Mattioli-Belmonte, V. Chiono, C. Ferretti, F. Baino, C. Tonda-Turo, C. Vitale-Brovarone, I. Pashkuleva, R. L. Reis and G. Ciardelli, J. Biomed. Mater. Res., Part A, 2012, 100, 2654–2667 CrossRef PubMed.
- J. Venkatesan, I. Bhatnagar and S. K. Kim, Mar. Drugs, 2014, 12, 300–316 CrossRef CAS PubMed.
- H.-Y. Lin and J.-H. Chen, Carbohydr. Polym., 2013, 97, 618–626 CrossRef CAS PubMed.
-
H. F. Lodish, Molecular cell biology, W.H. Freeman and Co., New York, 2013 Search PubMed.
- Z. Xia, X. Yu, X. Jiang, H. D. Brody, D. W. Rowe and M. Wei, Acta Biomater., 2013, 9, 7308–7319 CrossRef CAS PubMed.
- H. Lee, Y. Kim, S. Kim and G. Kim, J. Mater. Chem. B, 2014, 2, 5785–5798 RSC.
- X. Duan, X. Zhu, X. Dong, J. Yang, F. Huang, S. Cen, F. Leung, H. Fan and Z. Xiang, Mater. Sci. Eng., C, 2013, 33, 3951–3957 CrossRef CAS PubMed.
- A. Tampieri, G. Celotti, E. Landi, M. Montevecchi, N. Roveri, A. Bigi, S. Panzavolta and M. C. Sidoti, J. Mater. Sci.: Mater. Med., 2003, 14, 623–627 CrossRef CAS PubMed.
- J. Lacroix, E. Jallot and J. Lao, Chem. Eng. J., 2014, 256, 9–13 CrossRef CAS.
- J. Suwanprateeb, W. Suvannapruk, F. Thammarakcharoen, W. Chokevivat and P. Rukskul, J. Mater. Sci.: Mater. Med., 2013, 24, 2881–2888 CrossRef CAS PubMed.
- N. T. Linh, K. H. Lee and B. T. Lee, J. Biomed. Mater. Res., Part A, 2013, 101, 2412–2423 CrossRef PubMed.
- D. Singh, A. Tripathi, S. Zo, D. Singh and S. S. Han, Colloids Surf., B, 2014, 116, 502–509 CrossRef CAS PubMed.
- G. S. Diogo, V. M. Gaspar, I. R. Serra, R. Fradique and I. J. Correia, Biofabrication, 2014, 6, 025001 CrossRef CAS PubMed.
- T. Fuji, T. Anada, Y. Honda, Y. Shiwaku, H. Koike, S. Kamakura, K. Sasaki and O. Suzuki, Tissue Eng., Part A, 2009, 15, 3525–3535 CrossRef CAS PubMed.
- K. Ma, A. L. Titan, M. Stafford, C. Zheng and M. E. Levenston, Acta Biomater., 2012, 8, 3754–3764 CrossRef CAS PubMed.
- M. Neufurth, X. Wang, H. C. Schroder, Q. Feng, B. Diehl-Seifert, T. Ziebart, R. Steffen, S. Wang and W. E. Muller, Biomaterials, 2014, 35, 8810–8819 CrossRef CAS PubMed.
- Y. Tu, T. Wu, A. Ye, J. Xu, F. Guo and X. Cheng, Chin. J. Repar. Reconstr. Surg., 2013, 27, 1499–1505 CAS.
- D. S. Morais, M. A. Rodrigues, M. A. Lopes, M. J. Coelho, A. C. Mauricio, R. Gomes, I. Amorim, M. P. Ferraz, J. D. Santos and C. M. Botelho, J. Mater. Sci.: Mater. Med., 2013, 24, 2145–2155 CrossRef CAS PubMed.
- X. Huang Xiaowei, X. Liu, S. Liu, A. Zhang and Q. Lu, J. Biomed. Mater. Res., Part B, 2014, 102, 1720–1729 CrossRef PubMed.
- H. H. Kim, J. B. Park, M. J. Kang and Y. H. Park, Int. J. Biol. Macromol., 2014, 70, 516–522 CrossRef CAS PubMed.
- S.-H. Jin, H. Kweon, J.-B. Park and C.-H. Kim, J. Surg. Res., 2014, 192, e1–e9 CrossRef CAS PubMed.
- L.-P. Yan, J. Silva-Correia, M. B. Oliveira, C. Vilela, H. Pereira, R. A. Sousa, J. F. Mano, A. L. Oliveira, J. M. Oliveira and R. L. Reis, Acta Biomater., 2015, 12, 227–241 CrossRef CAS PubMed.
- S. Kim Beom, K. E. Park, M. H. Kim, H. K. You and J. Lee, Int. J. Nanomed., 2015, 10, 485–502 Search PubMed.
- G.-J. Lai, K. T. Shalumon and J.-P. Chen, Int. J. Nanomed., 2015, 10, 567–584 CAS.
- M. Sohrabi Mehri, S. Hesaraki and A. Kazemzadeh, J. Biomed. Mater. Res., Part B, 2014, 102, 561–573 CrossRef PubMed.
- S. Park Hyo, S. Y. Lee, H. Yoon and I. Noh, Pure Appl. Chem., 2014, 86, 1911–1922 Search PubMed.
- Y. Kim So and J.-S. Park, J. Appl. Polym. Sci., 2014, 131 Search PubMed.
- T. H. Wu Andy, T. Aoki, M. Sakoda, S. Ohta and S. Ichimura, Biomacromolecules, 2015, 16, 166–173 CrossRef CAS PubMed.
- M. R. M. R. Nejadnik, X. Yang, M. Bongio, H. S. Alghamdi and J. J. J. P. Van den Beucken, Biomaterials, 2014, 35, 6918–6929 CrossRef CAS PubMed.
- T. B. Nguyen Thuy Ba Linh and B.-T. Lee, Tissue Eng., Part A, 2014, 20, 1993–2004 CrossRef PubMed.
- M. L. Brandi, Expert Opin. Investig. Drugs, 2012, 21, 1169–1176 CrossRef CAS PubMed.
- P. Niemeyer, K. Fechner, S. Milz, W. Richter, N. P. Suedkamp, A. T. Mehlhorn, S. Pearce and P. Kasten, Biomaterials, 2010, 31, 3572–3579 CrossRef CAS PubMed.
- H. Pullisaar, H. Tiainen, M. A. Landin, S. P. Lyngstadaas, H. J. Haugen, J. E. Reseland and E. Ostrup, J. Tissue Eng., 2013, 4, 2041731413515670 Search PubMed.
- J. Liu, K. Mao, Z. Liu, X. Wang, F. Cui, W. Guo and S. Yang, PLoS One, 2013, 8, e75668 CAS.
- J. H. Lee, J. H. Park, A. El-Fiqi, J. H. Kim, Y. R. Yun, J. H. Jang, C. M. Han, E. J. Lee and H. W. Kim, Acta Biomater., 2014, 10, 2750–2761 CrossRef CAS PubMed.
- C. M. Murphy, A. Schindeler, J. P. Gleeson, N. Y. Yu, L. C. Cantrill, K. Mikulec, L. Peacock, F. J. O'Brien and D. G. Little, Acta Biomater., 2014, 10, 2250–2258 CrossRef CAS PubMed.
- E. Quinlan, A. Lopez-Noriega, E. Thompson, H. M. Kelly, S. A. Cryan and F. J. O'Brien, J. Controlled Release, 2015, 198, 71–79 CrossRef CAS PubMed.
- A. Ronca, V. Guarino, M. G. Raucci, F. Salamanna, L. Martini, S. Zeppetelli, M. Fini, E. Kon, G. Filardo, M. Marcacci and L. Ambrosio, J. Biomater. Appl., 2014, 29, 715–727 CrossRef CAS PubMed.
- M. Fan, J. Yan, H. Tan, D. Ben, Q. He, Z. Huang and X. Hu, Macromol. Biosci., 2014, 14, 1521–1527 CrossRef CAS PubMed.
- Z. S. Patel, S. Young, Y. Tabata, J. A. Jansen, M. E. K. Wong and A. G. Mikos, Bone, 2008, 43, 931–940 CrossRef CAS PubMed.
- S. Young, Z. S. Patel, J. D. Kretlow, M. B. Murphy, P. M. Mountziaris, L. S. Baggett, H. Ueda, Y. Tabata, J. A. Jansen, M. Wong and A. G. Mikos, Tissue Eng., Part A, 2009, 15, 2347–2362 CrossRef CAS PubMed.
- S. A. Abbah, J. Liu, J. C. Goh and H. K. Wong, Tissue Eng., Part A, 2013, 19, 350–359 CrossRef CAS PubMed.
- A. Sculean, G. C. Chiantella, P. Windisch, N. B. Arweiler, M. Brecx and I. Gera, J. Clin. Periodontol., 2005, 32, 720–724 CrossRef PubMed.
-
R. K. Heck Jr., Treatment of Benign Bone Lesions with a Calcium Sulfate/Calcium Phosphate Composite: Early Clinical Experience, Wright Medical Technology, Inc., Memphis, TN, 2008 Search PubMed.
- O. S. Schindler, S. R. Cannon, T. W. Briggs and G. W. Blunn, J. Orthop. Surg., 2008, 16, 66–74 CAS.
- D. De Leonardis and M. Paolantonio, J. Periodontol., 2013, 84, 444–455 CrossRef CAS PubMed.
-
M. Durand, D. Chauveaux, M. Moinard, T. Fabre, J. L. Rouvillain, M. Bagot d'Arc and G. Daculsi, TricOsTM and Fibrin Sealant Combined for Bone Defect Filling: from Pre-Clinical Tests to Prospective Clinical Study, Preliminary Human Data, 2008.
- M. Mekhail, J. Daoud, G. Almazan and M. Tabrizian, Adv. Healthcare Mater., 2013, 2, 1126–1130 CrossRef CAS PubMed.
- L. Cao, J. A. Werkmeister, J. Wang, V. Glattauer, K. M. McLean and C. Liu, Biomaterials, 2014, 35, 2730–2742 CrossRef CAS PubMed.
- X. Duan, X. Zhu, X. Dong, J. Yang, F. Huang, S. Cen, F. Leung, H. Fan and Z. Xiang, Mater. Sci. Eng., C, 2013, 33, 3951–3957 CrossRef CAS PubMed.
- J. Liu, K. Mao, Z. Liu, X. Wang, F. Cui, W. Guo, K. Mao and S. Yang, PLoS One, 2013, 8, e75668 CAS.
- S. Rupprecht, L. Petrovic, B. Burchhardt, J. Wiltfang, F. W. Neukam and K. A. Schlegel, J. Biomed. Mater. Res., Part B, 2007, 83, 314–319 CrossRef PubMed.
- S. Mathews Smitha, R. Bhonde, P. K. Gupta and S. Totey, J. Biomed. Mater. Res., Part B, 2014, 102, 1825–1834 CrossRef PubMed.
|
This journal is © The Royal Society of Chemistry 2016 |
Click here to see how this site uses Cookies. View our privacy policy here.