Preparation of hierarchical enamel-like structures from nano- to macro-scale, regulated by inorganic templates derived from enamel†
Received
5th September 2014
, Accepted 1st October 2014
First published on 3rd October 2014
Abstract
The artificial construction of enamel-like structure has been focusing on the utilization of an organic matrix, since proteins are believed to be essential in directing apatite orientation during enamel formation. Here we report that the orientation of apatite can be regulated by inorganic substrates and we successfully constructed enamel-like cross-arranged structure using natural enamel as template. The templating effect of inorganic substrates on apatite orientation may lead to the design of biomimetic materials.
1. Introduction
Enamel, the exterior coating of vertebrate teeth, is a highly mineralized tissue. It is composed of more than 95% carbonated hydroxyapatite (HA) by weight with less than 2% organic material.1 Enamel possesses a unique complex structure, which contains two main structural components: the prismatic and interprismatic enamel.2 These structures project from the dentine–enamel junction to the enamel surface. The prisms are nearly cylindrical and are aligned parallel to each other and separated by the interprismatic structure. Each prism is composed of rod-shaped bundles of nano-HA crystallites, which are oriented roughly parallel to the prism long axis within a prism. The interprismatic enamel is apatite crystallites outside the prisms, which have a different orientation degree as compared to crystallites in prisms. This special cross-arranged structure makes enamel the hardest tissue with remarkable resilience in human body.3 The development process of enamel is extremely complex and has not been clearly defined yet. It is believed that the initial formation of enamel apatite occurs under a protein-rich matrix gel condition and the major protein of forming enamel, amelogenin, plays an essential role in regulation of the mineralization and structural organization of the enamel layer of the teeth.4,5 During enamel maturation, most of these proteins that mediate the mineralization of apatite crystals are gradually degraded and removed.6 As the outermost layer of teeth, enamel can be damaged because of the acid-producing bacteria or acidic food during lifetime use since HA is unstable under acidic conditions. The mature enamel is acellular, and as a non-living tissue, it cannot regenerate after substantial mineral loss.
The artificial construction of enamel-like structure has been tried for decades for its significance in both tooth enamel repair/protection and hierarchical material design. But yet, few practical advances have been yielded. Because it is thought that amelogenin is necessary for structure formation during the natural enamel mineralization process, the use of amelogenin7,8 or other organic molecules such as amino acids9 to regulate apatite structures has also been extensively investigated. However, only nanoparticles with simple ordered structures at nanoscale could be fabricated under the control of these organic molecules, and these particles cannot assemble into ordered structures mimicking enamel structure at both nano-scale and macro-scale. Apatite crystals with certain degree of orientation can be grown on various templates by using many kinds of methods, such as the regulation of amelogenin,10,11 using hydrogen peroxide containing-calcium phosphate paste,12 re-mineralization under a gel system13 and a hydrothermal method with controlled release of calcium.14 However, the best structure obtained was the overall perpendicular growth of the crystals along c-axis, and cross-arranged apatite structure of natural enamel with both prismatic and interprismatic components, which are the key characteristic of enamel structure, still failed to be constructed. The reason is that the formation process of natural enamel in vivo is under the regulation of proteins and precise control of genes15 and the mechanism of such a system is too complicated to be clearly understood yet, so this process is extremely difficult to be simulated under in vitro conditions. Nowadays, it is still a challenge to prepare apatite crystallites that resemble natural enamel with both prismatic and interprismatic structures in both nano- and macro-scale in an in vitro process.
In recent years, the use of a hard template to control the structure of apatite crystals has attracted considerable attention. Our previous studies have shown that various kinds of inorganic hard precursors, such as monetite, xonotlite and calcium carbonate, are able to guide the formation of apatite crystals.16,17 The results demonstrate that the structure of apatite could be well controlled by the inorganic precursors under certain reaction conditions, and suggest that more complex hierarchical structures mimicking designs of natural structures from nano- to macro-scale may be obtained by selecting of the structure of inorganic substrates and controlling the reaction conditions. Based on these findings, we assume that apatite with enamel-like structure mimicking both prismatic and interprismatic structures in both nano- and macro-scale can be constructed by using a proper inorganic template under appropriate experimental conditions without the involvement of organic molecules. Here, we report a facile way to prepare cross-arranged enamel-like apatite (HA and fluorine-substituted HA (FHA)) with both prismatic and interprismatic structure by using natural enamel as a template. The natural enamel served as both mineral deposition substrate and template-directing reagent, and in contrast to the organic template method, the orientation of the apatite crystals is proved to be guided by the inorganic structure of natural enamel and both prismatic and interprismatic structures of enamel is perfectly duplicated.
2. Experimental section
2.1 Preparation of enamel-like apatite layer
Bovine enamel slices were used as a template to grow enamel-like apatite layer. They were polished and then slightly eroded with 0.1 M citric acid for 1 minute to expose the microstructure of the enamel surface before being used as a template. The reaction solution contained 0.468 g EDTA–Ca–Na2, 0.41 g Na3PO4 with 0.01 g NH4F for building enamel-like FHA (or without NH4F for HA), which was dissolved into 20 mL distilled water. The pH of the solution was about 12. After the solution was transferred into stainless steel autoclaves, enamel templates were vertically placed in the stainless steel autoclaves and then hydrothermally treated for 24 h at 120°C. After reaction, the enamel samples were ultrasonically cleansed with distilled water for 1 minute. The samples were dried naturally in air before further characterization. For cross-section examination, the enamel was embedded with epoxy resin and longitudinally cut in half. The section was meticulously polished to expose the delicate structure of the coating.
2.2 Exploration of the formation mechanism of the enamel-like apatite layer
The enamel-like apatite layer is prepared under hydrothermal condition in a solution obtained by dissolving 0.468 g EDTA–Ca–Na2, 0.41 g Na3PO4 and 0.01 g NH4F in 20 mL distilled water at 120°C (T) for 24 h (t) and the pH value of this solution without adjustment was 12. To investigate the mechanism of the formation of the enamel-like apatite layer, three groups of experiments (1) t = 1 h, 3 h or 6 h, T = 120°C, pH = 12; (2) t = 24 h, T = 60°C or 90°C, pH = 12; (3) t = 24 h, T = 120°C, pH = 6 (the pH of the solution was adjusted by 37% hydrochloric acid) were first carried out to illustrate the effect of the reaction conditions, such as reaction time, reaction temperature and pH value, on the orientation of the crystals. To evaluate the effect of residual proteins on the formation of enamel-like structure, the enamel was calcined at 400°C for 6 h to remove the residue proteins in natural enamel before utilizing as template. To explain the templating role of the natural enamel substrate, apatite layers were also grown on calcium phosphate ceramics (HA, β-TCP) under the same experimental condition as that for preparing enamel-like apatite layer using natural enamel as the templates (hydrothermally reacted in a pH 12 solution, which contained 0.468 g EDTA–Ca–Na2, 0.41 g Na3PO4 and 0.01 g NH4F in 20 mL distilled water at 120°C for 24 h). To prepare HA ceramics with different grain sizes, they were calcined at 1150 °C/1 h for the smallest grain size, 1200 °C/5 h for medium grain size and 1300 °C/10 h for the biggest grain size. The pretreatment of the ceramic substrates was the same as those enamel samples, which were polished and then etched with 0.1 M citric acid for 1 minute.
2.3 Evaluation and optimization of the properties of enamel-like apatite layer
To analyze the acid-resistant stability of the enamel-like FHA layer, the rest of the samples were embedded with epoxy resin, leaving just the apatite layer exposed. Then each of them was immersed in 10 mL of acetate buffer (pH 5.5) and kept at 37°C. After certain times, 0.1 mL of acetate solution was gently extracted from the top to examine the ion concentration and replaced with fresh acetate solution. The stability of sound enamel and acid etched enamel (0.1 M citric acid for 1 minute) was tested in the same way for comparison.
To mimic the enamel-like structure, samples with enamel-like apatite surface layer were immersed in 5 wt% and 10 wt% gelatin solution for 1 h at 37°C, respectively, and the immersion process was repeated 3 times. Then the sample was rinsed with distilled water several times and dried naturally in air before further characterization.
2.4 Characterization
The morphologies and EDS analysis of the materials were characterized by scanning electron microscopy (SEM, S-4800, Hitachi). The crystalline phases were identified using an X-ray diffractometer (XRD, D/max2550 V, Rigaku) with Cu-Ka radiation at an operating condition of 40 kV and 100 mA. The ion concentration was analyzed by inductively coupled plasma optical emission spectrometer (ICP, Varian 715-ES, Agilent Technologies). Testing of the hardness of the samples was carried out by Nanoindenter (G200, Agilent Technologies).
3. Results and discussion
3.1 Characterization of enamel-like structure
The microstructure of the natural enamel is shown in Fig. 1a and b, in which a flat surface is observed under low magnification of scanning electron microscopy (SEM) (Fig. 1a), and an enlarged image (Inset in Fig. 1a) shows a separated elliptical enamel prism unit with a dimension of about 6 μm in length and 3 μm in width. Apatite nanorods in the prism are highly organized along the c-axis and the nanorods around the prism, which have a different orientation degree as those in the prism, are interprismatic enamel. The cross-arranged structure can be clearly observed from the partial enlarged view of the boundary of the prismatic and interprismatic enamel, as seen in Fig. 1b. Using such an enamel template and reacting it under a unique hydrothermal condition, which is modified from our previous report,18 we successfully duplicated the sophisticated hierarchical enamel structure consisting of FHA (Fig. 1c–f) or HA (Fig. S1†). The surface images (Fig. 1c and d) show that the structure of the synthetic FHA is extremely similar to natural enamel both in nano-scale and macro-scale. From the low magnification image (Fig. 1c), the hierarchical structure of FHA layer can be clearly observed. The partial enlarged view of the boundary of the prismatic and interprismatic enamel (Fig. 1d) further confirms that the growth layer is composed of apatite crystallites with two different orientation directions. The cross-section images (Fig. 1e and f) show that the thickness of the grown apatite layer is about 1.7 μm. It is interesting to notice that the direction of each single FHA crystal maintained the same orientation as the natural enamel underneath (Fig. 1f); therefore the FHA layer maintained the same structure as the template that they grew on.
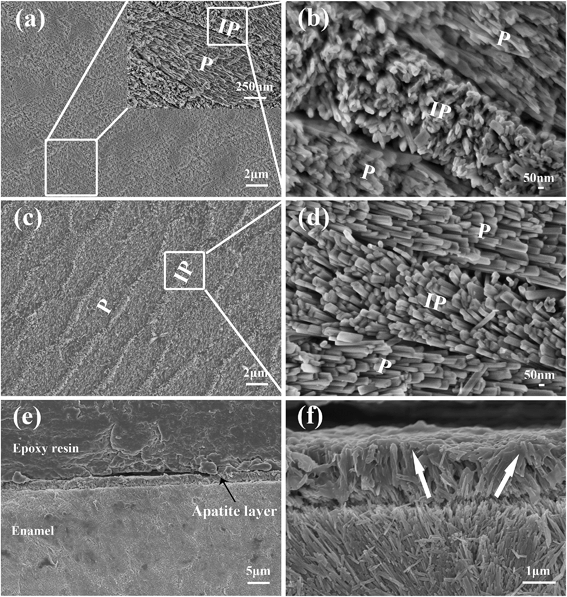 |
| Fig. 1 SEM images of enamel-like apatite layer grown on natural enamel. (a and b) Natural enamel, inset of (a) shows a separated enamel prism; (c and d) surface morphology of the apatite layer grown for 24 h; (e and f) cross-section images. (P) prismatic enamel; (IP) interprismatic enamel. The arrows in (f) indicate the direction of the newly grown apatite crystals, which was the same as natural enamel underneath. | |
It is known that the orientation degree of the apatite nanorods has a significant effect on the peak intensity of the XRD patterns of the samples, and can be evaluated quantitatively by the ratio of the intensities of peak (002) and (211).10,11,17,19,20 Therefore, we utilized the X-ray diffraction (XRD) analysis to further confirm the formation of enamel mimetic apatite layer on the natural enamel template. In previous studies, in which just prism like apatite was obtained, the peak intensity of (002) increased several times after apatite mineralization and the intensity of the (211) decreased, owing to the overall perpendicular growth of the crystals along c-axis.21,22 In our results, as shown in Fig. 2, the peak intensity before and after the apatite growth is extremely close, and this confirms that the orientation of the grown apatite crystallites is similar to the natural enamel. The peak intensity ratio of I002
:
I211 (R) was further calculated to quantitatively evaluate the orientation degree of apatite. The R values for natural enamel and FHA layer were 1.02 and 0.94, respectively, which were very close, whereas the R value for apatite crystals that highly oriented along c-axis could reach 3.10.17 This result, together with the observation of SEM, suggests that the newly formed apatite has similar orientation as the natural enamel in both prismatic and interprismatic parts.
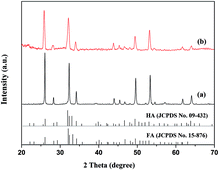 |
| Fig. 2 XRD patterns of natural enamel before and after growth of the FHA layer. (a) Before growth of FHA; (b) after growth of FHA. All the diffraction peaks correspond well with HA (JCPDS no. 09-432) or fluorapatite (FA, JCPDS no. 15-876). | |
3.2 Formation mechanism of enamel-like structure
Natural enamel contains minute amount of proteins, which might also affect HA crystal growth. In order to determine if the inorganic structure of the enamel plays the key role in directing HA growth, we calcined the natural enamel to remove the residue proteins before using it as template to confirm that the residual proteins in natural enamel make no contribution on the orientation of apatite crystals. The result showed no obvious change of the inorganic structure of natural enamel after calcination, and the re-grown layer on the calcined enamel (Fig. 3) still possessed the hierarchical structure, indicating that the proteins in natural enamel have no manifest influence on the orientation of newly formed apatite crystals, and the enamel-like structure is fabricated under the regulation of the inorganic template of natural enamel.
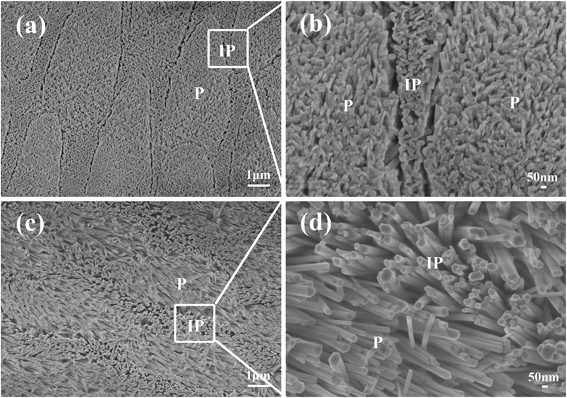 |
| Fig. 3 SEM images of apatite layer grown on calcined enamel. (a and b) Calcined enamel, after removal of proteins, the inorganic structure is not changed; (c and d) the grown apatite layer has the cross-arranged hierarchical structure. (P) Prismatic enamel; (IP) interprismatic enamel. | |
To illustrate the formation mechanism of the enamel-like apatite layer, we firstly investigated the reaction conditions, such as reaction time, temperature and pH value, on the orientation of the enamel-like apatite layer (SEM images are shown in Fig. S2–S4†). The results demonstrated that reaction time, temperature and pH value have no evident effect on the orientation of apatite crystals. In addition, the microstructure of the enamel-like apatite layer can be tailored by changing these reaction conditions.
To further explore the regulating mechanism of inorganic substrate on apatite formation, we utilized HA ceramics, which have the same composition but different structure with natural enamel as apatite deposition template. HA ceramics are composed of a large number of grains (Fig. 4a and b), with random orientations. After hydrothermal reaction, it is interesting to notice that, although newly formed apatite crystals were disordered with various arrangement angles at the macro-scale level (Fig. 4c and e), the apatite crystals on each grain in the ceramic disc formed bundles with well parallel aligned orientation, indicating that apatite crystals grown on each single crystal grain of the ceramic template had the same orientation angle and the growth of the HA crystals was regulated by the orientation of the single crystal grain (Fig. 4d and f). When we changed the grain size of the ceramic discs, the orientation of HA crystal bundles was changed at macro-scale, but the size and orientation of the HA nano crystals on each grain remained the same, which confirmed the regulation effect of the crystal grain of the template, and suggests a possibility to control the formation of HA nano crystals at macro-scale by adjusting the crystal grain size of the template (Fig. S5†).
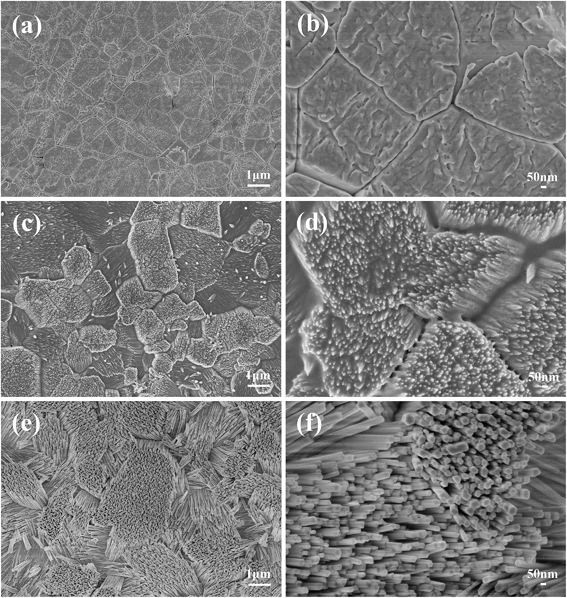 |
| Fig. 4 SEM images of the apatite crystals grown on HA ceramic. (a and b) HA ceramic before hydrothermal reaction; (c and d) growth for 40 minute; (e and f) growth for 24 h. | |
The regulation mechanism of HA growth on inorganic templates can be explained by nucleation kinetics in the heterogeneous nucleation process. Kinetically, the orientation of the nucleation phase grown on the substrate is mainly controlled by the interfacial free energy between the substrate and nucleation phase (γ) and the supersaturation of the solution (σ).23–25 The applied inorganic substrates provide templating for apatite mineral deposition through the heterogeneous nucleation process. In general, γ is determined by the structural match between the nucleation phase and substrate, and the optimal structural match will lead to the lowest γ, which will kinetically favor the orientated growth of the crystal on the substrate. However, the orientated HA growth occurs only at low σ, because under the condition of the optimal structural match, the nucleation barrier will be significantly affected by σ, and an increase of σ will result in the decrease of the nucleation barrier. At high σ, the nucleation barrier is very low and becomes less important and crystals with random orientations will be obtained, which is the so called “supersaturation-driven interfacial structure mismatch effect”.23–25
The above explanation can be confirmed, if we change the supersaturation degree of the reaction solution by changing the ion concentration. If we reduce the supersaturation degree, an apatite layer with ordered and compact structure should still be obtained. In contrast, increased supersaturation would lead to the formation of disordered structure. With this idea in mind, we carried out experiments with different supersaturations. Our results demonstrated that, when the ion concentrations of the reaction solution were doubled, the crystals in the interprismatic region kept compact and ordered, whereas those in the prism cores appeared to be a little disordered (Fig. S6†), and when the ion concentrations were further increased 4 times, the re-grown layer became totally disordered and the templating relation between apatite crystallites and the substrate no longer existed (Fig. 5a and b). In contrast, when other conditions were kept unchanged and the concentrations of all the ions (Ca, P and F) were decreased to 1/5 of the original, the enamel template surface was not thoroughly covered by newly grown apatite crystals, probably due to too low ion concentration (Fig. 5c and d). But yet, the apatite layer still possessed a perfect cross-arranged structure. When the ion concentrations were further decreased to 1/6, the concentration of the solution was too low and there was no apatite grown on enamel surface after reaction for 24 h. These results were in accordance with our explanation that the templating relation between the substrate and crystalline phase is controlled by the interfacial free energy, and only occurs under low supersaturation of the reaction solution.
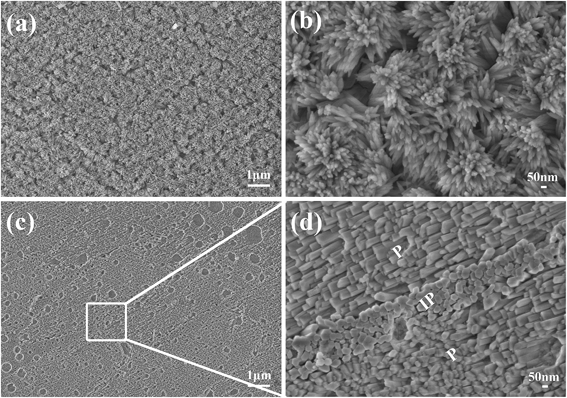 |
| Fig. 5 SEM images of the grown apatite layer at different concentrations using natural enamel as template. (a and b) The concentration of all the ions increased to four times, the apatite layer became disordered; (c and d) the concentration decreased to 1/5 of original solution, the apatite layer was compact and ordered. (P) Prismatic enamel; (IP) interprismatic enamel. | |
It is generally believed that organic matrix plays an essential role in regulating the orientation of apatite during dental enamel development,1,4 so the artificial fabrication of enamel-like structure mainly focused on the application of organic molecules to regulate the growth of apatite crystallites.7,10,11,20,26 However, with the help of organic molecules, only structures similar to enamel prism at nanoscale can be obtained in vitro, and the preparation of enamel-like structure with both prismatic and interprismatic components from nano- to macroscale had not been achieved till date. Very recently, researchers have found that water also plays a structuring role in directing apatite orientation during bone biomineralization.27 Based on our studies of inorganic template induced apatite orientation, we speculate that the proteins and water controlled the orientation of the first mineral deposition at the beginning of the mineral deposition, and further orientation of the HA crystals might be mainly regulated by the inorganic compositions. This is possible especially for the formation of natural enamel, in which only a small amount of proteins are present and most of them are concentrated in the dentine–enamel junction where the enamel tissue is initially formed.28
3.3 Properties of enamel-like structure
One of the potential applications of the enamel-mimetic apatite coating is the repair and protection of natural teeth. Therefore, we evaluated the acid-resistant ability and mechanical property of the enamel-like FHA layer that were newly grown on an enamel surface. As shown in Fig. 6a, the release speed of Ca of the newly grown FHA layer was considerably slower than those of sound enamel and acid-etched enamel under acidic condition, indicating that this FHA coating was more resistant to acid etching and it might be applied to protect original enamel from acid damage. The load-displacement curves of samples measured by nano indentation are illustrated in Fig. 6b. The hardness of the natural enamel was about 4.53 ± 0.31 GPa and the hardness of acid etched enamel that we utilized as templates decreased to 2.53 ± 0.21 GPa due to the removal of protein components. The re-grown apatite coating has a hardness of 1.84 ± 0.29 GPa. Although this is lower than the acid etched natural enamel, it is higher than the hardness of dentine, which is 1.05 ± 0.15 GPa. Most interesting is that if we recombine the enamel-like apatite layer with proteins, such as gelatin, by infiltration of gelatin into the gaps between nanocrystals of the apatite layer (Fig. S7†), the hardness of the FHA–gelatin layer increased to 4.20 ± 0.55 GPa, which was close to natural enamel.
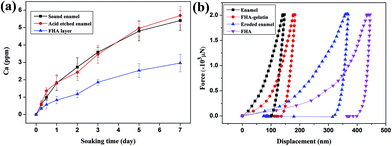 |
| Fig. 6 Chemical stability and mechanical properties of the FHA. (a) Change in the concentration of Ca when soaked in acetate buffer. The release speeds of Ca from sound enamel and acid etched enamel are similar, whereas that from FHA layer is much slower. (b) Loading–unloading curves of nanoindentation tests at maximum loading force of 2000 μN, loading rate of 500 μN s−1 and holding time of 3 s. | |
4. Conclusions
In summary, we have for the first time successfully duplicated the multi-level structure of natural enamel using a facile one-step hydrothermal method without the involvement of any organic molecules. The orientation of the apatite crystals is regulated by the inorganic structure of natural enamel. The phenomenon is well explained by interfacial structural match between the nucleation phase and substrate to reduce the nucleation barrier, which only occurs at low supersaturation of the reaction solution, owing to the “supersaturation-driven interfacial structure mismatch effect”. The enamel-like FHA layer showed a higher stability in acid environment as compared with natural enamel, and the enamel-like apatite/gelatin composites revealed excellent hardness, which is close to the natural enamel. Our results suggest that the application of apatite substrate with unique crystal structures as templates is a useful approach to design and fabricate biomimetic biomaterials for biomedical applications.
Acknowledgements
The authors gratefully acknowledge the support of Natural Science Foundation of China (Grant no.: 81171458, 81190132) and the Funds of the Shanghai Institute of Ceramics, Chinese Academy of Sciences for Innovation of Science and Technology (Grant no. Y26ZC1110G).
Notes and references
- A. Veis, Science, 2005, 307, 1419–1420 CrossRef CAS PubMed.
- M. C. Maas and E. R. Dumont, Evol. Anthr., 1999, 8, 133–152 CrossRef.
- L. H. He and M. V. Swain, J. Mech. Behav. Biomed. Mater., 2008, 1, 18–29 CrossRef PubMed.
- C. Du, G. Falini, S. Fermani, C. Abbott and J. Moradian-Oldak, Science, 2005, 307, 1450–1454 CrossRef CAS PubMed.
- C. W. Gibson, Z. A. Yuan, B. Hall, G. Longenecker, E. H. Chen, T. Thyagarajan, T. Sreenath, J. T. Wright, S. Decker, R. Piddington, G. Harrison and A. B. Kulkarni, J. Biol. Chem., 2001, 276, 31871–31875 CrossRef CAS PubMed.
- A. G. Fincham, J. Moradian-Oldak and J. P. Simmer, J. Struct. Biol., 1999, 126, 270–299 CrossRef CAS PubMed.
- P.-A. Fang, J. F. Conway, H. C. Margolis, J. P. Simmer and E. Beniash, Proc. Natl. Acad. Sci. U. S. A., 2011, 108, 14097–14102 CrossRef CAS PubMed.
- X. Yang, L. Wang, Y. Qin, Z. Sun, Z. J. Henneman, J. Moradian-Oldak and G. H. Nancollas, J. Phys. Chem. B, 2010, 114, 2293–2300 CrossRef CAS PubMed.
- H. Pan, J. Tao, X. Xu and R. Tang, Langmuir, 2007, 23, 8972–8981 CrossRef CAS PubMed.
- Y. Fan, Z. Sun and J. Moradian-Oldak, Biomaterials, 2009, 30, 478–483 CrossRef CAS PubMed.
- Y. Fan, J. R. Nelson, J. R. Alvarez, J. Hagan, A. Berrier and X. Xu, Acta Biomater., 2011, 7, 2293–2302 CrossRef CAS PubMed.
- K. Yamagishi, K. Onuma, T. Suzuki, F. Okada, J. Tagami, M. Otsuki and P. Senawangse, Nature, 2005, 433, 819 CrossRef CAS PubMed.
- S. Busch, Angew. Chem., Int. Ed., 2004, 43, 1428–1431 CrossRef CAS PubMed.
- H. Chen, Z. Tang, J. Liu, K. Sun, S.-R. Chang, M. C. Peters, J. F. Mansfield, A. Czajka-Jakubowska and B. H. Clarkson, Adv. Mater., 2006, 18, 1846–1851 CrossRef CAS.
- M. L. Paine, S. N. White, W. Luo, H. Fong, M. Sarikaya and M. L. Snead, Matrix Biol., 2001, 20, 273–292 CrossRef CAS.
- K. Lin, X. Liu, J. Chang and Y. Zhu, Nanoscale, 2011, 3, 3052–3055 RSC.
- X. Liu, K. Lin, C. Wu, Y. Wang, Z. Zou and J. Chang, Small, 2014, 10, 152–159 CrossRef CAS PubMed.
- Y. Wang, C. Wu, K. Lin and J. Chang, Chem.–Asian J., 2013, 8, 990–996 CrossRef CAS PubMed.
- L. Li, C. Mao, J. Wang, X. Xu, H. Pan, Y. Deng, X. Gu and R. Tang, Adv. Mater., 2011, 23, 4695–4701 CrossRef CAS PubMed.
- D. Wu, J. Yang, J. Li, L. Chen, B. Tang, X. Chen, W. Wu and J. Li, Biomaterials, 2013, 34, 5036–5047 CrossRef CAS PubMed.
- R. Xie, Z. Feng, S. Li and B. Xu, Cryst. Growth Des., 2011, 11, 5206–5214 CAS.
- Y. Yin, S. Yun, J. Fang and H. Chen, Chem. Commun., 2009, 5892–5894 RSC.
- X. Y. Liu and S. W. Lim, J. Am. Chem. Soc., 2003, 125, 888–895 CrossRef CAS PubMed.
- X. Y. Liu and C. S. Strom, J. Chem. Phys., 2000, 113, 4408–4411 CrossRef CAS PubMed.
- H. Jiang and X.-Y. Liu, J. Biol. Chem., 2004, 279, 41286–41293 CrossRef CAS PubMed.
- R. Kniep and S. Busch, Angew. Chem., Int. Ed., 1996, 35, 2624–2626 CrossRef CAS.
- Y. Wang, S. Von Euw, F. M. Fernandes, S. Cassaignon, M. Selmane, G. Laurent, G. Pehau-Arnaudet, C. Coelho, L. Bonhomme-Coury, M.-M. Giraud-Guille, F. Babonneau, T. Azaïs and N. Nassif, Nat. Mater., 2013, 12, 1144–1153 CrossRef CAS PubMed.
- L. C. Palmer, C. J. Newcomb, S. R. Kaltz, E. D. Spoerke and S. I. Stupp, Chem. Rev., 2008, 108, 4754–4783 CrossRef CAS PubMed.
Footnote |
† Electronic supplementary information (ESI) available: Structural characteristics of references. See DOI: 10.1039/c4tb01476f |
|
This journal is © The Royal Society of Chemistry 2015 |
Click here to see how this site uses Cookies. View our privacy policy here.