DOI:
10.1039/C5RA20015F
(Paper)
RSC Adv., 2015,
5, 97127-97132
A stable 3D porous coordination polymer as multi-chemosensor to Cr(IV) anion and Fe(III) cation and its selective adsorption of malachite green oxalate dye†
Received
28th September 2015
, Accepted 3rd November 2015
First published on 5th November 2015
Abstract
Based on Zn(NO3)2·6H2O and 4,4′-((5-carboxy-1,3-phenylene)bis(oxy))dibenzoic acid (H3cpbda), a 3D porous coordination polymer, {[Zn2.5(cpbda)(OH)2]·solvent2}n (solvent = DMF and DMA) (1), was synthesized by three solvent-thermal methods. The complex was characterized by single-crystal X-ray diffraction, IR spectroscopy, power X-ray diffraction, thermal-gravimetric analysis and fluorescence spectroscopy. Owing to its good thermal stability and fluorescence, the sensing experiments (eighteen kinds of cations and eleven kinds of anions) and the further titration processes show the complex can sensitively detect the Fe(III) metal cation and Cr(VI) anions (Cr2O72− and CrO42−) by the quenching responses. In addition, further adsorption research of seven dyes shows the complex 1 can selectively adsorb the Malachite Green Oxalate (MGO) dye.
Introduction
Luminescent coordination polymers (LCPs) have been proved to be excellent luminescent materials for their tunable structure, prominent optical properties, and relatively long emission wavelengths even in the visible region.1 The porous coordination polymers (PCPs) are a new class of metal–organic materials with porous architectures, which have shown a variety of potential applications.2 Particularly, numbers of luminescent PCPs have been synthesized, and widely investigated as sensing materials.3 The chemosensors are of great importance for public health and environment protection due to their capabilities of detecting of heavy metal ions, toxic organic matters, etc.4
As it is known, iron (Fe3+) is an essential element in human body and plays an important role in the transport and storage of oxygen.5 Besides, among the two common oxidation states of chromium, Cr(III) and Cr(VI), the Cr(VI) is highly toxic and can easily migrate in groundwater. Recently, it reported a metal–organic hybrid sorbent can reductive removal of Cr(VI) from aqueous solution.6 The detection of such kind of metal ions and toxicants has been a hot topic for scientists. However the detection of Cr(III) and Cr(VI) in mixed solution is hard to accomplish because of their similarities. Especially the Cr2O72− and CrO42−, they both are Cr(VI) anions. Up to now, there is not an effective approach to detect Cr(III) cation and Cr(VI) anions respectively, using a coodination polymer sensor.
On the other hand, the separation and purification potentials of PCPs in the liquid phase are remarkable. The removal of organic dyes (such as Methylene Blue, Crystal Violet, Rhodamine B, Pyronin Y, Pyronin B, Thionine, Toluidine Blue O, Azure A, Brilliant Green and Methyl Yellow) from wastewater is vital for human life, which remains rarely explored to date.7 Therefore, the guest-detection and dye-adsorption in liquid phase are becoming two significant applications for coordination polymers.
In this work, it reports a highly stable PCP, {[Zn2.5(cpbda)(OH)2]·solvent2}n (solvent = DMF and DMA) (1). This crystal was first synthesized by Zheng's group, and its gas adsorptions and solvatochromic behaviors were investigated.8 Furthermore, based on our previous research backgrounds on H3cpbda ligands,9 it is considered that the PCPs constructed by H3cpbda would be of great application potentials. Using Zn(NO3)2·6H2O and H3cpbda, the complex 1 was synthesized by three solvent-thermal methods (Scheme 1), which was further characterized by single-crystal X-ray diffraction, IR spectroscopy, power X-ray diffraction, thermal-gravimetric analysis and fluorescence spectroscopy.
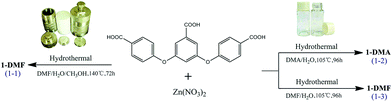 |
| Scheme 1 The synthesis methods of complex 1. | |
Owing to its good thermal stability and fluorescence, the sensing experiments of complex 1 on eighteen cations (Ni2+, Pb2+, Co2+, Cu2+, Cd2+, Zn2+, Ag+, Mn2+, Cr3+, Bi3+, Fe3+, Ca2+, Mg2+, Ba2+, Sr2+, Zr4+, K+, Na+), eleven anions (Cl−, Br−, I−, CO32−, NO3−, SO42−, ClO3−, PO43−, Cr2O72−, CrO42−, SCN−) and the further titration processes were investigated by the quenching responses. Moreover, the adsorptions of seven aqueous organic dye solutions, Methyl Green (MG), Xylenol Orange (XO), Malachite Green Oxalate (MGO), Congo Red (CR), Alizarin Red (AR), Methyl Orange (MO), Cresol Red (CR), have been investigated. Moreover, the maximum dye absorption capability and recycle absorption properties are also studied.
Experimental
Materials and physical measurements
The reagents were used directly as supplied commercially without further purification. Infrared spectra on KBr pellets were recorded on a Bruker Equinox-55 spectrometer in the range of 4000–400 cm−1. Luminescence spectra for the solid samples were investigated with a Hitachi F-4500 fluorescence spectrophotometer. The X-ray powder diffraction pattern was examined with a Pigaku D/Max 3III diffractometer. Thermal analysis was determined with a Netzsch STA 449C microanalyzer under flowing N2 atmosphere at a heating rate of 10 °C min−1.
Preparation of {[Zn2.5(cpbda)(OH)2]·solvent2}n (1)
1-1 (1-DMF). Zn(NO3)2·6H2O (0.125 mmol/37.3 mg), H3cpbda (0.05 mmol/19.7 mg), N,N-dimethylformamide (DMF, 3 mL), H2O (3 mL), methanol (CH3OH, 3 mL) and triethylamine (25%, 0.1 mL) were mixed in a 25 mL Teflon-lined stainless steel vessel. The mixture was sealed and heated at 140 °C for 72 h, and then cooled to room temperature at a rate of 5°C h−1. Finally, colorless block-like crystals of 1-1 were obtained in 78% yield (based on H3cpbda) and were isolated by washing with DMF/H2O/CH3OH, and dried in air. IR (KBr, cm−1) (Fig. S6†): 3614s, 3437s, 3075s, 2923s, 1914w, 1663s, 1600vs, 1568vs, 1389vs, 1306m, 1234m, 1163m, 1111m, 1093m, 990m, 897w, 855w, 785s, 702m, 632w, 496w, 446w.
1-2 (1-DMA). Zn(NO3)2·6H2O (0.125 mmol/37.3 mg), H3cpbda (0.05 mmol/19.7 mg), N,N-dimethylacetamide (DMA, 4 mL), H2O (4 mL) and NaOH (25%, 0.1 mL) were mixed in a 10 mL glass bottle. The mixture was heated at 105 °C for 72 h, and then colorless block-like crystals of 1-2 were obtained in 82% yield (based on H3cpbda) and were isolated by washing with DMA/H2O, and dried in air. IR (KBr, cm−1) (Fig. S7†): 3734m, 3616m, 3438m, 3074m, 2923m, 1915w, 1662s, 1604s, 1563vs, 1501m, 1390vs, 1307m, 1231s, 1162m, 1114m, 1093m, 992m, 963w, 896m, 854m, 787m, 704m, 637m, 497w, 448w.
1-3 (1-DMF). The synthesis of 1-3 was similar to that described above, except that the solvent DMA was replaced by DMF. The yield was 84% (based on H3cpbda).
Crystallographic data collection and refinement
Single-crystal X-ray diffraction analysis of the complex was carried out on a Bruker SMART APEX II CCD diffractometer equipped with a graphite monochromated MoKα radiation (λ = 0.71073 Å) using ϕ/ω scan technique at room temperature. The structures were solved using direct methods and successive Fourier difference synthesis (SHELXS-97),10 and refined using the full-matrix least-squares method on F2 with anisotropic thermal parameters for all non-hydrogen atoms (SHELXL-97).11 All non-hydrogen atoms were refined anisotropically. The hydrogen atoms of organic ligands were placed in calculated positions and refined using a riding on attached atoms with isotropic thermal parameters 1.2 times those of their carrier atoms. Table S1† summarizes the crystal data for 1 and details of data refinements and collection. Table S2† gives the selected angles and bond lengths.
Results and discussion
Description of the crystal structures
[Zn2.5(cpbda)(OH)2]n (1). Complex 1 crystallizes in the Triclinic P
space group, and the asymmetric unit consists of three Zn(II) atoms, one cpbda3− ligand and two deprotonated coordinated water molecules (Fig. 1). The disordered solvent molecules in crystal lattice were omitted in the refining procedure. The Zn1 and Zn3 exhibit the distorted octahedral configurations, and the Zn2 shows four-coordinated tetrahedral configuration. The Zn–O bond distances range from 1.924(4) to 2.435(3) Å, and the bond angles around the Zn(II) atom are at the range of 78.40(12) and 180.00(19)°(Table S2†).
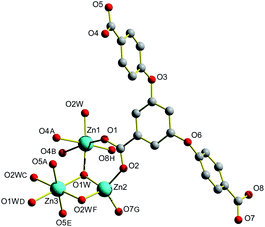 |
| Fig. 1 The coordination environment of Zn(II) in 1. Symmetry codes: A, −x + 2, −y + 1, −z + 1; B, x, y − 1, z; C, −x + 2, −y, −z + 1; D, −x + 3, −y, −z + 1; E, x + 1, y − 1, z; F, x + 1, y, z; G, −x + 3, −y, −z; H, −x + 2, −y, −z. | |
The Zn(II) atoms are connected by coordinated water molecules, forming a novel double-strand 1D Zn–O–Zn chain (Fig. 2b). The 1D chains bond to the T-shaped cpbda3− ligands via the Zn–O bonds, showing a 3D structure (Fig. S1 and S2†). It contains two different shapes of open channels with dimensions of approximately 8.8 × 7.4 and 15.7 × 3.4 Å2 (excluding van der Waals radii of the atoms) along the [001] (Fig. 2a). The removal of water and DMF molecules gives rise to the accessible void volume of 24.4%, as calculated by PLATON.
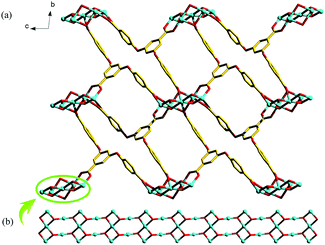 |
| Fig. 2 The 3D structure of 1. (a) The two 1D open channels in 3D framework, viewed along the a axis. (b) The double-strand 1D Zn–O–Zn chain in 1, viewed along the b axis. | |
The topology of 1 viewed along the a axis is shown in Fig. S3.† The cpbda3− bond with seven Zn(II) ions, can be simplified as a seven-connect node, while the two deprotonated water molecules (OH−) act as the three-connect nodes. And the Zn1, Zn2 and Zn3 are 6-, 4- and 6-connect node respectively. The 3D structure of 1 can be described as a (5, 7, 8, 8)-network with the Point symbol of (34·44·52)2(34.45.56.66)2(36.411.56.65)2(38.48.58.64). The views of b and c axis are shown in Fig. S4 and S5.†
PXRD and TGA results
The complexes exhibit highly synthesized yields. In order to confirm the phase purity of the bulk materials, PXRD analyses of 1-DMF and 1-DMA were carried out. The PXRD results with good agreements are listed in the ESI (Fig. S8†).
Complex 1 is air-stable and can keep its crystalline integrity at ambient conditions. Thermogravimetric curves of 1-DMF and 1-DMA (Fig. S9†) show the great losses from 100 °C to 280 °C, corresponding to the release of guest molecules (1-DMF, calc. 19.9%, obser. 19.3%; 1-DMA, calc. 22.8%, 22.6%). The structures of 1-DMF and 1-DMA are stable up to 450 °C, and then quickly decompose.
Luminescence and sensing property
The solid-state luminescence of H3cpbda and complex 1 were investigated at room temperature (Fig. S10†). Excitation wavelength of complex 1 is 310 nm, resulting in a fluorescence emission peak at 343 nm. The fluorescence of H3L shows an emission peak at 366 nm (excitation 230 nm). The blue shift of emission peak of 1 should be attributed to the π → π* transitions or the ligand-to-metal charge transfer (LMCT), the incorporation of metal-ligand coordination interaction. After the ligands bond with metal ions, the increased rigidity of complex 1 enhances the intensity of emission peak.
To probe the sensing properties of complex 1 on metal cations and anions, 10 mg of 1-DMF and 1-DMA were introduced into 5 mL of different M(NO3)x (M = Ni2+, Pb2+, Co2+, Cu2+, Cd2+, Zn2+, Ag+, Mn2+, Cr3+, Bi3+, Fe3+, Ca2+, Mg2+, Ba2+, Sr2+, Zr4+, K+, Na+) and Kx(A) (A = Cl−, Br−, I−, CO32−, NO3−, SO42−, ClO3−, PO43−, Cr2O72−, CrO42−, SCN−) solutions, and the emission data were recorded for luminescence studies. The studies of complex 1-DMF (Fig. 3 and Fig. 4) showed that the Ag+, Cr3+ and Fe3+ cations and the Cr2O72−, CrO42− anions can distinctly induce the luminescence quenching. The similar sensing results of complex 1-DMA were obtained, as listed in ESI (Fig. S11 and S12).†
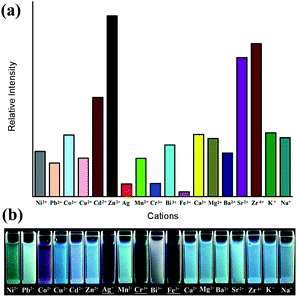 |
| Fig. 3 The luminescence intensities of 1-DMF suspensions with various metal cations. | |
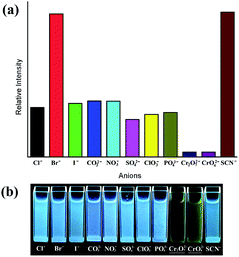 |
| Fig. 4 The luminescence intensities of 1-DMF suspensions with various anions. | |
In order to further detect the sensing sensitivities of complex 1, the titration experiments were conducted. The 1-DMF and 1-DMA suspensions were prepared by dispersing 10 mg complexes into 3 mL DMF/H2O and DMA/H2O (1
:
1). The concentrations of cations (Ag+, Cr3+ and Fe3+) and anions (Cr2O72−, CrO42−) were increased from 0 to 2771 ppm to monitor the emission responses. The analysis results of 1-DMF suspensions are shown in Fig. 5–9. Along with the injections of Ag+ and Cr3+ ions solutions, the luminescence slowly decrease. When the concentration of Ag+ increases to 2308 ppm, the luminescence intensity decreases to 4% of the original one (Fig. 5). It is worse for Cr3+. The luminescence intensity is still 43.1% of the original one when the concentration increases to 2771 ppm (Fig. 6). However, the injections of Fe3+, Cr2O72− and CrO42− show such remarkable quenching effects that the ion concentration of merely 164 ppm can render the luminescence intensities decrease to 4.2%, 6.4% and 8.9% (Fe3+, Cr2O72− and CrO42−) respectively (Fig. 7–9). The titration results of 1-DMA are listed in Fig. S17 and S18,† and the quenching result is: Fe3+ > Cr2O72− > CrO42− ≫ Ag+ > Cr3+. Obviously, complex 1 can sensitively detect the Fe(III) metal cation and Cr(VI) anions (Cr2O72− and CrO42−) by the quenching responses. Generally, there are two reasons for the sensing phenomena, photo induced electron transfer or resonance energy transfer or both.12 Considering the dense 3D structure of the complex 1, the changes of fluorescence intensity may not be attributed to the internal corporation, but the surface interactions and the photo-induced electron transfers between the excited frameworks and the guest molecules is more probable.
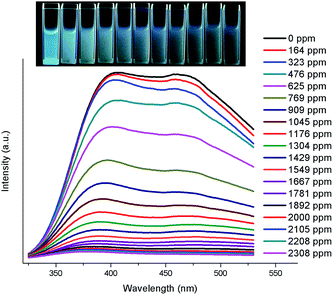 |
| Fig. 5 The luminescence intensities of 1-DMF suspensions with Ag(I) concentration varying from 0 to 2308 ppm. | |
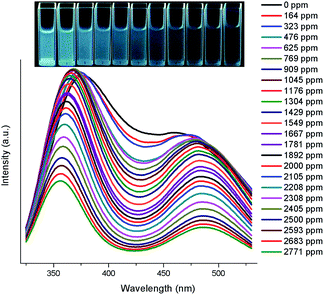 |
| Fig. 6 The luminescence intensities of 1-DMF suspensions with Cr(III) concentration varying from 0 to 2771 ppm. | |
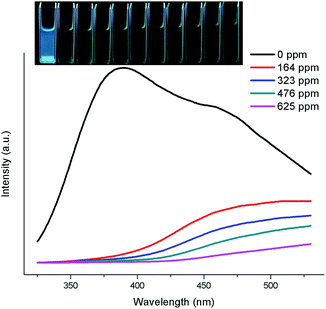 |
| Fig. 7 The luminescence intensities of 1-DMF suspensions with Fe(III) concentration varying from 0 to 1429 ppm. | |
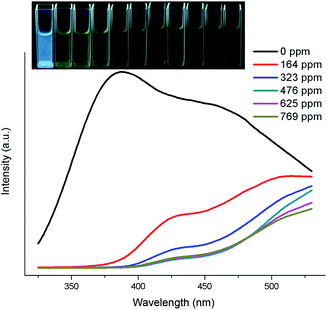 |
| Fig. 8 The luminescence intensities of 1-DMF suspensions with Cr2O72− concentration varying from 0 to 1429. | |
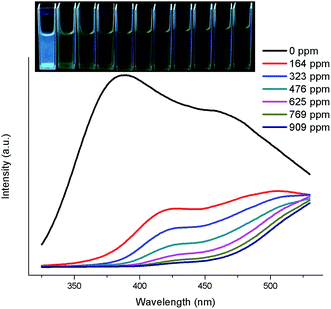 |
| Fig. 9 The luminescence intensities of 1-DMF suspensions with CrO42− concentration varying from 0 to 1429 ppm. | |
Organic dyes adsorptions
The excellent sensing properties of polymers motivate the further investigation interests of the organic dyes adsorption. The 10 mg 1-DMF and 1-DMA complexes were put into seven kinds of aqueous organic dye solutions with concentration of 30 ppm, MG, XO, MGO, CR, AR, MO and CR (Fig. S19†). After two hours, the concentration of MGO decreased to 14.75 ppm. However, the others exhibited no obvious changes (MG is 29.22, XO is 28.16, CR is 29.27, AR is 28.53, MO is 29.00 and CR is 29.32), as shown in Fig. 10. The complex 1 shows a great adsorption of MGO. There are some difficulties in the further demonstration that the complex could selective adsorb MGO dye in a mixed solution. As the Fig. S22 and S23† shown, although the UV spectra of seven separate kinds of dye are different, the mixture of seven dyes (include MGO) and the mixture of six dye (exclude MGO) generate almost the same UV spectra. It is hard to measure and demonstrate whether the MGO dye is been selective absorbed in a mixture. The HPLC-MS was considered to separate the dyes in a mixed solution. However, the suitable mobile phase which can separate the similar seven dyes is difficult to seek. The further explorations on the ways to detect and separate the mixed dyes solutions are crucial.
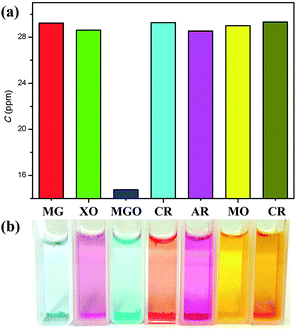 |
| Fig. 10 The (a) concentrations and (b) naked-eye photos of different dyes in 2 hours after the additions of complexes 1-DMF. | |
After the soaking and washing by DMSO, the dyes can be released from the complex. The recycle adsorptions of MGO (30 ppm) are discussed, the complex reveals good stability and repeatability which can recycle absorb the MGO dye more than 13 times. The maximum adsorption capacity is 50.3% (Fig. 11). The detection of MGO concentration over time (Fig. 12) shows that the adsorption process is very fast within 40 minutes. However, compared with the adsorption data of MO dye reported in the ref. 13, the adsorption velocity and quantity of MGO in the present work are still unsatisfactory, which should be attributed to the lower porosity of complex 1.
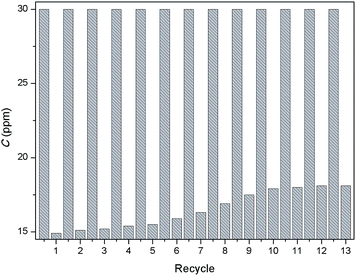 |
| Fig. 11 The recycle adsorptions of Malachite Green Oxalate (MGO) by complex 1-DMF. | |
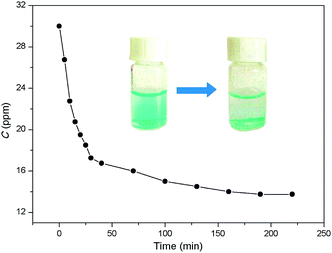 |
| Fig. 12 The MGO concentration changing over time, after the addition of 1-DMF. | |
Conclusion
In summary, the 3D porous coordination polymer 1-DMF (or 1-DMA) based on H3cpbda ligand was synthesized by multiple methods and exhibited good thermal stability. According to its fluorescence property, complex 1 showed the sensitive detections of Fe(III) metal cation and Cr(VI) anions (Cr2O72− and CrO42−) by the quenching responses. In addition, further research revealed the complex 1 demonstrated high selective and recycled adsorption of the Malachite Green Oxalate (MGO) dye. The results indicated that the polymer 1 could be employed as a stable multi-functional material acting as good luminescent sensor and dye absorber.
Acknowledgements
This work is supported by the National Natural Science Foundation of China (Grants 21371142, 21201139, 20931005, 91022004 and 21401121), Natural Science Foundation of Shaanxi Province (Grant 2013JQ2016, 2014JQ2061), Doctor Foundation of Shaanxi University of Science & Technology (BJ14-22).
Notes and references
-
(a) K. Chen, Q. H. Shu and M. Schmittel, Chem. Soc. Rev., 2015, 44, 136 RSC;
(b) P. C. A. Swamy, S. Mukherjee and P. Thilagar, Anal. Chem., 2014, 86, 3616 CrossRef CAS PubMed;
(c) S. Heng, A. M. Mak and D. Stubing, et al., Anal. Chem., 2014, 86, 3268 CrossRef CAS PubMed.
-
(a) R. Matsuda, R. Kitaura, S. Kitagawa, Y. Kubota, R. V. Belosludov, T. C. Kobayashi, H. Sakamoto, T. Chiba, M. Takata, Y. Kawazoe and Y. Mita, Nature, 2005, 436, 238 CrossRef CAS PubMed;
(b) S. Ma, D. Sun, J. M. Simmons, C. D. Collier, D. Yuan and H. C. Zhou, J. Am. Chem. Soc., 2008, 130, 1012 CrossRef CAS PubMed;
(c) S. Horike, M. Dinca, K. Tamaki and J. R. Long, J. Am. Chem. Soc., 2008, 130, 5854 CrossRef CAS PubMed;
(d) S. Yang, X. Lin, A. J. Blake, G. Walker, P. Hubberstey, N. R. Champness and M. Schröder, Nat. Chem., 2009, 1, 487 CrossRef CAS PubMed;
(e) Z. Z. Lu, R. Zhang, Y. Z. Li, Z. J. Guo and H. G. Zheng, J. Am. Chem. Soc., 2011, 133, 4172 CrossRef CAS PubMed.
-
(a) L. Liu, J. H. Hao and C. Hao, et al., RSC Adv., 2015, 5, 3045 RSC;
(b) J. W. Ye, L. M. Zhao and G. L. Ning, et al., Chem.–Eur. J., 2015, 21, 2029 CrossRef CAS PubMed;
(c) M. Carboni, Z. Lin and W. B. Lin, et al., Chem.–Eur. J., 2014, 20, 14965 CrossRef CAS PubMed;
(d) J. S. Qin, S. R. Zhang and Z. M. Su, et al., Chem.–Eur. J., 2014, 20, 5625 CrossRef CAS PubMed;
(e) Y. Yu, X. M. Zhang and Y. B. Dong, et al., Chem. Commun., 2014, 50, 1444 RSC.
-
(a) C. Bazzicalupi, C. Caltagirone and N. Zaccheroni, et al., Chem.–Eur. J., 2013, 19, 14639 CrossRef CAS PubMed;
(b) Y. P. Zhu, T. Y. Ma and Z. Y. Yuan, et al., ACS Appl. Mater. Interfaces, 2014, 6, 16344 CrossRef CAS PubMed;
(c) S. Bhattacharyya, A. Chakraborty and T. K. Maji, et al., Chem. Commun., 2014, 50, 13567 RSC.
-
(a) Z. H. Xiang, C. Q. Fang, S. H. Leng and D. P. Cao, J. Mater. Chem. A, 2014, 2, 7662 RSC;
(b) Y. Lu, B. Yan and J. L. Liu, Chem. Commun., 2014, 50, 9969 RSC;
(c) H. B. Xu, S. H. Zhou and Q. H. Yuan, et al., J. Mater. Chem. C, 2015, 3, 291 RSC.
- S. Sarkar, S. Dutta, P. Bairi and T. Pal, Langmuir, 2014, 30, 7833 CrossRef CAS PubMed.
-
(a) S. Han, Y. Wei, C. Valente, I. Lagzi, J. J. Gassensmith, A. Coskun, J. F. Stoddart and B. A. Grzybowski, J. Am. Chem. Soc., 2010, 132, 16358 CrossRef CAS PubMed;
(b) C. Zou, Z. Zhang, X. Xu, Q. Gong, J. Li and C. D. Wu, J. Am. Chem. Soc., 2012, 134, 87 CrossRef CAS PubMed;
(c) R. A. Smaldone, R. S. Forgan, H. Furukawa, J. J. Gassensmith, A. M. Z. Slawin, O. M. Yaghi and J. F. Stoddart, Angew. Chem., Int. Ed., 2010, 49, 8630 CrossRef CAS PubMed;
(d) L. Li, F. Sun, J. Jia, T. Borjigin and G. Zhu, CrystEngComm, 2013, 15, 4094 RSC.
- J. H. Cui, Y. Z. Li, Z. J. Guo and H.-G. Zheng, Chem. Commun., 2013, 49, 555 RSC.
-
(a) W. H. Huang, G. P. Yang and Y. Y. Wang, et al., Cryst. Growth Des., 2013, 13, 66 CrossRef CAS;
(b) W. H. Huang, X. J. Luan and Y. Y. Wang, et al., CrystEngComm, 2013, 15, 10389 RSC;
(c) W. H. Huang, Y. Y. Wang and Y. N. Zhang, et al., Inorg. Chim. Acta, 2015, 433, 52 CrossRef CAS.
- G. M. Sheldrick, SHELXL-97, Program for Crystal Structure Determination, University of Göttingen, Germany, 1997 Search PubMed.
- G. M. Sheldrick, SHELXL-97, Program for Crystal Structure Refinement, University of Göttingen, Germany, 1997 Search PubMed.
-
(a) A. J. Lan, K. H. Li and H. H. Wu, et al., Angew. Chem., Int. Ed., 2009, 48, 2334 CrossRef CAS PubMed;
(b) S. Pramanik, C. Zheng and X. Zhang, et al., J. Am. Chem. Soc., 2011, 133, 4153 CrossRef CAS PubMed;
(c) S. S. Nagarkar, B. Joarder and A. K. Chaudhari, et al., Angew. Chem., Int. Ed., 2013, 52, 2881 CrossRef CAS PubMed;
(d) S. J. Toal and W. C. Trogler, J. Mater. Chem., 2006, 16, 2871 RSC;
(e) J. C. Sanchez, A. G. DiPasquale, A. L. Rheingold and W. C. Trogler, Chem. Mater., 2007, 19, 6459 CrossRef CAS.
- Y. X. Tan, Y. P. He, M. Wang and J. Zhang, RSC Adv., 2014, 4, 1480 RSC.
Footnote |
† Electronic supplementary information (ESI) available: Synthesis, crystallographic details, additional figures and tables. CCDC 888821. For ESI and crystallographic data in CIF or other electronic format see DOI: 10.1039/c5ra20015f |
|
This journal is © The Royal Society of Chemistry 2015 |