DOI:
10.1039/C5RA17819C
(Paper)
RSC Adv., 2015,
5, 97121-97126
Triphenylphosphine-assisted highly sensitive fluorescent chemosensor for ratiometric detection of palladium in solution and living cells†
Received
2nd September 2015
, Accepted 30th October 2015
First published on 30th October 2015
Abstract
Accurate and sensitive detection of palladium (Pd) is essential for both environmental and human health applications. This paper describes a triphenylphosphine (PPh3)-assisted highly sensitive fluorescent chemosensor for ratiometric detection of Pd2+ concentrations up to 300 nM with an LOD of 1 nM. During the detection, PPh3 plays a crucial role in improving the sensitivity of this chemosensor. The chemosensor also has suitable water-solubility which allows detection of Pd2+ in solution. Our studies show that it exhibits excellent selectivity toward Pd2+ and undergoes a color change from colorless to yellow to allow visual detection of Pd2+. Besides detection in solution, this chemosensor also shows great potential as an imaging reagent to detect Pd2+ as low as 0.03 ppm in diverse cells.
1. Introduction
Palladium (Pd) detection has received great attention in chemical and biological studies due to the fact that it is a critical material in a variety of industrial applications; however, it brings potential harm to the environment and human health. In industry, Pd-derived compounds form efficient universal catalysts,1–3 which are used on a large scale to promote numerous chemical conversions in the production of fine chemicals, synthetic drugs and polymers.4,5 Pd metal is an indispensable material in many areas such as automobile catalytic converters, electronics technology, hydrogen storage, dentistry, jewelry and photography.6,7 However, Pd-containing substances released from industry can cause serious problems for environment and human health.8 Current studies indicate Pd can be absorbed by biological materials, and accumulated in the food chain. Once human eats food containing Pd, the heavy metal enters the human body and binds to thiol-containing amino acids, proteins, DNA or other macromolecules.9,10 The binding to these biological moieties can disturb various physiological processes inside the human body. According to the governmental restrictions, the dietary Pd intake should not exceed than 1.5–15 μg per day per person and the Pd threshold in drugs is 5–10 ppm.11 In order to have systems help detecting and monitoring the Pd levels, sensitive and accurate method for Pd detection is in great need.
Known for its high sensitivity and specificity, operational simplicity and non-invasive detection, fluorescent chemosensor is particularly preferable for the quantitative or quantitative analysis for environmental and biological samples.12–15 Using Pd's specific coordination or catalysis reactions as recognition mechanisms,16 a large number of fluorescent chemosensors have been reported to detect Pd2+,17–29 which is the most abundant existing form of the palladium metal under physiological conditions or in environment. Some of these chemosensors are suitable for measuring Pd2+ in biological system.30–34 However, most of these chemosensors for quantitatively measuring Pd2+ concentration are based on fluorescent fluctuation in a single emission channel. The drawback for utilizing single emission channel is that it can bring in uncertainties in detection due to different factors such as variability in chemosensor distribution, instability in the excitation source and effects of the microenvironment.35–37 In contrast, ratiometric chemosensors can help eliminate some of these uncertainties by introducing the self-calibration mechanism using two emission channels. Though a small number of ratiometric fluorescent chemosensors have been developed, some of them have insufficient soluble in an aqueous medium.38–41 Additionally, we also noticed among these studies, a high concentration (≥20 μM) of Pd2+ are usually used to be incubated with living cells to test the performance of these chemosensor in cellular environment.30,32–34,42,43 However, the results drawn from these experiments may not directly prove the chemosensor performance in the detection of biological samples containing low levels (e.g. nanomolar level) of Pd2+.
To address these issues, we previously designed a 4-hydroxynaphthalimide-derived ratiometric fluorescent chemodosimeter for imaging palladium in living cells,43 shown in Scheme 1. Based on the excellent internal charge transfer (ICT) structure of 4-hydroxynaphthalimide fluorophore,44,45 the fluorescence wavelength of the chemodosimeter presented a 73 nm redshift after Pd-catalyzed cleavage of propargyl ethers owing to the stronger electron-donating ability of oxygen anion.46–48 The chemosensor displayed an appropriate solubility in PBS aqueous solution and high selectivity for Pd detection via measuring fluorescence intensity ratios between the two emission channels (500 nm/558 nm). However, even though the chemodosimeter with a detection limit of 70 nM presented excellent sensitivity for Pd detection in vitro, a high level of Pd2+ (40 μM) was still needed to be incubated with cells to perform intracellular imaging experiment. A number of the ratiometric chemosensors that has been reported recently also suffered from the similar problem in high level of Pd2+ for incubation.30,32–34,42,43 These studies indicated that intracellular complex testing conditions might affect the chemosensor sensitivity for Pd2+ detection. As a continuation of our previous work, we are dedicated to improve the chemosensor sensitivity for intracellular Pd2+ detection. In this work, we developed a high sensitive fluorescent chemosensors 1 for intracellular Pd2+ imaging based on triphenylphosphine (PPh3)-supported Pd-catalyzed deallylation reaction (Scheme 1). For the similar chemical structures, chemosensor 1 still maintains the advantages of our previous chemodosimeter including proper aqueous solubility and dual-channel fluorescence emission. However, the detection limit in vitro decreased from 70 nM to 1 nM and the Pd2+ concentration used in cell incubation was reduced from 40 μM to 300 nM. The analytical performance of the two chemosensors was compared in Table 1 in ESI.† To account for these difference, we found that PPh3 play a crucial role in improving the sensitivity of chemosensor 1 for Pd2+ detection in solution and living cells.
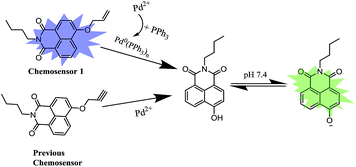 |
| Scheme 1 Proposed recognition mechanisms of chemosensor 1 and previous chemosensor toward Pd2+. | |
2. Materials and methods
2.1 Materials
The N-butyl-4-chloro-1,8-naphthalimide was synthesized according to our previous work.49 All other materials were purchased from commercial suppliers and used without further purification. Silica gel (200–300 mesh, Qingdao Haiyang Chemical Co.) was used for column chromatography. 1H NMR spectra were acquired on a Bruker AMX400 spectrometer. Chemical shifts (δ) were reported in ppm relative to a Me4Si standard. Electrospray ionization (ESI) mass spectra were measured with an LC-MS 2010A (Shimadzu) instrument. Fluorescence measurements were obtained on a Hitachi F-2500 fluorescence spectrometer with a 10 mm quartz cuvette. The pH measurements were made with a Sartorius basic pH-meter PB-10. Confocal imaging was performed in Olympus FV1000-LX81. HPLC was performed on a TechMate C18-ST (5 μM, 4.6 × 250 mm) column (TechMate Technology CO., LTD.) using an HPLC system composed of two pumps (LC-6AD, SHIMADZU) and a detector (SPD-20AV, SHIMADZU).
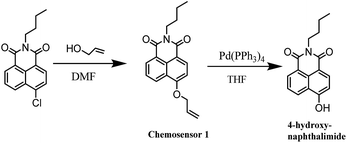 |
| Scheme 2 The synthesis of chemosensor 1 and its Pd0-catalyzed deallylation product. | |
2.2 Synthetic procedures
Chemosensor 1. To a mixture of N-butyl-4-chloro-1,8-naphthalimide (863.2 mg, 3 mmol), K2CO3 (1.3 g, 10.0 mmol) and 18-crown-6 (30.0 mg, 0.1 mmol) in 10 mL DMF was added allyl alcohol (900.0 mg, 15 mmol). The resulting solution was heated to 100 °C for 12 h. After cooling to room temperature, the reaction mixture was dropped into 200 mL doubly distilled water. The product was exacted from water using CHCl3 as solvent. After removal of CHCl3, the residues were purified by silica gel column chromatography using CHCl3/petroleum ether (v/v, 1
:
10) as eluent to afford 1 as a pale yellow solid (431 mg, 46%) (Scheme 2). 1H-NMR (400 MHz, CDCl3-d6) δ (*10−6): 0.98 (t, J = 7.3 Hz, 3H), 1.42–1.48 (m, 2H), 1.68–1.75 (m, 2H), 4.17 (t, J = 7.5 Hz, 2H), 4.85 (d, J = 5.0 Hz, 2H), 5.42 (d, J = 10.5 Hz, 1H), 5.55 (d, J = 17.2 Hz, 1H), 6.14–6.21 (m, 1H), 7.03 (d, J = 8.2 Hz, 1H), 7.26 (s, 1H), 7.70 (t, J = 7.8 Hz, 1H), 8.53 (d, J = 8.2 Hz, 2H), 8.60 (d, J = 8.0 Hz, 2H). 13C-NMR (100 MHz, CDCl3-d6): δ (*10−6): 13.99, 20.56, 30.44, 40.25, 69.73, 106.39, 115.47, 118.85, 122.71, 123.81, 126.08, 128.77, 129.64, 131.68, 132.05, 133.40, 159.80, 164.09, 164.68. ESI-MS calcd for C19H19NO3 [M + Na]+ 332.1, found 332.1.
The reaction product of chemosensor 1 with Pd(PPh3)4. To a solution of chemosensor 1 (620 mg, 2.00 mmol) in THF (20 mL) was added morpholine (195 mg, 2.20 mmol), sodium borohydride (90.8 mg, 2.40 mmol) and Pd(PPh3)4 (11.6 mg, 0.01 mmol) at 24 °C. After reaction for 4 h, 3 N HCl (4 mL) was added very slowly to quench the reaction. Then, the solution was extracted with EtOAc, and the organic phase was washed with brine, dried over Na2SO4, then filtered and the solvent was evaporated. The residue was purified by silica gel flash chromatography (CHCl3/petroleum ether, v/v, 1
:
50) to afford compound 2 as a yellow solid (500 mg, 90%). 1H-NMR (400 MHz, DMSO-d6) δ (*10−6): 0.85 (t, J = 6.8 Hz, 3H), 1.23–1.39 (m, 2H), 1.56–1.64 (m, 2H), 4.03 (t, J = 7.4 Hz, 2H), 7.16 (d, J = 8.0 Hz, 1H), 7.75–7.79 (m, 1H), 8.35 (d, J = 1.2 Hz, 1H), 8.37 (d, J = 1.2 Hz, 1H), 8.48 (d, J = 7.6 Hz, 1H), 8.54 (d, J = 8.4 Hz, 1H), 11.85 (s, 1H). 13C-NMR (100 MHz, DMSO-d6): δ (*10−6): 14.12, 20.25, 30.16, 110.31, 113.00, 122.16, 122.73, 125.87, 129.19, 129.51, 131.40, 133.83, 160.60, 163.35, 164.01. HRMS (ESI positive) calcd for C16H15NO3 [M + H]+ 270.11247, found 270.11240.
2.3 Cell culture and imaging
HepG2 cells and RAW 264.7 macrophage cells (gifted from the center of cells, Institute of Basic Medical Sciences, Chinese Academy of Medical Sciences) were cultured in culture media (DMEM/F12) supplemented with 10% FBS, 1.5 g L−1 sodium bicarbonate, 1% antibiotics (penicillin/streptomycin, 100 U mL−1) at 37 °C under a humidified atmosphere containing 5% CO2. Before use, HepG2 cells were seeded in a 6-well plate at a density of 104 cells per well in culture media. For detecting Pd2+, the cells were incubated with the chemosensor 1 (5 μM) and PPh3 (4 μM) for 15 min, washed twice with culture media, and observed under confocal fluorescence microscope. Then, the cells were incubated with Pd2+ (final concentration: 300 nM) for 15 min and were measured the fluorescence changes. RAW 264.7 cells were treated with the same procedures as HepG2 cells.
3. Results and discussion
3.1 Spectral response of chemosensor 1 to Pd2+ in PPh3-reducing environment
First, we assessed the catalyzed deallylation reaction between chemosensor 1 and Pd2+ in PPh3-reducing environment. Referring to the previous literatures,50,51 Pd2+ can be reduced to Pd0(PPh3)n (n = 1–4) in situ by using PPh3 as a reducing agent and ligand, shown in Scheme 1. The generated Pd0(PPh3)n catalyzes the allylic oxidative insertion to cleave the allylic C–O bond in chemosensor structures, accompanied by changes in fluorescence signal. Under simulated physiological conditions (PBS solution, 10 mM, pH 7.4), the solution containing chemosensor 1 (5 μM) and PPh3 (4 μM) exhibited absorption and emission band centered at 375 nm and 460 nm, respectively (Fig. 1). Upon an addition of Pd2+ (0.5 μM), the maximum absorption peak showed a 75 nm redshift to 450 nm, with the solution color changed from colorless to yellow (Fig. 1A). Meanwhile, the maximum emission peak underwent a 100 nm redshift to 560 nm (Fig. 1B). These results confirmed that chemosensor 1 could serve as a naked-eye and ratiometric indicator for Pd2+ detection in solution.
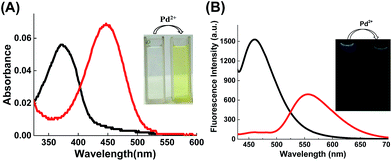 |
| Fig. 1 (A) Absorption spectra and (B) fluorescence spectra of chemosensor 1 (5 μM, containing 4 μM PPh3) before and after addition of Pd2+ (0.5 μM), measured in PBS buffer (10 mM, pH 7.4). The blank line is for chemosensor 1 and the red line is for chemosensor 1 + Pd2+. Inserts show the changes of the color and fluorescence of the solution before and after addition of Pd2+. Each spectrum was acquired after Pd2+ addition for 1 h at room temperature. λex = 410 nm. | |
To confirm the mechanism of chemosensor 1 in sensing Pd2+, the reaction process was monitored by high phase liquid chromatography (HPLC). As shown in Fig. S1,† in PBS solution (pH = 7.4), chemosensor 1 can converted into 4-hydroxy-naphthalimide in the presence of PdCl2 and PPh3 (Fig. S1C†). However, in THF medium (see synthetic procedures), the deallylation reaction can be hardly performed in the presence of Pd(PPh3)4 (Fig. S1A†). To enhance turnover, NaBH4 was chosen as a reducing agent because of its known ability to efficiently reduce Pd2+ to Pd0.50 The result showed that NaBH4 significantly enhanced the Pd-catalyzed deallylation of chemosensor 1 in THF medium (Fig. S1B†).
3.2 The effect of PPh3 on the recognition reaction
Next, we examined the effect of PPh3 on the recognition reaction. As seen in Fig. 2, the fluorescence spectral of chemosensor 1 (5 μM) presented limited change in the presence of Pd2+ (0.5 μM), indicating the deallylation reaction is hardly performed under Pd2+ catalysis. Chemosensor 1 showed almost the same fluorescence spectra in the absence and presence of PPh3 (4 μM), confirming that PPh3 itself does not affect the chemosensor emission spectra. Additionally, PPh3 and Pd(PPh3)4 show no fluorescence emission in detection wavelength range. The fluorescence spectral only underwent a large redshift in both presence of PPh3 and Pd2+. These results revealed that PPh3 plays a crucial role in recognition reaction. According to the previous study by Koide's group,50,51 Pd2+ can be converted to Pd0(PPh3)n in PPh3-reducing environment. As a result, we achieved the same conversion in the chemosensor spectral when Pd(PPh3)4 was used in lieu of PdCl2 and PPh3 (Fig. 2). Moreover, in PPh3-reducing environment, an optimal time for the reaction between chemosensor 1 and Pd2+ was between 60 to 90 min (Fig. S2†).
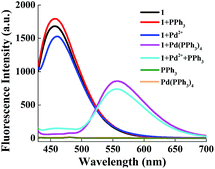 |
| Fig. 2 Fluorescence response of chemosensor 1 (5 μM) toward PPh3 (4 μM), Pd2+ (0.5 μM), Pd(PPh3)4 (0.5 μM), PPh3 (4 μM) + Pd2+ (0.5 μM), respectively, and the fluorescence spectral of PPh3 (4 μM) and Pd(PPh3)4 (0.5 μM) in wavelength range of 430–700 nm. All experiments were performed in PBS buffer (10 mM, pH 7.4). λex = 410 nm. | |
3.3 Sensitivity of chemosensor 1 toward Pd2+
Chemosensor 1 showed high sensitivity for ratiometric detection of Pd2+ in PBS solution (10 mM, pH 7.4). As seen in Fig. 3, upon an increasing addition of Pd2+ (final concentration: 0–250 nM), the solution containing chemosensor 1 (5 μM) and PPh3 (4 μM) presented a gradual decrease in fluorescent intensity at 460 nm, with a corresponding increase at 560 nm. In addition, a well-defined isoemission point at around 517 nm was also observed, indicating that a new species was formed. The logarithm of the fluorescence intensity ratio at two emission peaks, log(F560/F460), changed from −1.1 to 0.31 after addition of Pd2+ (250 nM). There was a good linearity (R2 = 0.990) between log(F560/F460) and Pd2+ concentrations in the range of 20–250 nM (Fig. 2). The detection limit of chemosensor 1 is 1 nM (see ESI†), to our knowledge, this value is the lowest in reported ratiometric chemosensors for measuring Pd2+.
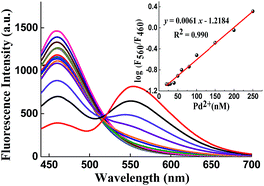 |
| Fig. 3 Fluorescence responses of chemosensor 1 (5 μM, containing 4 μM PPh3) toward Pd2+ (final concentration: 0, 4, 8, 10, 15, 20, 25, 30, 40, 50, 60, 80, 100, 150, 200, 250 nM). Insert: the linear relationship between log(F560/F460) and Pd2+ concentrations. All experiments were performed after reacting with metal ions for 1 h in PBS solution (10 mM, pH = 7.4). λex = 410 nm. | |
3.4 Selectivity of chemosensor 1 toward Pd2+
The selectivity of chemosensor 1 toward various metal ions was also investigated. Under the same conditions (10 mM PBS, pH 7.4), chemosensor 1 exhibited no apparent fluorescence change in the presence of other metal ions, even other π-philic metals such as Ag, Ni, Au, Co, Hg (Fig. 4A). Moreover, the interference of the above-mentioned metal ions on monitoring Pd were also studied (Fig. 4B). These results showed chemosensor 1 possesses high selectivity toward Pd2+ even in the presence of other metal ions.
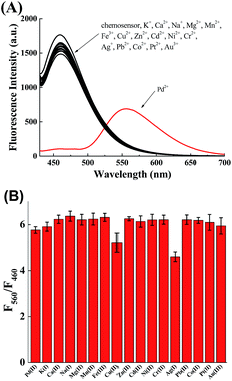 |
| Fig. 4 (A) Fluorescence responses of chemosensor 1 (5 μM, containing 4 μM PPh3) toward various metal ions (0.5 μM for Pd2+, 3 μM for other ions). (B) Fluorescence responses of chemosensor 1 (5 μM, containing 4 μM PPh3) toward Pd2+ (0.5 μM) in the presence of various metal ions (3 μM for other ions). All experiments were performed after reacting with metal ions for 1 h in PBS solution (10 mM, pH = 7.4). λex = 410 nm. | |
3.5 Ratiometric imaging of Pd2+ in living cells
To further demonstrate the practical application of chemosensor 1 in living systems, the experiments were carried out in HepG2 and RAW 264.7 macrophage cells. As seen in Fig. 5, the cells incubated with chemosensor 1 (5 μM) and PPh3 (4 μM) for 15 min showed an intense intracellular fluorescence, indicating that chemosensor 1 could penetrate cell membranes. Then, upon addition of Pd2+ (300 nM, equivalent to 0.03 ppm), the fluorescence intensity ratios at two emission channels increased immediately (Fig. 5c). These results demonstrate that the cellular uptake of Pd2+ as low as 0.03 ppm, which is substantially lower than the specified threshold in drugs (5–10 ppm), could be determined by using chemosensor 1 as an imaging reagent. Moreover, the RAW 264.7 cells showed a similar phenomenon in dual emission channels under stimulation of Pd2+ (Fig. 6), indicated that chemosensor 1 can be used for Pd2+ detection in diverse cells.
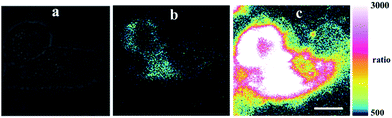 |
| Fig. 5 Ratiometric imaging of Pd2+ in living HepG2 cells by using confocal fluorescence microscope. The cells were incubated with chemosensor 1 (5 μM) and PPh3 (4 μM) for 15 min: (a) the bright-field transmission image; (b) the ratio images generated from green channel at 515 ± 30 nm and blue channel at 450 ± 35 nm. Then, the cells were incubated with Pd2+ (300 nM) for 15 min: (c) the ratio images generated from green channel at 515 ± 30 nm and blue channel at 450 ± 35 nm. Incubation was performed at 37 °C under a humidified atmosphere containing 5% CO2. Scale bar = 10 μm. | |
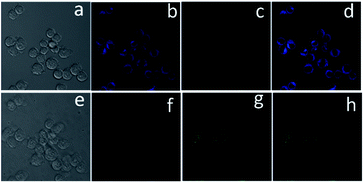 |
| Fig. 6 Fluorescence imaging of Pd2+ in RAW 264.7 macrophage cells. The cells were incubated with chemosensor 1 (5 μM) and PPh3 (4 μM) for 15 min: (a) bright-field image, (b) blue channel at 450 ± 35 nm, (c) green channel at 515 ± 30 nm, and (d) mixed image generated from (c) and (b). Then, the cells were incubated with Pd2+ (300 nM) for 15 min: (e) bright-field image, (f) blue channel at 450 ± 35 nm, (g) green channel at 515 ± 30 nm, and (h) mixed image generated from (f) and (g). Incubation was performed at 37 °C under a humidified atmosphere containing 5% CO2. | |
To estimate the cytotoxicity of chemosensor 1, we performed 3-(4,5-dimethylthiazol-2-yl)-2,5-diphenyltetrazolium bromide (MTT) assays in RAW 264.7 cells with 5, 10 and 20 μM chemosensor 1 (containing 4 μM PPh3) for 24 h, respectively. The experimental results in Fig. S3,† showed that our probe exhibited very low toxicity to cultured cells at a concentration of 5 μM in the experimental conditions.
4. Conclusions
In conclusion, we have developed a highly sensitive 4-hydroxynaphthalimide-derived fluorescent chemosensor 1 for ratiometric detection of Pd2+ in PPh3-reducing environment. Chemosensor 1 can detect Pd2+ quantitatively by ratiometric fluorescence method with a 100 nm red-shifted emission wavelength in the concentration up to 300 nM with a detection limit of 1 nM. During the detection, PPh3 play a crucial role in improving the chemosensor sensitivity. The chemosensor also has suitable water-solubility, exhibits excellent selectivity toward Pd2+, and undergoes a color change from colorless to yellow for “naked eye” detection of Pd2+. Finally, we demonstrated that chemosensor 1 can be used as an imaging reagent to detect Pd2+ as low as 0.03 ppm in diverse cells.
Acknowledgements
This work was financially supported by the National Nature Science Foundation of China (No. 21275018, 21575015 and 21203008), Beijing National Laboratory for Molecular Sciences (BNLMS) (20140121), Research Fund for the Doctoral Program of Higher Education of China (RFDP) (No. 20121101110049) and the 111 Project (B07012) for financial support.
References
- C. Xu, L. Deiana, S. Afewerki, C. Incerti-Pradillos, O. Cordova, P. Guo, A. Cordova and N. Hedin, Adv. Synth. Catal., 2015, 357, 2150–2156 CrossRef CAS.
- X. Wu, H. Neumann and M. Beller, Chem. Rev., 2013, 113, 1–35 CrossRef CAS PubMed.
- G. Zeni and R. C. Larock, Chem. Rev., 2004, 104, 2285–2309 CrossRef CAS PubMed.
- S. L. Buchwald, C. Mauger, G. Mignani and U. Scholz, Adv. Synth. Catal., 2006, 348, 23–39 CrossRef CAS.
- C. E. Garrett and K. Prasad, Adv. Synth. Catal., 2004, 346, 889–900 CrossRef CAS.
- R. Rushforth, Platinum Met. Rev., 2004, 48, 30–31 CAS.
- W. Grochala and P. P. Edwards, Chem. Rev., 2004, 104, 1283–1315 CrossRef CAS PubMed.
- S. Zimmermann, F. Alt, J. Messerschmidt, A. von Bohlen, H. Taraschewski and B. Sures, Environ. Toxicol. Chem., 2002, 21, 2713–2718 CrossRef PubMed.
- International Program on Chemical Safety, Palladium, Environmental Health Criteria Series 226, World Health Organization, Geneva, 2002 Search PubMed.
- J. Kielhorn, C. Melber, D. Keller and I. Mangelsdorf, Int. J. Hyg. Environ. Health, 2002, 205, 417–432 CrossRef CAS PubMed.
- C. E. Garrett and K. Prasad, Adv. Synth. Catal., 2004, 346, 889–900 CrossRef CAS.
- Z. Guo, S. Park, J. Yoon and I. Shin, Chem. Soc. Rev., 2014, 43, 16–29 RSC.
- X. Li, X. Gao, W. Shi and H. Ma, Chem. Rev., 2014, 114, 590–659 CrossRef CAS PubMed.
- H. N. Kim, Z. Guo, W. Zhu, J. Yoon and H. Tian, Chem. Soc. Rev., 2011, 40, 79–93 RSC.
- Y. Sun, J. Liu, X. Lv, Y. Liu, Y. Zhao and W. Guo, Angew. Chem., Int. Ed., 2012, 51, 7634–7636 CrossRef CAS PubMed.
- H. Li, J. Fan and X. Peng, Chem. Soc. Rev., 2013, 42, 7943–7962 RSC.
- M. Wang, X. Liu, H. Lu, H. Wang and Z. Qin, ACS Appl. Mater. Interfaces, 2015, 7, 1284–1289 CAS.
- A. K. Mahapatra, S. K. Manna, K. Maiti, S. Mondal, R. Maji, D. Mandal, S. Mandal, M. R. Uddin, S. Goswami, C. K. Quah and H. Fun, Analyst, 2015, 140, 1229–1236 RSC.
- R. Zhang, M. Gao, S. Bai and B. Liu, J. Mater. Chem. B, 2015, 3, 1590–1596 RSC.
- W. R. Kitley, P. J. Santa Maria, R. A. Cloyd and L. M. Wysocki, Chem. Commun., 2015, 51, 8520–8523 RSC.
- J. Cui, D. Li, S. Shen, J. Liu and B. Zhao, RSC Adv., 2015, 5, 3875–3880 RSC.
- H. Wang, Y. Lang, H. Wang, J. Lou, H. Guo and X. Li, Tetrahedron, 2014, 70, 1997–2002 CrossRef CAS.
- D. Keum, S. Kim and Y. Kim, Chem. Commun., 2014, 50, 1268–1270 RSC.
- A. Kumar, M. Chhatwal, A. K. Singh, V. Singh and M. Trivedi, Chem. Commun., 2014, 50, 8488 RSC.
- Z. Wang, S. Zheng, J. Cai, P. Wang, J. Feng, X. Yang, L. Zhang, M. Ji, F. Wu, N. He and N. Wan, Anal. Chem., 2013, 85, 11602–11609 CrossRef CAS PubMed.
- J. M. Williams and K. Koide, Ind. Eng. Chem. Res., 2013, 52, 8612–8615 CrossRef CAS.
- J. He, M. Zha, J. Cui, M. Zeller, A. D. Hunter, S. Yiu, S. Lee and Z. Xu, J. Am. Chem. Soc., 2013, 135, 7807–7810 CrossRef CAS PubMed.
- H. Li, J. Fan, J. Du, K. Guo, S. Sun, X. Liu and X. Peng, Chem. Commun., 2010, 46, 1079–1081 RSC.
- L. Duan, Y. Xu and X. Qian, Chem. Commun., 2008, 6339 RSC.
- H. Cui, H. Chen, Y. Pan and W. Lin, Sens. Actuators, B, 2015, 219, 232–237 CrossRef CAS.
- U. Reddy G, F. Ali, N. Taye, S. Chattopadhyay and A. Das, Chem. Commun., 2015, 51, 3649–3652 RSC.
- M. Yang, Y. Bai, W. Meng, Z. Cheng, N. Su and B. Yang, Inorg. Chem. Commun., 2014, 46, 310–314 CrossRef CAS.
- J. Hou, K. Li, K. Yu, M. Ao, X. Wang and X. Yu, Analyst, 2013, 138, 6632 RSC.
- M. Santra, S. Ko, I. Shin and K. H. Ahn, Chem. Commun., 2010, 46, 3964 RSC.
- L. Yuan, W. Lin, K. Zheng and S. Zhu, Acc. Chem. Res., 2013, 46, 1462–1473 CrossRef CAS PubMed.
- W. Niu, L. Fan, M. Nan, Z. Li, D. Lu, M. S. Wong, S. Shuang and C. Dong, Anal. Chem., 2015, 87, 2788–2793 CrossRef CAS PubMed.
- Y. Han, C. Ding, J. Zhou and Y. Tian, Anal. Chem., 2015, 87, 5333–5339 CrossRef CAS PubMed.
- X. Chen, H. Li, L. Jin and B. Yin, Tetrahedron Lett., 2014, 55, 2537–2540 CrossRef CAS.
- X. Wang, Z. Guo, S. Zhu, H. Tian and W. Zhu, Chem. Commun., 2014, 50, 13525–13528 RSC.
- S. Sun, B. Qiao, N. Jiang, J. Wang, S. Zhang and X. Peng, Org. Lett., 2014, 16, 1132–1135 CrossRef CAS PubMed.
- J. Jiang, H. Jiang, W. Liu, X. Tang, X. Zhou, W. Liu and R. Liu, Org. Lett., 2011, 13, 4922–4925 CrossRef CAS PubMed.
- W. Liu, J. Jiang, C. Chen, X. Tang, J. Shi, P. Zhang, K. Zhang, Z. Li, W. Dou, L. Yang and W. Liu, Inorg. Chem., 2014, 53, 12590–12594 CrossRef CAS PubMed.
- B. Zhu, C. Gao, Y. Zhao, C. Liu, Y. Li, Q. Wei, Z. Ma, B. Du and X. Zhang, Chem. Commun., 2011, 47, 8656–8658 RSC.
- R. M. Duke, E. B. Veale, F. M. Pfeffer, P. E. Kruger and T. Gunnlaugsson, Chem. Soc. Rev., 2010, 39, 3936–3953 RSC.
- P. Jin, C. Jiao, Z. Guo, Y. He, S. Zhu, H. Tian and W. Zhu, Chem. Sci., 2014, 5, 4012–4016 RSC.
- B. Zhu, B. Guo, Y. Zhao, B. Zhang and B. Du, Biosens. Bioelectron., 2014, 55, 72–75 CrossRef CAS PubMed.
- C. Liu, H. Wu, Z. Wang, C. Shao, B. Zhu and X. Zhang, Chem. Commun., 2014, 50, 6013–6016 RSC.
- F. Song, S. Watanabe, P. E. Floreancig and K. Koide, J. Am. Chem. Soc., 2008, 130, 16460–16461 CrossRef CAS PubMed.
- S. Zhang, J. Geng, W. Yang and X. Zhang, RSC Adv., 2014, 4, 12596–12600 RSC.
- A. L. Garner, F. Song and K. Koide, J. Am. Chem. Soc., 2009, 131, 5163–5171 CrossRef CAS PubMed.
- F. Song, A. L. Garner and K. Koide, J. Am. Chem. Soc., 2007, 129, 12354–12355 CrossRef CAS PubMed.
Footnote |
† Electronic supplementary information (ESI) available. See DOI: 10.1039/c5ra17819c |
|
This journal is © The Royal Society of Chemistry 2015 |