DOI:
10.1039/C5RA19465B
(Paper)
RSC Adv., 2015,
5, 84872-84878
Mechanism of sodium tripolyphosphate inhibiting the syneresis of HPAM hydrogel
Received
21st September 2015
, Accepted 24th September 2015
First published on 28th September 2015
Abstract
Experimental investigations have been conducted to elucidate the mechanism of sodium tripolyphosphate (STPP) inhibiting hydrogel syneresis with respect to the reaction between STPP and partly hydrolyzed polyacrylamide (HPAM). Viscosity measurements indicate that STPP can increase the viscosity of HPAM. The results of light scattering measurements show that STPP can improve the hydrophilicity of HPAM since both hydrodynamic radius (Rh) and second virial coefficient (A2) of HPAM are increased by STPP, and STPP can also increase the weight average molecular weight (Mw) of HPAM. FTIR and NMR measurements show that the mechanism of the above effect of STPP on HPAM is the following: STPP molecule can react with the C–O in the –COO− group of HPAM whereby the new bond of C–O–P is formed, so a large quantity of STPP molecules “adsorb” on the molecular chains of HPAM and produce the intramolecular or intermolecular crosslinking between HAPM and STPP. As a result, the increase of the hydrophilicity and stability of the crosslinked HPAM improve the water-holding capacity of the hydrogel, and then the hydrogel syneresis is decreased. On the basis of the crosslinking reaction between STPP and HPAM, a hydrogel, which can be successfully used at 160 °C, has been developed with HPAM and Cr3+ for the first time. Therefore, STPP is an effective syneresis inhibitor for the hydrogel formulated with HPAM, and it deserves to be popularization and applied in enhancing hydrogel stability.
Introduction
It is well-known that at the end of the step of primary oil recovery, which is accomplished by exploiting the natural energy stored in the oil reservoir, or by means of mechanical energy, a large amount of oil still remains in the oil reservoir. Therefore, in order to increase the amount of oil which can be extracted, techniques of secondary recovery are customarily used. Secondary recovery techniques is essentially to inject the water or polymer solution into the oil reservoir, which performs the task of conveying the oil to the production well.1–3 Unfortunately, owing to the lack of homogeneity in the oil-bearing rock, which is constituted by zones with different permeability characteristics, such fluids tend to preferentially flow through higher-permeability zones. On the contrary, the lower-permeability zones remain non-fluxed or are only partially fluxed, and such a drawback prevents the oil contained in the lower-permeability zones from being further extracted.4
Polymer hydrogels are generally employed to solve such a problem. This technology uses a flowable mixture of polymer, crosslinker and water. This mixture of relatively low viscosity is injected into the reservoir. Then, with time and temperature, it forms a physical barrier occluding the high-permeability zones, so the fluid injected into the oil field at a later time can be diverted to flow through the low-permeability zones.5–7 However, hydrogels typically used in profile modification applications share a common phenomenon, termed syneresis, in which the solvent phase separates from the hydrogel phase as a result of hydrogel shrinkage. The rate of this separation determines the lifetime of the hydrogel under any given set of circumstances. As such, syneresis is the main reason why the plugging effectiveness of the hydrogel decreases with time.8–10 Therefore, inhibiting the syneresis of hydrogel is the key to extend the validity of water-shutoff treatment.
Hydrogels formulated with HPAM and Cr have been used extensively as water-shutoff agents to reduce water production, and they also suffer syneresis problem.11–13 The common method to decrease the syneresis is increasing the HPAM concentration, but high HPAM concentration leads to the unreasonable cost and excess high injection pressure.14,15 Although a fairly wide number of new acrylamide polymers, which can be employed to prepare hydrogels, have been described in the literatures,16,17 only a relatively small number of them, including acrylamide copolymers of N-vinyl pyrrolidone (N-VP), 2-acrylamido-2-methyl-propanesulfonate (AMPS), and N-vinyl acetamide (N-VA), have been commercialized. Besides, several types of novel polymer hydrogels, such as topological (TP) hydrogels, double-network (DN) hydrogels and nanocomposite (NC) hydrogels have been developed.18–20 These polymer hydrogels were generally found to possess excellent stability, but their suitability for petroleum applications is limited by their high price. Besides, it is worth emphasizing that a more serious problem for most of the new polymers used in the hydrogel is their high adsorption on reservoir rock. Therefore, adding a simple and cost-effective stabilizer in the HPAM hydrogel may be the best method of improving the hydrogel stability to increase the validity of water-shutoff treatment.
In this paper, the sodium tripolyphosphate (STPP) was proved to be an effective syneresis inhibitor for HPAM/Cr hydrogel, and its mechanism of inhibiting syneresis was investigated in the aspect of the effect of STPP on HPAM. This investigation provides a clear understanding of the effect of STPP on HPAM, and the findings can be utilized for obtain the hydrogels with high stability.
Experimental section
1. Materials
Sodium bichromate (Na2Cr2O7), sodium sulfite (Na2SO3), sodium chloride (NaCl) and STPP are all analytically pure, and purchased from Sinopharm. HPAM was purchased from Beijing Hengju Chemical Group Corporation, and its molecular weight and hydrolysis degree is 4.7 × 106 g mol−1 and 25% respectively. It should be noted that the material concentration in the paper is on a weight basis.
2. Measurements of syneresis rate
First, the polymer stock solution was prepared by dissolving solid polymer in fresh water. A container with a known amount of water was vigorously stirred to create a deep vortex. Polymer was slowly added to the shoulder of the vortex to effectively wet the polymer beads. The container was sealed to minimize evaporation and was stirred continuously for 24 h to ensure complete dissolution of polymer. The crosslinker, whose amount was carefully tuned, was dissolved in water to prepare a crosslinker solution. Finally, the gelling solution was prepared by mixing the polymer stock solution and crosslinker solution. In the gelling solutions, the concentration of HPAM, Na2Cr2O7 and Na2SO3 was 0.2%, 0.3% and 0.5% respectively, which were obtained through many screening procedures. After the gelling solution was prepared, it was sealed in a bottle and put into an oven at 60 °C. Syneresis rate is defined as the decrease in the hydrogel weight at a given time relative to the initial hydrogel weight.
3. Measurements of viscosity
The 0.2% HPAM solutions with different concentrations of STPP were prepared respectively (to inhibit the oxidative degradation of HPAM, the oxygen in the solution has been removed by nitrogen), and then they were sealed in a bottle and put into an oven at 60 °C for different times. The viscosity of these solutions was measured at a shear rate of 1.22 s−1 by the Brookfield viscometer (DV-II+Pro) at 25 °C. Viscosity retention is defined as the viscosity at a given time relative to the initial viscosity of HPAM solution.
4. Measurements of light scattering
For the light scattering measurements, the polymer solutions were carried out in the light scattering vials. All glassware was kept dustfree by rinsing in hot acetone prior using. The solutions were filtered through membrane filters (pore size = 0.38 μm) directly into the vials. This process was carried out in a dustfree glovebox.
Static light scattering (SLS) and dynamic light scattering (DLS) measurements were carried out at 25 °C using a commercial multi-angle light scattering BI-200SM (Brookhaven Instruments Corporation) equipped with a digital correlator BI-9000AT. The SLS intensities were recorded from angles θ = 10° to 160°. The results of weight average molecular weight (Mw) and the second virial coefficient (A2) were obtained from the angular dependence of the excess absolute scattering intensity, known as the Rayleigh ratio R(q), on the basis of
where
K = 4π
n2(d
n/d
C)
2/
NAλ04, and
q = (4π
n/
λ0)sin(
θ/2), with
n, d
n/d
C,
λ0, and
θ being the solvent refractive index, the specific refractive index increment, the wavelength of the light
in vacuo, and the scattering angle, respectively. The SLS system was calibrated against a toluene standard. For the DLS measurement, the scattering angle and wavelength were fixed to be 90° and 532 nm respectively. The result of hydrodynamic radius (
Rh) was obtained by the dynamic light scattering software Ver. 5.74. All the light scattering measurements were repeated for three times under the same experimental condition.
5. Measurements of FTIR
FTIR spectra were obtained on a Nicolet 6700 FTIR Spectrometer. The HPAMs (ω = 0.2%) with 0 and 0.03% STPP in sealed bottles were all firstly put at 60 °C for 24 h, and then they were dried by the lyophilization method (vacuum freeze drying plant: Cammal-16LSC). The FTIR specimen was prepared by mixing the above dry HPAM sample with KBr, and spectral analysis was performed over the range 4000–400 cm−1.
6. Measurements of NMR
The STPP solution (ω = 0.3%), the HPAM solution (ω = 2%), and the composite solution composed of STPP (ω = 0.3%) and HPAM (ω = 2%) in sealed bottles were put at 60 °C for 24 h, and then they were dried by the lyophilization method. 31P solid state NMR spectra of the three dry samples were measured with a Bruker AVANCE III 400M instrument using a cross polarization pulse sequence, and operating at 161 MHz, with a 4 mm, 3-channel, magic angle spinning (MAS) probe. The contact time of the cross polarization/magic angle spinning experiment was set to be 100 μs. The acquisition time was 50 ms, the recycle delay was 5 s, and all NMR experiments were performed at room temperature. The spectra were processed and analyzed using Bruker Topspin V3.0.
Results and discussion
1. Effect of STPP on the hydrogel syneresis
HPAM/Cr hydrogel has been used extensively as water-shutoff agents to reduce water production in common oilfield on account of its low price and simple preparation. However, further application of this hydrogel in difficult reservoir condition is limited due to its unsatisfactory stability. In order to decrease the hydrogel syneresis, some inorganic additives, including sodium lactate, sodium salicylate, sodium oxalate, sodium malonate, STPP etc., were employed in the hydrogel, and the results showed that only STPP could inhibit the syneresis. The effect of the concentration of STPP on the hydrogel syneresis is showed in Fig. 1. For the hydrogel without STPP, the syneresis rate on the 180th day reaches up to 35%, and it is only 10% for the hydrogel with 0.01% STPP. When the concentration of STPP is 0.03%, the syneresis rate on the 180th day decreased to 3%. When the concentration of STPP is higher than 0.03%, the syneresis rate increases, but is still lower than that of the hydrogel without STPP. Hence, STPP (about 600$/t) is an effective syneresis inhibitor for HPAM/Cr hydrogel. To clarify the mechanism of STPP inhibiting the hydrogel syneresis, the effect of STPP on HPAM was investigated in the following parts.
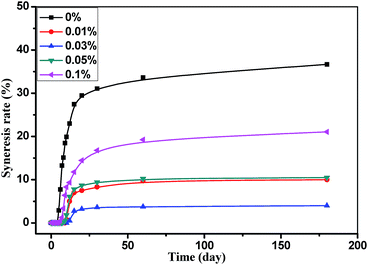 |
| Fig. 1 Effect of the concentration of STPP on the hydrogel syneresis. | |
2. Effect of STPP on the viscosity of HPAM
As showed in Fig. 2, the viscosity of HPAM with STPP decreases when the solutions were just prepared, and the high concentration of STPP results in the high decrease of the viscosity. It is because HPAM is susceptible to the salt, whereby STPP makes the HPAM molecule get coiled, therefore the viscosity reduces (salt sensitive effect).21 With the increase of the heating time, the viscosity of HPAM without STPP decreases continuously, which is caused by the thermal degradation of HPAM. In contrast, the viscosity of HPAM with STPP increases at first and then decreases slightly. The viscosity retention of HPAM with 0.01% STPP rises from 98.7% to 105.9% on the 1st day, whereas the one of HPAM with 0.1% STPP increases from 82.9% to 111.0% during the same time, which indicates that the high thickening rate is obtained by the high concentration of STPP. However, due to the negative “salt sensitive effect” of STPP, the highest viscosity is obtained when the STPP concentration is 0.03% rather than 0.1%.
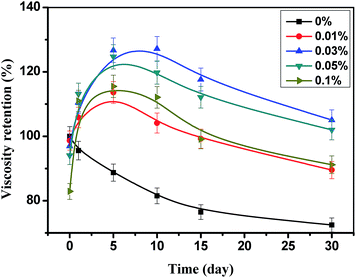 |
| Fig. 2 Effect of the concentration of STPP on the viscosity of HPAM prepared with fresh water. | |
When the fresh water was replaced by 1 mol L−1 NaCl to prepare the HPAM–STPP solution, the STPP still had thickening effect on the HPAM solution during the heating process, as showed in Fig. 3. Closer inspection of the data in Fig. 2 and 3 indicates that the thickening effect of HPAM is facilitated by NaCl, since the highest viscosity retention can reach up to 150% whereas only 130% in the absence of NaCl. Besides, when the STPP concentration is 0.03% and 0.1%, the highest viscosity retention was obtained on the 5th day and the 1st day respectively, but the viscosity of HPAM solution without NaCl is the highest on the 10th day and the 5th day, so the presence of NaCl in HPAM solution is benefit to the thickening rate.
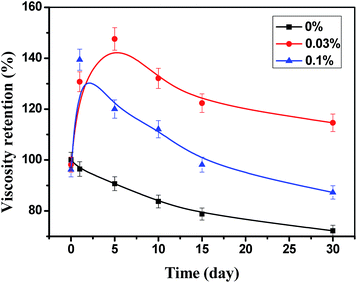 |
| Fig. 3 Effect of the concentration of STPP on the viscosity of HPAM prepared with 1 mol L−1 NaCl. | |
As it is generally accepted, the addition of common salts (NaCl, CaCl2, etc.) reduces the viscosity of the polymer solution, since the salt can compress the twin electrical layer and decrease the hydrophilicity of polymer, leading to the decrease of the polymer viscosity. However, STPP can increase the viscosity of HPAM solution. Hence, STPP is an uncommon salt for the HPAM solution, and it must have some especial effect on the HPAM.
3. Effect of STPP on the hydrophilicity of HPAM
The syneresis is the process that the water escapes from the network structure of hydrogel. This phenomenon can be intuitively understood as the following: the binding force of the HPAM to the water molecule is reduced, that is, the hydrophilicity of HPAM is decreased. Hence, the mechanism of the effect of STPP on the hydrogel syneresis was studied by investigating the influence of STPP on the HPAM hydrophilicity. The 0.2% HPAM solution with 0%, 0.03% and 0.1% STPP was respectively heat-treated at 60 °C for different days, and then the hydrodynamic radius (Rh) of HPAM was measured. The average Rh (aRh) is summarized in Fig. 4. As showed in Fig. 4, the aRh of HPAM without STPP decreases with time, and it reduces from 137.2 nm at the initial time to 52.2 nm on the 15th day. But for the HPAM with 0.03% STPP, it is interested that the aRh increases with time, reaching up to 162.3 nm from 105.2 nm during the same time. Generally, when the HPAM solution is mixed with common salts, such as NaCl, CaCl2 and MgCl2, the Rh of HPAM will decrease.21 It is because that the common salts reduce the electrostatic repulsion among HPAM molecules, and then the molecules get coiled. Obviously, the effect of STPP on the Rh of HPAM can't be explained by the above “salt sensitive effect”, and some other mechanisms must be existed in the STPP–HPAM system. To our best knowledge, it can be inferred that when STPP is exposed to the HPAM molecule, it may be “adsorbed” on the HPAM molecule chain by the electrostatic attraction or chemical reaction. Since STPP is highly hydrophilic, the hydration layer of STPP undoubtedly contributes to increase the hydration layer thickness of HPAM. As a result, the Rh of HPAM with STPP is higher than that of HPAM without STPP. However, when the “adsorption” of STPP reaches its saturation value, the surplus STPP will exist in free state in water, and then it will compete with the HPAM to adsorb water molecules, leading to the decrease of the Rh of HPAM.22 This may be the reason why the Rh of HPAM with 0.1% STPP is lower than that of HPAM with 0.03% STPP.
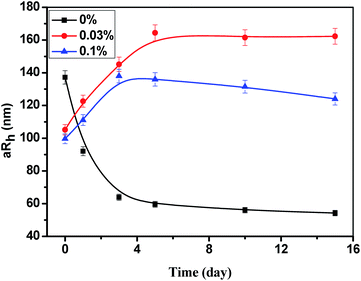 |
| Fig. 4 Effect of STPP on the aRh of HPAM. | |
Additional, the time dependence of the polydispersity index (PDI) affected by STPP is showed in Fig. 5. In the absence of STPP, the PDI firstly increases and then decreases, which is caused by the continuous degradation of HPAM. The PDI of HPAM sample with STPP increases with time, indicating the increase distribution width of molecular weight due to the aging process. It is also observed that the PDI with 0.1% STPP is higher than the one with 0.03% STPP. A high concentration of STPP leads to more “adsorption” of STPP onto HPAM molecule, whereby the HPAM with a high molecular weight is obtained. As a result, more STPP results in higher PDI.
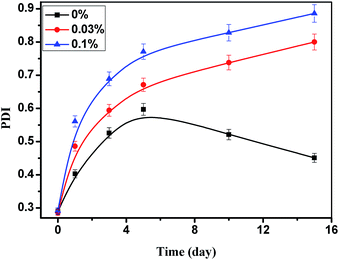 |
| Fig. 5 Time dependence of the PDI affected by STPP. | |
4. Effect of STPP on the Mw of HPAM
HPAM is a linear polymer made of the combination of acrylamide and acrylic acid. At high temperature condition, the molecular chain is easy to be broken, which has been considered as the reason leading to the hydrogel instability.23 The 0.2% HPAM solution with 0%, 0.03% and 0.1% STPP was respectively heat-treated at 60 °C for 24 h, and then the effect of STPP on the Mw of HPAM was investigated by the SLS technology. As showed in Fig. 6, the Mw of the three HPAMs is about 4.70 × 106 g mol−1 before the heat-treatment. The Mw of HPAM without STPP suffers an obvious decrease due to the heat-treatment, and reduces to 4.31 × 106 g mol−1. However, the Mw of HPAM with 0.03% STPP increases from 4.70 × 106 g mol−1 to 5.08 × 106 g mol−1. When the STPP concentration increases to 0.1%, the Mw of HPAM reaches up to 5.14 × 106 g mol−1. The results indicate that STPP can increase the Mw of HPAM, which may be a reason why STPP can improve the Rh of HPAM and thereby enhance the hydrogel stability.
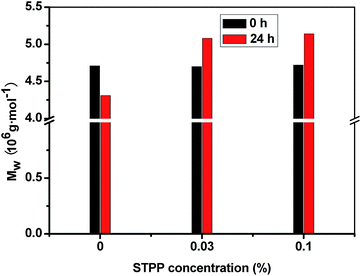 |
| Fig. 6 Effect of STPP on the Mw of HPAM. | |
Acrylamide polymers are susceptible to be degraded under thermal condition.24 The release of acrylamide monomer and the generation of ammonia due to the condensation reaction among the amide groups are the main reasons why the Mw of polymers decreases.25 The precious literatures26–28 indicate that the introduction of hydrophilic groups (for instance, acryloyloxyethyl trimethyl ammonium chloride, 2-acrylamido-2-methylpropanesulfonic acid) to the polymer molecular chain is beneficial to improve the stability of acrylamide polymers. The steric hindrance can be increased by these hydrophilic groups due to their large volume, and then the deamination reaction is inhibited. Besides, the introduction of hydrophilic groups changes the polymer molecular conformation, which can increase the energy to break the polymer backbone. Therefore, it can be supposed that a bridge connection may be formed between the STPP and HPAM resulted from the physical adsorption or the chemical reaction. For this reason, the Mw of HPAM with STPP increases due to the heat-treatment, and the stability of HPAM is improved.
5. Effect of STPP on the A2 of HPAM
The second virial coefficient (A2) can be used to characterize the interaction between macromolecules and solvent molecules. When A2 is positive, the net interaction between macromolecules and solvent molecules is attractive, and the macromolecule tends to dissolve; when A2 is negative, the net interaction between macromolecules and solvent molecules is repulsive, and the macromolecule tends to accumulate; when A2 is zero, the net interaction between macromolecules and solvent molecules equals to the interaction among macromolecules, and the macromolecule is in free status.29 As showed in Fig. 7, the A2 of the HPAM without STPP is 2.4 × 104 cm3 mol g−2 before the heat-treatment, and it decreases to 2.1 × 104 cm3 mol g−2 because of the heat-treatment. When 0.03% STPP is added to the HPAM solution, the A2 increases a lot, reaching up to 5.9 × 104 cm3 mol g−2, and it even increases to 8.1 × 104 cm3 mol g−2 after the heat-treatment. This indicates that the STPP promotes the dissolution of HPAM, and increases the hydrophilicity of HPAM. For the A2 of the HPAM with 0.1% STPP, it is up to 5.1 × 104 cm3 mol g−2, and increases to 6.6 × 104 cm3 mol g−2 due to the heat-treatment. Obviously, 0.1% STPP can also improve the hydrophilicity of HPAM, but its effect is worse than that of 0.03% STPP. These results are in good agreement with the data obtained from the DLS measurement, so it can be deduced that increasing the hydrophilicity of HPAM is an important reason of the STPP inhibiting the hydrogel syneresis.
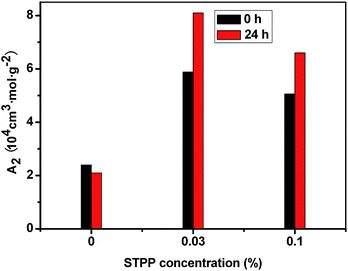 |
| Fig. 7 Effect of STPP on the A2 of HPAM. | |
6. Reaction mechanism of STPP and HPAM
STPP and pretreated HPAMs with 0 and 0.03% STPP were characterized by FTIR spectroscopy respectively. As showed in Fig. 8, the stretching peaks of the two HPAMs at 1410, 1570 and 1660 cm−1 assigned to C–N, C
O in –COO− and C
O in –CONH2 (ref. 30) respectively are nearly identical. However, due to the effect of STPP on HPAM, the stretching frequency of C–O in –COO− group of HPAM is increased from 1119 cm−1 to 1132 cm−1, and a new stretching peak of the HPAM/STPP system appears at 993 cm−1 which assigns to the C–O–P group.30 Therefore, it can be concluded that the STPP reacts with C–O in –COO− group of HPAM, whereby the new bond of C–O–P is formed.
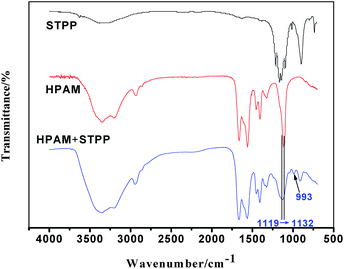 |
| Fig. 8 FTIR spectra of HPAM and HPAM/STPP. | |
To further verify the reaction between HPAM and STPP, 31P solid state NMR spectrum was measured, and the result was showed in Fig. 9. The NMR curves of the STPP with pretreated time of 0 h and 24 h are almost identical, which indicates that STPP may be not hydrolyzed at 60 °C in 24 hours. Due to the reaction between STPP and HPAM, the two absorption peaks (3 ppm, −6.85 ppm) merge into a wide one (−3.16 ppm), and a new characteristic peak at −15.04 ppm appears, which indicates the phosphorus in STPP molecule has reacted with HPAM and produced a novel chemical bond. According to the previous reports,31,32 the characteristic peak at about −15 ppm can be assigned to the C–O–P bond. It is therefore concluded that the crosslinking reaction can occur between STPP and HPAM and produce the C–O–P bond.
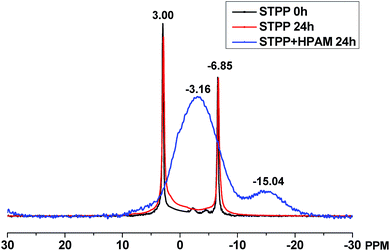 |
| Fig. 9 31P NMR spectrum of HPAM and HPAM/STPP (0 h and 24 h are the heat treatment time). | |
Hence, the mechanism of STPP affecting on HPAM is clarified: (1) because of the crosslinking reaction between STPP and HPAM, the intramolecular or intermolecular crosslinking occurs among the HPAM molecule (showed in Fig. 10), whereby the viscosity of HPAM solution is increased. (2) This crosslinking reaction results in the increase of the macromolecular chain length and the hydration layer thickness, so the Mw and aRh of HPAM rise. (3) The additional crosslinking between the –COO− group of HPAM and the crosslinker (Cr), which decreases the hydrophilicity of HPAM and then reduces the water-holding capacity of hydrogel, is considered as an important reason for the syneresis of HPAM hydrogel.33 The crosslinking between the –COO− group and STPP not only increases the hydrophilicity of HPAM (or the hydrogel network) due to the strong hydration capability of STPP, but also inhibits the additional crosslinking between the –COO− group and the Cr caused by the competition crosslinking. As a result, the hydrogel syneresis is mitigated. In conclusion, the increase of the hydrophilicity of HPAM (or the hydrogel network) improves the water-holding capacity of the hydrogel, whereby the hydrogel syneresis is decreased.
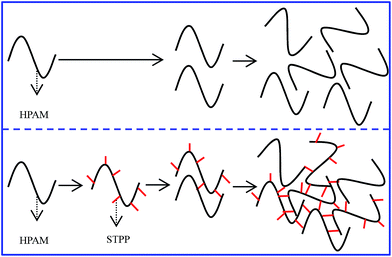 |
| Fig. 10 Schematic illustration of STPP affecting on HPAM. | |
7. Application of STPP in temperature–resistance hydrogel
Since HPAM is easy to be degraded at high temperature, the HPAM hydrogel is generally used below 80 °C.2 Although a fairly wide number of new polymers were generally found to possess excellent thermal stability, but the syneresis problem of the hydrogels formulated with these polymers at high temperature condition is still inevitable, since their dosage in the hydrogels is limited by their unreasonable cost. Hence, employing HPAM of low price to prepare temperature–resistance hydrogel is significantly important. On the basis of the crosslinking reaction between STPP and HPAM, a hydrogel, which is steady at 160 °C, has been developed. As showed in Fig. 11, the hydrogel without STPP suffers serious syneresis problem at 160 °C, and the syneresis rate on the 30th day reaches up to 95.3%, which means that almost all the hydrogel changes to water. Along with the increase of the STPP, the syneresis is decreased, and the syneresis rate can be reduced to 8.2%. It can be seen that STPP can dramatically improve the stability of the HPAM hydrogel at high temperature. To our best knowledge, this robust and persistent gel is the first example which is prepared with HPAM and inorganic chromium.
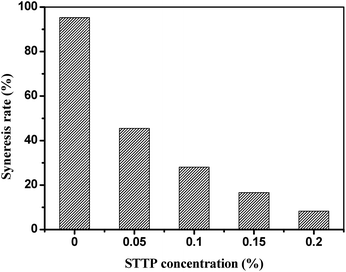 |
| Fig. 11 Effect of the concentration of STPP on the HPAM hydrogel syneresis on the 30th day (composition: 0.4% HPAM + 0.3% Na2Cr2O7 + 0.5% Na2SO3 + 0.3% thiourea + 0.5% bentonite + x% STPP, x = 0–0.2). | |
As showed in Fig. 12, the thermostability of the hydrogel can be further improved below 160 °C, and the syneresis rate on the 30th day is all below 8.2% at the temperature range from 90 °C to 160 °C. Therefore, this HPAM hydrogel can not only be a water shutoff agent in high-temperature oil reservoir, but also be selected as a channeling sealing agent in the thermal recovery of heavy oil.
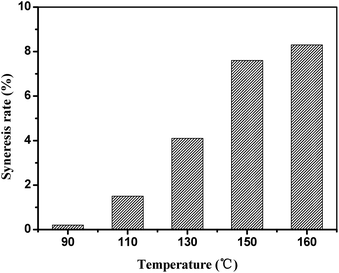 |
| Fig. 12 Effect of temperature on the HPAM hydrogel syneresis on the 30th day. | |
Conclusions
STPP is an effective syneresis inhibitor for the hydrogel formulated with HPAM. The mechanism of STPP inhibiting the syneresis of HPAM hydrogel is the following: STPP molecule can react with the C–O in –COO− group of HPAM whereby the new bond of C–O–P is formed, leading to a large quantity of STPP molecule “adsorbing” on the molecular chains of HPAM. As a result, the hydrophilicity of HPAM is increased due to the strong hydrophilicity of STPP, and the degradation of HPAM is inhibited owing to the intramolecular or intermolecular crosslinking between HAPM and STPP. The increase of the hydrophilicity and stability of HPAM improves the water-holding capacity of the hydrogel, whereby the hydrogel syneresis is decreased. On the basis of the crosslinking reaction between STPP and HPAM, a hydrogel prepared with HPAM and Cr3+ has been developed and can be successfully used in high temperature oil reservoir of 160 °C.
Acknowledgements
Financial support by the Natural Science Foundation of China (NSFC) (Grant 51474234 and 51474235), China Postdoctoral Science Foundation funded project (2014M551988), the Special Funds for Shandong Province Postdoctoral Innovative Projects (201302016) and Shandong Provincial Natural Science Foundation, China (ZR2014EZ002) is gratefully acknowledged.
Notes and references
- M. Zargartalebi, R. Kharrat and N. Barati, Fuel, 2015, 143, 21–27 CrossRef CAS PubMed.
- K. S. M. El-Karsani and G. A. Al-Muntasheri, SPE J., 2014, 19, 135–149 CrossRef.
- F. C. Wang and H. A. Wu, Soft Matter, 2013, 9(33), 7974–7980 RSC.
- H. Jia, J. Z. Zhao and F. Y. Jin, Ind. Eng. Chem. Res., 2012, 51(38), 12155–12166 CAS.
- R. S. Seright, J. Liang and W. B. Lindquist, J. Pet. Sci. Eng., 2003, 39(3), 217–230 CrossRef CAS.
- H. Zhang, R. S. Challa and B. Bai, Ind. Eng. Chem. Res., 2010, 49(23), 12284–12293 CrossRef CAS.
- W. Wang, Y. Liu and Y. Gu, Colloid Polym. Sci., 2003, 281(11), 1046–1054 CAS.
- A. Moradi-Araghi, J. Pet. Sci. Eng., 2000, 26(1), 1–10 CrossRef CAS.
- P. Albonico and T. P. Lockhart, J. Pet. Sci. Eng., 1997, 18(1), 61–71 CrossRef CAS.
- S. M. Vargas-Vasquez and L. B. Romero-Zerón, Pet. Sci. Technol., 2009, 27(15), 1727–1743 CrossRef CAS PubMed.
- S. M. Vargas-Vasquez and L. B. Romero-Zerón, Pet. Sci. Technol., 2008, 26(4), 481–498 CrossRef CAS PubMed.
- H. Jia, J. Z. Zhao and F. Y. Jin, Ind. Eng. Chem. Res., 2012, 51(38), 12155–12166 CAS.
- J. Liu, X. Lu and S. Sui, J. Appl. Polym. Sci., 2012, 124(5), 3669–3677 CrossRef CAS PubMed.
- A. D. Koohi, M. V. Seftie and A. Ghalam, Iran. Polym. J., 2010, 19(10), 757–770 CAS.
- R. Wilton and K. Asghari, J. Can. Pet. Technol., 2007, 46(2), 33–39 CAS.
- L. Mespouille, J. L. Hedrick and P. Dubois, Soft Matter, 2009, 5(24), 4878–4892 RSC.
- J. Genzer and R. R. Bhat, Langmuir, 2008, 24(6), 2294–2317 CrossRef CAS PubMed.
- P. Fu, K. Xu, H. Song, G. Chen, J. Yang and Y. Niu, J. Mater. Chem., 2010, 20, 3869–3876 RSC.
- Z. Hu and G. Chen, J. Mater. Chem. A, 2014, 2, 13593–13601 CAS.
- Z. Hu and G. Chen, Adv. Mater., 2014, 26, 5950–5956 CrossRef CAS PubMed.
- Q. Ying, G. Wu and B. Chu, Macromolecules, 1996, 29(13), 4646–4654 CrossRef CAS.
- Y. Mansourpanah and Z. Amiri, Desalination, 2014, 335(1), 33–40 CrossRef CAS PubMed.
- W. M. Leung, D. E. Axelson and J. D. van Dyke, J. Polym. Sci., Part A: Polym. Chem., 1987, 25(7), 1825–1846 CrossRef CAS PubMed.
- E. A. Smith, S. L. Prues and F. W. Oehme, Ecotoxicol. Environ. Saf., 1996, 35(2), 121–135 CrossRef CAS PubMed.
- M. J. Caulfield, G. G. Qiao and D. H. Solomon, Chem. Rev., 2002, 102(9), 3067–3084 CrossRef CAS PubMed.
- X. Tian, E. Ren and J. Wang, Carbohydr. Polym., 2012, 87(3), 1956–1962 CrossRef CAS PubMed.
- C. C. Torres, B. F. Urbano and C. H. Campos, Mater. Chem. Phys., 2015, 152, 69–76 CrossRef CAS PubMed.
- T. C. Suekama, J. Hu and T. Kurokawa, ACS Macro Lett., 2013, 2(2), 137–140 CrossRef CAS.
- N. A. Mahynski, S. K. Kumar and A. Z. Panagiotopoulos, Soft Matter, 2015, 11(2), 280–289 RSC.
- R. M. Silverstein, G. C. Bassler and T. C. Morrill, Spectrometric identification of organic compounds, John Wiley & Sons, New York, 2001 Search PubMed.
- J. H. Letcher and J. R. van Wazer, J. Chem. Phys., 1966, 44(2), 815–829 CrossRef CAS PubMed.
- D. G. Gorenstein, Phosphorous-31 NMR: principles and applications, Academic Press, New York, 2012 Search PubMed.
- J. R. Gales, T. S. Young and G. P. Willhite, SPE J., 1994, 2(02), 190–198 Search PubMed.
|
This journal is © The Royal Society of Chemistry 2015 |