DOI:
10.1039/C5RA17266G
(Paper)
RSC Adv., 2015,
5, 91723-91733
64CuCl2 produced by direct neutron activation route as a cost-effective probe for cancer imaging: the journey has begun
Received
26th August 2015
, Accepted 20th October 2015
First published on 20th October 2015
Abstract
Copper-64 (t½ = 12.7 h, E.C. 45%, β− 37.1%, β+ 17.9%) is a promising radionuclide for positron emission tomography (PET) imaging and is generally produced by the 64Ni(p, n)64Cu nuclear reaction in a cyclotron. Despite the excellent attributes of 64Cu for PET imaging, the utility of this radioisotope is still limited to countries having good cyclotron facilities and excellent production logistics. We have explored the feasibility of using 64Cu in the form of 64CuCl2, produced in research reactors by an (n, γ) route, as a probe for tumor imaging by PET. The production strategy has been optimized to obtain 64CuCl2 with adequate specific activity and radionuclidic purity suitable for clinical use. Exploring copper metabolism as an imaging biomarker, 64CuCl2 can directly be used as an effective probe for non-invasive visualization of tumors. The stability of 64CuCl2 under physiological conditions was assessed by detailed in vitro studies in mouse serum medium. The biological efficacy of 64CuCl2 was studied in C57BL/6 mice bearing melanoma tumors and Swiss mice bearing fibrosarcoma tumors. The results of the biodistribution studies revealed significant tumor uptake (7.64 ± 1.71% ID per g in melanoma, 6.54 ± 1.41% ID per g in fibrosarcoma) within 4 h post-injection, with good tumor-to-background contrast. To the best of our knowledge, this is the first study which employs neutron activated 64CuCl2 as a potential radiotracer for PET imaging in preclinical settings. Owing to the availability of several medium-flux nuclear reactors with good geographical distribution in different parts of the world, the promising results obtained in this study would set the stage for wide-scale utilization of neutron activated 64CuCl2 as a cost-effective probe for PET imaging.
Introduction
The role of molecular imaging to visualize, characterize, and quantify endogenous biological processes at the cellular and molecular levels in living subjects using a range of imaging modalities is well recognized.1–4 Among various imaging modalities, PET is increasingly being used in clinics all over the world for medical decision making and patient management.5 Evolution and continued success of PET, has been, in large part, due to the evolution of imaging technology and rapid development of specific probes using suitable positron-emitting radionuclides. The main factors contributing to the choice of the positron-emitting radionuclides are their physical and chemical characteristics, availability, and the timescale of the biological process intended to be studied.
Copper-64 (t½ = 12.7 h, E.C. 45%, β− 37.1%, β+ 17.9%) is a unique intermediate half-lived positron-emitting radionuclide which decays by three processes, namely, electron capture, β− and β+ decays.6 There appears to be enticing interest to consider the use of 64Cu owing to simultaneous emission of β− and β+ particles, which holds promise not only in development of diagnostic radiotracers for PET imaging but can also be used in preparation of radiotherapeutic agents for cancer treatment.7 While the attributes of 64Cu is quite promising, utilization of this radioisotope is mostly limited to preclinical studies and that too mainly in the developed countries of the world.7 Currently, use of 64Ni(p, n)64Cu reaction in a cyclotron has remained as the most common procedure for the production of 64Cu.6 While this cyclotron production route constitutes a viable approach, the implicit need of cyclotron facilities and elaborate procedures of radiochemical separation and purification as well as excellent logistics for its supply to distant user sites are some of the issues that made availability of this radioisotope very limited. Competition from other established PET radiotracers based on 18F and 68Ga with easier availability might be the other reason behind less popularity of this radioisotope in clinical context.
Copper-64 can also be produced in a nuclear reactor adopting thermal neutron capture, 63Cu(n, γ)64Cu, or the fast neutron induced, 64Zn(n, p)64Cu reactions.8,9 Though the latter route results in production of 64Cu in a no-carrier-added (NCA) form, it requires access to the reactor with reasonably high fast neutron flux positions which is not available in majority of research reactor facilities across the world. On the other hand, majority of the radioisotopes produced in the research reactors across the world are the products of thermal neutron induced nuclear reactions, mainly by (n, γ) reaction.10 Among the available options for production of 64Cu, the 63Cu(n, γ)64Cu reaction represents the least intricate route since it precludes the separation of 64Cu from its precursor. Moreover, the neutron activation route involves negligible radioactive waste generation and is inexpensive.
While the 63Cu(n, γ)64Cu production route is quite promising, this approach provides 64Cu of low specific activity which is unsuitable for labeling receptor targeted ligands.6,7 In this premise, use of neutron activated 64Cu in the form of 64CuCl2 as a radiotracer for non-invasive assessment of various types of cancers was deemed worthy of consideration and there are few reports to support its merit.11–14 It is worthwhile to point out that adaptation of this strategy would obviate the radiolabeling step, preclude the need of expensive target specific ligands such as peptides and antibodies, and would be easy to implement under current good manufacturing practices (cGMP) compliant conditions because simple target dissolution capabilities would suffice to produce the radiotracer in a form suitable for use in PET imaging.
Herein, we report a systematic approach for establishing 64CuCl2 produced by direct neutron activation route in a medium flux research reactor as a cost-effective probe for cancer imaging by PET. Towards this, the irradiation parameters (neutron flux and irradiation time) for production of 64CuCl2 with adequate specific activity and radionuclidic purity for use as a PET radiotracer were optimized by theoretical calculations. The practicality of the approach was demonstrated by production of this radioisotope in several batches in the Dhruva reactor of our research centre. As a proof of concept, the efficacy of the radiotracer was established by biodistribution studies in C57BL/6 mice bearing melanoma tumors and Swiss mice bearing fibrosarcoma tumors.
Experimental
Materials and equipment
Copper oxide (spectroscopic grade > 99.99% pure) used as the target material for production of 64Cu was obtained from E. Merck, Germany. All other chemicals were of Analytical Reagents (AR) grade and purchased from established manufacturers. Standard source of 152Eu (t½ = 13.6 year) was obtained from Amersham, Inc., USA. Flexible silica plates (coating thickness 0.25 mm) from J. T. Baker Chemical Company, USA were used for thin layer chromatography (TLC) studies. The activity of 64Cu was measured using a pre-calibrated Capintec dose calibrator (Ramsey, NJ). The presence of radionuclidic impurities in 64Cu was determined by recording gamma ray spectra using a calibrated high purity germanium detector (HPGe) detector coupled to a 4K multichannel analyzer (MCA) system. All other radioactivity measurements were carried out using a well-type NaI(Tl) scintillation counter, unless mentioned otherwise, keeping the baseline at 400 keV and a window of 200 keV thereby utilizing the 511 keV gamma photon of 64Cu due to positron-annihilation. Dynamic light scattering (DLS) measurements were performed using a Malvern 4800 Autosizer employing a 7132 digital correlator for the determination of hydrodynamic diameter.
Production of 64Cu via (n, γ) route
Copper-64 was produced by thermal neutron bombardment on natural CuO (69.1% in 63Cu) target. A known amount of target was taken in a quartz ampoule. The ampoule was subsequently flame sealed and irradiated at a thermal neutron flux of ∼1 × 1014 n cm−2 s−1 for 7 days in Dhruva research reactor at Bhabha Atomic Research Centre, India. At the end of irradiation, the irradiated targets were cooled for 24 h and subsequently dissolved in 5 mL of 1 M suprapure HCl by gentle warming inside a lead shielded facility. The resultant solution was evaporated to near dryness and reconstituted in 2 mL of de-ionized water.
Determination of yield and specific activity of 64Cu produced via (n, γ) route
After radiochemical processing, the activity of 64Cu was measured using a dose calibrator. The activity of 64Cu was accurately determined using HPGe detector coupled to MCA system. For this purpose, a small aliquot of 64Cu was withdrawn and the γ-spectrum was recorded after appropriate dilution of the radioactivity so that the dead time of the detector was <5%. Energy and efficiency calibrations of the HPGe detector were carried out using standard 152Eu reference source prior to the measurement of activity. The specific activity was determined from the total yield of 64Cu and expressed as TBq g−1 of Cu.
Quality control of 64Cu produced via (n, γ) route
Radionuclidic purity.
The presence of radionuclidic impurities in 64Cu produced via (n, γ) route could be determined from the γ-spectrum of 64Cu recorded using HPGe detector coupled to MCA system, as described above. For accurate quantification of the level of radionuclidic impurities, the γ-spectra of 64Cu samples were recorded after allowing them to decay for 1 week. The characteristic γ-peaks corresponding to the extraneous radionuclides were monitored for determination of their radioactivity. From this data, the level of radionuclidic impurities in 64Cu was calculated after proper decay corrections.
Radiochemical purity.
The radiochemical purity of 64Cu produced in the form of 64Cu2+ ions was determined by radio-thin layer chromatography (radio-TLC) assay. For this purpose, 5 μL of 64CuCl2 solution was spotted on a TLC plate at 1.5 cm from the bottom. The chromatogram was developed in 0.1 M sodium citrate medium. Subsequently, the TLC plate was dried and cut into 1 cm pieces and activity of each piece was determined using a NaI(Tl) counter. The radiochemical purity of 64Cu in the form of 64Cu2+ ion was expressed as the percentage of the total activity which migrated to the solvent front (Rf = 0.9–1) in the chromatogram developed.
Determination of octanol/water partition coefficients (log
D) of 64CuCl2 and 64Cu–NOTA complex
The octanol/water partition coefficient (log
D) of 64CuCl2 was determined adopting the reported method.15 In brief, 200 μL of 64CuCl2 was added to 1.8 mL of phosphate buffered saline solution (pH ∼ 7.4) and thoroughly mixed. Subsequently, 2 mL of 1-octanol was added to the above solution, vortexed for 1–2 min. The layers were separated by centrifugation and 100 μL aliquots were withdrawn from each layer and counted in a NaI(Tl) scintillation counter. A 0.8 mL aliquot of the octanol layer was withdrawn and added to an equal volume of normal saline solution and the extraction process was repeated, as above. The results of this back extraction were used to compute the ratio of the activity in octanol to phosphate buffered saline layers and the log
D value was calculated.
Also, 1,4,7-triazacyclononane-1,4,7-triacetic acid (NOTA) was radiolabeled with 64Cu as per the reported procedure16 and log
D value for 64Cu–NOTA complex at pH ∼ 7.4 was determined as described above.
Study on protein aggregation in mouse serum in presence of Cu2+ ions by dynamic light scattering
DLS measurements were carried out for mouse serum samples containing varied amounts of CuCl2 (6.7–33.3 μg Cu per mL) using a Malvern 4800 photon correlation spectrometer. The instrument is equipped with a He–Ne laser (λ = 632.8 nm) with a vertically polarized light. All measurements were carried out at an output power of 15 mW and at 25 ± 0.1 °C. The intensity correlation functions were first analyzed by the method of cumulants to extract the average hydrodynamic diameter and polydispersity index of size distribution. Since the polydispersity index was high (>0.3) in all samples, all data were analyzed by the CONTIN algorithm for inverse Laplace transformation of the correlation function. The corresponding fits are shown as solid line in the graph. The correlation function was measured five times for each sample.
In vitro stability of 64CuCl2 in mouse serum
The stability of CuCl2 in mouse serum medium was studied by low resolution nuclear magnetic resonance (NMR) diffusivity measurements using Xigo Nanotools Acorn Area instrument. The homogenous solution of CuCl2 in mouse serum (20 μg Cu per mL of serum) was filled in the NMR tube and inserted into the magnetic field. The relaxation time was determined at 25 ± 0.1 °C by T2 measurement using Carr–Purcell–Meiboom–Gill (CPMG) method.17
The stability CuCl2 in mouse serum medium was also studied by radio-TLC assay. For this purpose, tracer level of 64Cu was mixed with inactive CuCl2 and added to mouse serum to maintain an effective concentration of 20 μg Cu per mL and incubated at 37 ± 0.1 °C. The radio-TLC pattern was developed adopting the procedure described earlier. The stability of CuCl2 in mouse serum over a prolonged period of time was analyzed by radio-TLC assays at different time intervals.
Biodistribution studies in tumor bearing mice
All animal studies were conducted under a protocol approved by the Bhabha Atomic Research Centre Animal Ethics Committee. The biological behavior of the radiotracer prepared was studied in C57BL/6 mice bearing melanoma tumors and Swiss mice bearing fibrosarcoma tumors. Melanoma tumors were developed by injecting ∼1 × 106 melanoma cells (ATCC®-CRL-6475™) suspended in 200 μL of phosphate buffered saline (PBS) subcutaneously into the right thigh of each C57BL/6 mouse weighing 20–25 g. Similarly, fibrosarcoma tumors were raised in Swiss mice by injecting ∼1 × 106 fibrosarcoma cells (ATCC® CRL-2295™) suspended in 200 μL of phosphate buffered saline (PBS) subcutaneously into the right thigh of each mouse weighing 20–25 g. The animals were observed for visibility of tumors and subsequently allowed to grow for about 2 weeks to attain a tumor mass of 0.2–0.4 g.
The radiotracer (64CuCl2 in 0.9% NaCl medium, 100 μL, 3.7–5.5 MBq) was injected into each animal through a lateral tail vein. The animals were sacrificed by cardiac puncture post-anesthesia at 1, 4, 24 and 48 hours post-injection (p.i.). Four animals were used at each time point. Various organs, tissues, and tumors were excised after sacrifice, washed with physiological saline, dried, and the radioactivity associated with each organ and tissue was determined using a flat-type NaI(Tl) counter. The weight of each organ and tumor was also determined by using an analytical balance. The percent injected activity (% ID) in various organs, tissues, and tumor was calculated from the above data and expressed as percentage injected activity per gram (% ID per g) of organ/tissue.
As control experiment, 64Cu–NOTA complex was prepared as per the reported procedure16 and administered (100 μL, 3.7–5.5 MBq) in melanoma and fibrosarcoma tumor bearing mice and biodistribution studies were carried out as described above.
Results
Production of 64Cu via (n, γ) route
Copper-64 can be produced by neutron activation of 63Cu. The production of 64Cu along with the possibility of formation of other radionuclidic impurities is indicated in Fig. 1. During the course of irradiation, 64Cu decays to stable 64Zn by β− decay, which in turn would undergo neutron activation to form long-lived 65Zn (t½ = 244 d) (Fig. 1A). Also, 64Cu decays by β+ decay to stable 64Ni, which would undergo neutron activation to form 65Ni (t½ = 2.5 h) (Fig. 1A). Since, natural CuO is used for irradiation, 66Cu (t½ = 5.1 min) would also be produced by neutron activation of 65Cu (Fig. 1A). Apart from 65Zn, other short lived radionuclidic impurities (65Ni and 66Cu) present in 64Cu are not of much concern as they would decay completely within few hours after end of irradiation (Fig. 1B).
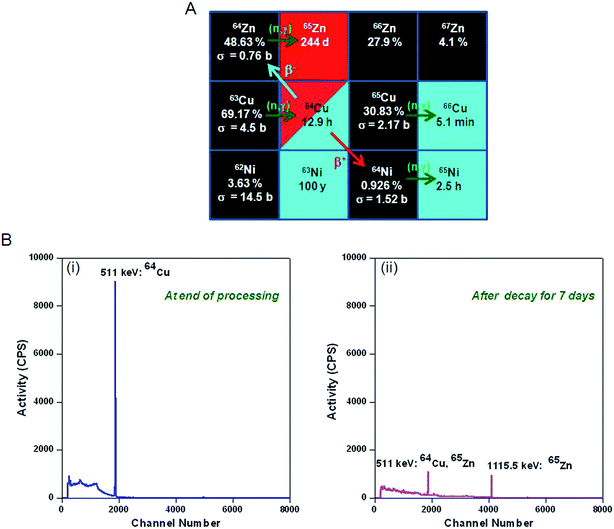 |
| Fig. 1 Production of neutron activated 64Cu. (A) Production of 64Cu along and possibility of co-production of radionuclidic impurities on irradiation of natural CuO target in a research reactor. (B) γ-spectra of 64Cu (i) just after completion of radiochemical processing and (ii) after decay for 7 days. | |
The activity of 64Cu produced by thermal neutron activation of natural CuO oxide in a research reactor can be theoretically calculated using the standard Bateman equations:18
|  | (1) |
| A64Cu(t) = N64Cu(t) × λ64Cu | (2) |
| 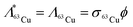 | (3) |
| 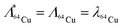 | (4) |
where,
N64Cu(
t) and
A64Cu(
t) are the number of atoms and activity of
64Cu produced on irradiation of
63Cu target for time ‘
t’,
σ63Cu is the thermal neutron capture cross-section of
63Cu, and
λ64 is the decay constant of
64Cu.
The yield of the extraneous radionuclide, 65Zn, can also be calculated using similar Bateman equations:
|  | (5) |
| A65Zn(t) = N65Zn(t) × λ65Zn | (6) |
| 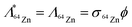 | (7) |
where,
N65Zn(
t) and
A65Zn(
t) are the number of atoms and activity of
65Zn produced on irradiation of
63Cu target for time ‘
t’,
σ64Zn is the thermal neutron capture cross-section of
64Zn, and
λ65 is the decay constant of
65Zn.
Based on the above equations, activities of 64Cu and 65Zn produced during neutron irradiation of natural CuO at different neutron flux positions and for different irradiation times were theoretically calculated in order to arrive at the optimum conditions that would provide 64Cu with maximal specific activity while ensuring that the contamination from 65Zn impurity was negligibly low (Fig. 2).
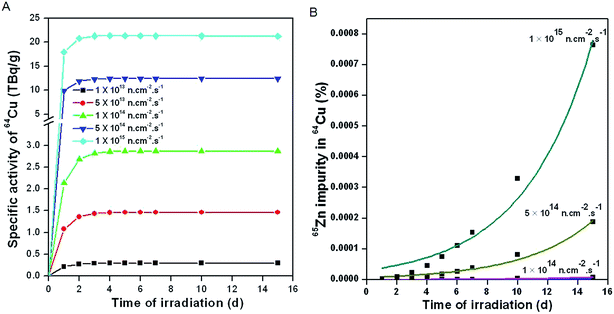 |
| Fig. 2 Optimization of irradiation conditions for production of neutron activated 64Cu based on theoretical calculations using Bateman equation. (A) Specific activity of 64Cu, (B) level of 65Zn impurity co-produced (as a % of 64Cu), on irradiation of 1.25 g CuO (equivalent to 1 g Cu) target at different flux positions for different time intervals. | |
Fig. 2A depicts the calculated specific activity of 64Cu at different flux positions for different durations of irradiation. It is evident from the figure that the specific activity of 64Cu generally increases with increase in flux of the irradiation position. However, the specific activity of 64Cu reaches a level of saturation within 2 days of irradiation, indicating that this is the optimal irradiation time at all flux positions. When irradiation is carried out at flux ≤1 × 1014 n cm−2 s−1, the level of 65Zn impurity formed in 64Cu is negligibly low (Fig. 2B). The level of the radionuclidic impurity significantly increases with increase in flux as well as the time of irradiation (Fig. 2A).
Though, 64Cu can be produced with appreciably high specific activity on irradiation at flux >1 × 1014 n cm−2 s−1, the radionuclidic impurity burden of long-lived 65Zn is a cause of major concern from the perspective of clinical utility of this radioisotope. Owing to the short half-life of 64Cu, the radionuclidic impurity burden of 65Zn in 64Cu would rapidly increase with time. Hence, in order to minimize the radionuclidic impurity in 64Cu, it is essential to carry out the irradiation of 63Cu under lower flux conditions (∼1 × 1014 n cm−2 s−1). This would however lower the specific activity of 64Cu (Fig. 2A). A balance between specific activity and radionuclidic purity of 64Cu would be required in order to utilize the radioisotope for clinical PET imaging.
Production yield and specific activity of 64Cu from practical irradiations via (n, γ) route
The practicality of the theoretical calculations discussed in the previous section was experimentally demonstrated by production of 64Cu by irradiation of natural CuO target in the Dhruva reactor at a flux of ∼1 × 1014 n cm−2 s−1 for 7 days. Though the theoretical calculations indicated saturation of activity of 64Cu on irradiation for >2 d, the minimum time-slot for irradiation available in our research reactor is 7 days and the target cannot be taken out of the reactor in between. On irradiation of 13–22 mg of natural CuO target, the activity of 64Cu after radiochemical processing varied between 30 and 57 GBq, which is appreciably high for both preclinical as well as clinical studies (Table 1). The specific activity of 64Cu produced via (n, γ) route in different batches was ∼3 TBq g−1. Owing to its simplicity, the production process is easily amenable for scale-up to meet the demand of the nuclear medicine community in future.
Table 1 Production of (n, γ)64Cu in different batches by irradiation of natural CuO target in the Dhruva reactor at a flux of ∼1 × 1014 n cm−2 s−1 for 7 days
Batch No. |
Target weight (mg) |
At the end of radiochemical processing (8 h after end of irradiation) |
After decay for 24 h (16 h after end of radiochemical processing) |
Activity of 64Cu [GBq (Ci)] |
Specific activity of 64Cu [TBq (Ci) g−1] |
65Zn impurity in 64Cu (%) |
Activity of 64Cu [GBq (Ci)] |
Specific activity of 64Cu [TBq (Ci) g−1] |
65Zn impurity in 64Cu (%) |
1 |
13.3 |
30.7 (0.83) |
2.9 (78.3) |
1.8 × 10−6 |
13.0 (0.35) |
1.2 (33.0) |
4.3 × 10−6 |
2 |
15.0 |
35.5 (0.96) |
3.0 (80.6) |
2.4 × 10−6 |
15.0 (0.40) |
1.3 (33.6) |
5.4 × 10−6 |
3 |
22.1 |
56.6 (1.53) |
3.2 (86.9) |
1.2 × 10−6 |
23.9 (0.64) |
1.3 (36.3) |
2.9 × 10−6 |
4 |
14.2 |
36.5 (0.99) |
3.1 (83.2) |
2.1 × 10−6 |
15.5 (0.42) |
1.3 (35.3) |
5.1 × 10−6 |
5 |
13.0 |
34.0 (0.91) |
3.2 (87.8) |
1.3 × 10−6 |
14.3 (0.38) |
1.4 (36.6) |
3.1 × 10−6 |
Quality control of 64Cu produced via (n, γ) route
Radionuclidic purity.
The radionuclidic purity of 64Cu produced was determined by γ-ray spectrometry using a calibrated HPGe detector coupled to MCA system (Fig. 1B). The γ-ray spectra of 64Cu recorded immediately after the radiochemical processing only shows the 511 keV annihilation peak and no photopeak corresponding to 65Zn could be seen. However, when 64Cu samples were decayed for 7 days, the 511 keV and 1115.5 keV photopeaks corresponding to 65Zn could be seen in the γ-spectra (Fig. 1B). Decay of 64Cu samples for 7 days was required for quantitative estimation of the trace level of 65Zn impurities, the characteristic photopeaks of which otherwise got masked by the intense 511 keV peak of 64Cu in the γ-spectra. By analysis of the γ-spectra, the levels of radionuclidic impurities at end of radiochemical processing and 24 h after end of irradiation (as shown in Table 1) were calculated applying suitable decay corrections.
The level of 65Zn present in 64Cu was found to be <10−5% at the end other radiochemical processing (Table 1). Since radiochemical processing of 64Cu was performed at least 8 h after the end of irradiation, the presence of other short-lived radioisotopes (65Ni and 66Cu) could not be detected. No other radionuclidic impurity in 64Cu could be detected from the γ-spectra. Assuming that the radioisotope if approved for clinical studies would reach the hospitals only on the next day, the specific activity and radionuclidic purity of 64Cu were determined at an interval of 24 h after end of irradiation (Table 1). Though the specific activity decreased significantly within this period of time, the level of 65Zn impurity was still negligibly low (<10−5%) and hence 64CuCl2 was suitable for clinical use (Table 1). Irradiations were carried out in 5 different batches and the results were quite reproducible in all the batches.
Radiochemical purity.
For determination of radiochemical purity of 64Cu, the TLC was developed in 0.1 M sodium citrate medium which would form copper citrate complex with 64Cu in ionic form. On development of the chromatogram, the activity at the point of application (Rf = 0) indicates the presence of colloidal 64Cu, while the activity at the solvent front (Rf = 0.9–1) is indicative of the amount of 64Cu in ionic form (Cu2+). After radiochemical processing, the radiochemical purity of 64Cu in the form of Cu2+ ion was determined to be >99% in all the batches.
Determination of octanol/water partition coefficients (log
D) of 64CuCl2 and 64Cu–NOTA complex
The log
D values of 64CuCl2 and 64Cu–NOTA at pH 7.4 (the physiological pH of blood serum) were determined to be −2.5 ± 0.2 and −2.2 ± 0.1 (n = 5), respectively, which indicates that both the compounds are highly hydrophilic in nature.
Study on protein aggregation in mouse serum in presence of Cu2+ ions by dynamic light scattering
Serum albumin is an important protein present in blood and is capable of binding with various additives such as drugs, hormones, transition metal ions etc.19 Reports indicate that the presence of metal ions such as Cu2+, Zn2+etc. lead to aggregation of a variety of proteins including human serum albumin.19 To determine the cut-off limit for administration of neutron activated 64CuCl2 in biological system so that aggregation of serum protein could be avoided, varying amounts of CuCl2 were added to mouse serum (6.7–33.3 μg Cu per mL) and characterized by DLS study. DLS is an ensemble averaged technique used to study the aggregation of proteins under different experimental conditions. As a control study, DLS measurement of ‘blank’ mouse serum medium was also recorded. DLS study of ‘blank’ mouse serum indicated presence of nanosized protein particles with effective hydrodynamic diameter ∼76 nm. Particle size information after addition of varying amounts of CuCl2 in mouse serum was obtained by analysis of the electric field correlation function, [g(τ)], calculated over a wide range of correlation time, τ.20 A significant shift in g(τ) was observed only when the concentration of CuCl2 in mouse serum was >20 μg mL−1, indicating the formation of colloidal aggregates above this concentration (Fig. 3A). Moreover, the aggregation was found to be time dependent, once the concentration of Cu2+ ions exceeds 20 μg mL−1. To understand the time-dependent increase in the particle size of the aggregates, DLS measurements were made at different time intervals after addition of CuCl2 (26.7 μg Cu per mL) in mouse serum and g(τ) was calculated (Fig. 3B). As indicated from the shift in g(τ), the effective particle size increased from 76 nm to 176 nm over a period of 40 min (inset of Fig. 3B). This study indicated that in all in vivo studies in mice the concentration of Cu2+ ions in blood should preferably be ≤20 μg mL−1 to avoid aggregation of serum proteins.
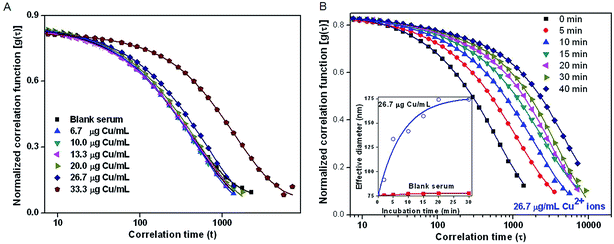 |
| Fig. 3 Correlation function from serum samples containing (A) different concentration of CuCl2, (B) 26.7 μg Cu per mL of serum at different time intervals. Inset shows the variation in effective particle size of serum sample with time. | |
In vitro stability of CuCl2 in mouse serum
The negligible tendency of CuCl2 to induce protein aggregation at a concentration ≤20 μg mL−1 was corroborated by analysis of the dispersion behavior of the mouse serum medium over a prolonged period of 24 h (Fig. 4A). Homogenous solution of CuCl2 in mouse serum (20 μg mL−1) displayed a near constant relaxation time of 2200 ms over a period of 24 h, confirming the stability of the suspension.
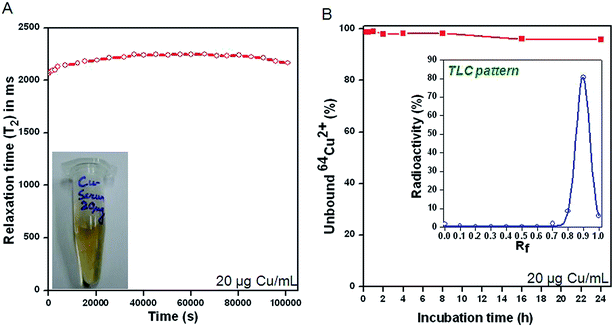 |
| Fig. 4 Stability of CuCl2 in serum medium. (A) Measurement of relaxation time over a period of 24 h. (B) Percentage of 64CuCl2 as free 64Cu2+ ions in serum medium over a period of 24 h. Inset shows the TLC pattern of 64CuCl2 in serum medium when developed in 0.1 M sodium citrate solution. | |
The stability of 64CuCl2 at a concentration ≤20 μg mL−1 was further confirmed by radio-TLC assay. As indicated from the radio-TLC pattern (inset of Fig. 4B), 64Cu remained in ionic form (64Cu2+) in mouse serum medium over a period of 24 h. Thus it could be confirmed that 64CuCl2 at a concentration ≤20 μg Cu per mL did not lead to aggregation of serum proteins incorporating 64Cu over a prolonged period of 24 h.
Biodistribution studies in tumor bearing mice
The uptake of 64CuCl2 produced by (n, γ) route in different organs/tissues of C57BL/6 mice bearing melanoma tumors and Swiss mice bearing fibrosarcoma tumors expressed as % ID per g at different p.i. times is shown in Fig. 5A and 6A. The results of the biodistribution studies revealed significant uptake of 64CuCl2 in both melanoma as well fibrosarcoma tumors. In mice bearing melanoma tumors, tumor uptake of 5.8 ± 1.1% ID per g was observed within 1 h p.i., which increased to 7.6 ± 1.7% ID per g at 4 h p.i. The uptake in fibrosarcoma tumor was slightly lower, wherein a maximum tumor uptake of 6.5 ± 1.4% ID per g was observed at 4 h p.i. For both melanoma and fibrosarcoma tumor bearing mice, initial accumulation of activity was observed in various non-target organs viz. liver, GIT, stomach, lungs etc. However, with the progress of time, the uptake in non-target organs gradually reduced. The tumor-to-blood, tumor-to-muscle and tumor-to-liver ratios of 64CuCl2 at different time points p.i. are shown in Fig. 5B and 6B. In melanoma tumor bearing mice, the tumor-to-muscle ratio was observed to increase from 12.7 ± 1.2 at 1 h p.i. to 24.9 ± 1.8 at 24 h p.i., while the tumor-to-liver and tumor-to-blood ratios increased from 0.12 ± 0.07 to 0.62 ± 0.23 and 2.92 ± 0.65 to 13.27 ± 1.98, respectively, between the same time points. Similarly, in fibrosarcoma tumor bearing mice, the tumor-to-muscle, tumor-to-liver ratio and tumor-to-blood ratios were observed to increase from 10.11 ± 2.22 to 21.63 ± 1.77, 0.09 ± 0.04 to 0.75 ± 0.43, 2.05 ± 0.63 to 18.41 ± 1.68, respectively, between 1 h to 24 h p.i.
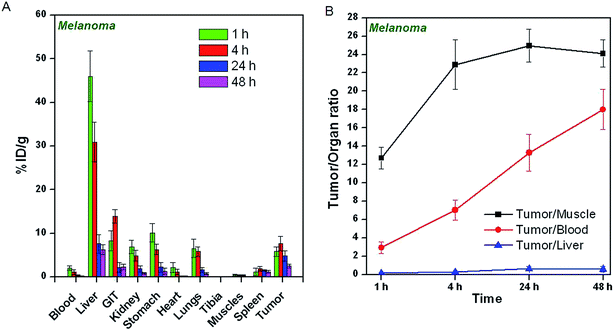 |
| Fig. 5 Biodistribution of 64CuCl2 in C57BL/6 mice bearing melanoma tumors. (A) Uptake of 64CuCl2 in different organs/tissues at different time points p.i. (B) The tumor-to-blood, tumor-to-muscle and tumor-to-liver ratios of 64CuCl2 at different time points p.i. | |
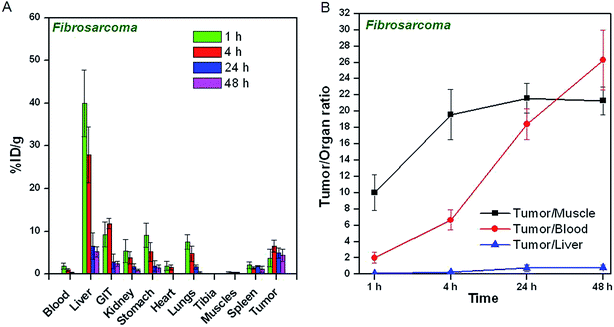 |
| Fig. 6 Biodistribution of 64CuCl2 in Swiss mice bearing fibrosarcoma tumors. (A) Uptake of 64CuCl2 in different organs/tissues at different time points p.i. (B) The tumor-to-blood, tumor-to-muscle and tumor-to-liver ratios of 64CuCl2 at different time points p.i. | |
As a control experiment, 64Cu–NOTA complex was administered in fibrosarcoma and melanoma tumor bearing mice and biodistribution studies were carried out at 4 h p.i. Since, maximum tumor uptake on administration of 64CuCl2 was observed at 4 h p.i. in both the tumor models, this time point was chosen for the control studies. The uptake of 64Cu–NOTA in different organs/tissues of C57BL/6 mice bearing melanoma tumors and fibrosarcoma tumors at 4 h p.i. times is shown in Fig. 7. The figure also provides a comparison of uptakes of 64Cu–NOTA and 64CuCl2 in same animal models at 4 h p.i. Uptakes of negatively charged 64Cu–NOTA complex21 in melanoma and fibrosarcoma tumors at 4 h p.i. were 0.12 ± 0.11% ID per g and 0.09 ± 0.08% ID per g, respectively, which are negligible compared to uptake of free 64CuCl2 (as positively charged Cu2+ ions) in the same tumor models (Fig. 7). As expected, 64Cu–NOTA complex administered in mice rapidly cleared through renal route.
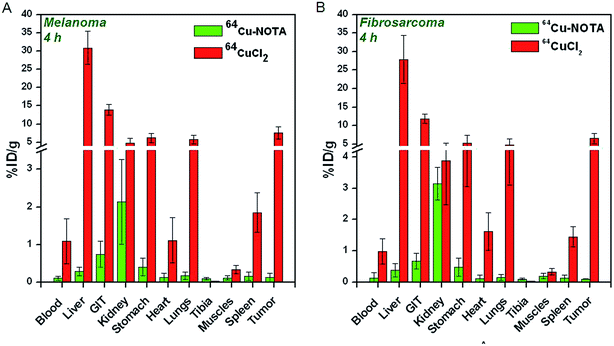 |
| Fig. 7 Comparison of biodistribution patterns of 64Cu–NOTA and 64CuCl2 at 4 h p.i. in (A) C57BL/6 mice bearing melanoma tumors, (B) Swiss mice bearing fibrosarcoma tumors. | |
In order to validate that the biological efficacy of low specific activity 64CuCl2 produced by (n, γ) route in a research reactor is comparable to that of NCA 64CuCl2 produced by standard method in a cyclotron, the results of the biodistribution studies carried out in mice bearing melanoma tumors were compared with the results reported by Qin et al. (Fig. 8).14 In the reported method, biodistribution studies were carried out using NCA 64CuCl2 in the same animal model.14 The biodistribution patterns were compared at 4 h p.i. since the tumor uptake was highest at this time point. It can be seen from Fig. 8 that the biodistribution pattern of low specific activity 64CuCl2 produced by (n, γ) route is comparable to that of NCA 64CuCl2. The radioactivity cleared from the biological system through both hepatobiliary as well as renal route. These results amply demonstrate the suitability of 64CuCl2 produced by (n, γ) route as a radiotracer for PET imaging.
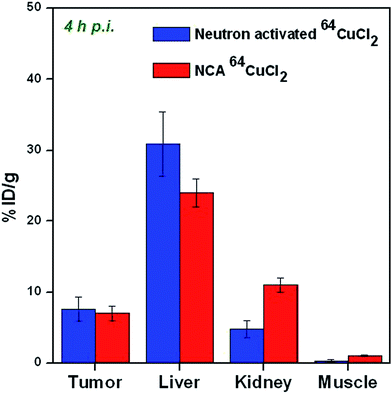 |
| Fig. 8 Comparison of biodistribution patterns of neutron activated 64CuCl2 and NCA 64CuCl2 at 4 h p.i. in C57BL/6 mice bearing melanoma tumors. | |
Discussion
Copper is an essential micronutrient which plays a vital role in a variety of biochemical processes, which include cell proliferation, angiogenesis and tumor growth.7,14,22 Over the last few years, several studies have explored copper metabolism as an imaging biomarker and increased copper uptake was observed in various types of cancerous tissues.22 The increased uptake of copper ions in cancerous lesions could be attributed to human copper transporter 1 (CTR1), a 190-amino-acid protein of 28 kDa with 3 transmembrane domains.12,14 In fact, CTR1 is the primary protein responsible for copper transport in mammalian cells.14 This protein is found to be overexpressed in a variety of cancer cells, and 64CuCl2 has been used as a probe for non-invasive PET imaging of various types of tumor xenografts in preclinical studies.11,12,14,22 Such results hold tremendous promise as they provide evidence of the potential utility of simple injection of 64Cu2+ ions for non-invasive visualization of tumors. Direct utilization of 64CuCl2 would be much cheaper than the conventionally used PET probes involving complex targeting ligands and can easily be prepared while adhering to strict regulatory guidelines.
This strategy of direct utilization of neutron activated 64CuCl2 as a PET radiotracer would especially be advantageous for countries with limited cyclotron facilities for radioisotope production. The International Atomic Energy Agency (IAEA) database provides a summary of approximately 251 research reactors currently operate worldwide.10 Fifty of these research reactors have thermal neutron flux >1 × 1014 n cm−2 s−1, and the thermal flux of an additional 85 reactors ranges from 1 × 1012 to 1 × 1014 n cm−2 s−1. Seventy eight of the above reactors are already involved in radioisotope production, and these reactors have a good geographic distribution. Many of these research reactors can be used for production of neutron activated 64Cu, which in turn would increase the global availability of this radioisotope.
Considering the cost of the target material for 64Cu production, it can be expected that use of neutron activated 64Cu would be much more cost-effective for clinical PET imaging studies where 64CuCl2 can directly be used as a radiotracer. It is reported that when 64Cu is produced by 64Ni(p, n)64Cu reaction in a cyclotron, ∼55 mg of enriched (>95%) 64Ni target is required to produce ∼22.2 GBq (600 mCi) of 64Cu.6 The cost of 1 g of enriched (>95%) 64Ni target is ∼$ 32
000.23 Therefore, based on the cost of target material, the estimated cost of ∼37 GBq (1 Ci) of 64Cu produced in a cyclotron is ∼$ 3200. For countries with limited cyclotron facilities and which have to depend on imported 64Cu to meet their research and clinical needs, the real cost of 64Cu would be much higher if the decay loss of the radioactivity during transit is taken into consideration. On the contrary, adopting our method, 64Cu can be produced by neutron activation of natural CuO target which is much cheaper (∼$ 600 for 1 g CuO).24 The specific activity of neutron activated 64Cu is ∼3 TBq (80 Ci) g−1. Based on similar considerations, the cost of ∼37 GBq (1 Ci) of 64Cu produced by neutron activation route in a medium flux research reactor is <$ 10. Thus, neutron activation route for production of 64Cu is especially advantageous for routine clinical use in developing countries which have operational research reactors for radioisotope production.
It is pertinent to point out that much lower specific activity of neutron activated 64Cu (∼3 TBq g−1) compared to that of NCA 64Cu (3700–11
100 TBq g−1)6 might appear as a cause of concern especially when administered in human subjects in an uncomplexed form. It is well known that excess Cu is potentially hazardous to human health since it can participate in the Fenton reaction, producing radical species which can lead to oxidative stress and subsequent oxidative damage to proteins, lipids and nucleic acids in the biological system.25–27 However, for PET imaging in human subjects with good contrast, only 185–259 MBq (5–7 mCi) of 64CuCl2 is expected to be administered.28 Even while considering specific activity of 64Cu as low as 740 MBq (20 mCi) mg−1 due to decay losses during transportation of the radioisotope to distant user sites, the radioactive solution would not contain >0.5 mg Cu ions. It is reported that the cytotoxic effects of Cu ions are manifested at concentration ≥7.42 mg L−1.29,30 Assuming that the volume of volume of blood in an average adult varies between 4 and 5 L, the concentration of Cu2+ ions in human blood when administered for PET imaging would be much lower than the cytotoxic limit. However, for therapeutic procedures wherein larger doses need to be administered, detailed dosimetry and toxicity studies would be required before human administration.
Another important issue concerning the use of low specific activity 64CuCl2 as an imaging probe is the possibility of aggregation of serum proteins in presence of Cu2+ ions.19 In case there is an aggregation of serum protein incorporating 64Cu after in vivo administration of 64CuCl2, a high uptake of the radioactivity would be observed in reticuloendothelial system (RES). The radioactivity incorporated protein aggregates would rapidly be cleared through the hepatobiliary route and negligible tumor uptake would be observed. The aggregation of serum proteins in presence of Cu2+ ions was not of much concern in the earlier studies with cyclotron produced NCA 64CuCl2 (ref. 14 and 22) wherein the concentration of Cu2+ ions administered in vivo was extremely low. In order to investigate the possibility of aggregation of serum proteins on administration of low specific activity 64CuCl2 in biological system, DLS studies after addition of different concentrations of non-radioactive CuCl2 in serum medium were carried out. The results of these studies indicated that aggregate formation would be manifested only at a concentration >20 μg Cu per mL of serum. Since a healthy mouse generally has ∼1 mL of blood, a maximum amount of 20 μg of Cu corresponding to 14.8 MBq (0.4 mCi) of 64Cu (assuming specific activity as low as 740 MBq (20 mCi) mg−1 due to decay losses) can be administered which is adequate for small animal PET imaging.31,32 Based on similar considerations, administration of ∼185 MBq (5 mCi) 64CuCl2, which is adequate for PET imaging in human subjects,28 would not result in aggregation of serum proteins in vivo. Thus, low specific activity 64CuCl2 produced through (n, γ) route in nuclear reactors can safely be used as a PET probe in clinical studies.
Ex vivo biodistribution studies after administration of 64CuCl2 in tumor bearing mice showed that the radiotracer accumulated in the tumor rapidly and a decent tumor-to-background contrast could be achieved with 4 h p.i. Negligible tumor uptake of negatively charged 64Cu–NOTA complex along with rapid clearance of the radioactivity through renal route validated that the increased tumor uptake of 64CuCl2 was indeed due to cellular uptake of free 64Cu2+ ions. Though in vivo PET imaging could not be carried out due to unavailability of small animal PET imaging facility in our research centre, the results of this study amply demonstrated that neutron activated 64CuCl2 is an effective PET probe for non-invasive detection of various types of cancers. The only limitation of this radiotracer is that it might not be suitable for detection of primary cancer or its metastasis in the abdominal region because of the excretion of most 64CuCl2 from the liver to the intestinal tract through the bile ducts.14 The biodistribution pattern observed after administration of neutron activated 64CuCl2 in tumor bearing mice was comparable to that with NCA 64CuCl2.14 It is envisaged that the promising results obtained in this study would set the stage for widespread utilization of neutron activated 64CuCl2 in clinical context.
Conclusions
In this study, we established the feasibility of using low specific activity 64CuCl2 produced by (n, γ) route, as a cost-effective radiotracer for non-invasive visualization of tumors by PET. A viable strategy for large-scale production of 64Cu with high radionuclidic purity and acceptable specific activity for use as a PET radiotracer was developed by careful optimization of irradiation parameters. In vitro studies revealed that 64CuCl2 remains in ionic form in the biological system at doses suitable for PET imaging in both preclinical as well as clinical settings. Biodistribution studies carried out in mice bearing melanoma and fibrosarcoma tumors revealed rapid and significant tumor uptake with a satisfactory tumor-to-background ratio achieved within 4 h p.i. of 64CuCl2. The practicality of low specific activity 64CuCl2 as a PET radiotracer was found to be comparable to its NCA counterpart in terms of tumor targeting efficacy and pharmacokinetic profile, which demonstrated the enormous potential of neutron activated 64Cu to become the radionuclide of choice for future PET procedures, especially in countries with limited cyclotron facilities.
Acknowledgements
Research at Bhabha Atomic Research Centre is a part of ongoing activities of the Department of Atomic Energy, India and is fully supported by government funding. The authors are grateful to Dr K. L. Ramakumar, Director, Radiochemistry and Isotope Group, Bhabha Atomic Research Centre for his valuable support to this program. Thanks are due to Mr K. C. Jagadeesan and Dr S. V. Thakare for arranging the irradiation of the CuO targets in the Dhruva reactor of our research centre.
References
- M. D. Farwell, A. S. Clark and D. A. Mankoff, JAMA Oncology, 2015, 1, 421–422 CrossRef PubMed.
- A. Mahajan, V. Goh, S. Basu, R. Vaish, A. J. Weeks, M. H. Thakur and G. J. Cook, Clin. Radiol., 2015, 70, 1060–1082 CrossRef CAS PubMed.
- G. C. Pereira, M. Traughber and R. F. Muzic Jr, BioMed Res. Int., 2014, 2014, 231090 Search PubMed.
- R. Weissleder and M. J. Pittet, Nature, 2008, 452, 580–589 CrossRef CAS PubMed.
- S. M. Ametamey, M. Honer and P. A. Schubiger, Chem. Rev., 2008, 108, 1501–1516 CrossRef CAS PubMed.
- D. W. McCarthy, R. E. Shefer, R. E. Klinkowstein, L. A. Bass, W. H. Margeneau, C. S. Cutler, C. J. Anderson and M. J. Welch, Nucl. Med. Biol., 1997, 24, 35–43 CrossRef CAS.
- A. Niccoli Asabella, G. L. Cascini, C. Altini, D. Paparella, A. Notaristefano and G. Rubini, BioMed Res. Int., 2014, 2014, 786463 Search PubMed.
- T. Bokhari, A. Mushtaq and I. Khan, J. Radioanal. Nucl. Chem., 2010, 284, 265–271 CrossRef CAS.
- K. V. Vimalnath, A. Rajeswari, V. Chirayil, P. L. Sharad, K. C. Jagadeesan, P. V. Joshi and M. Venkatesh, J. Radioanal. Nucl. Chem., 2011, 290, 221–225 CrossRef CAS.
- International Atomic Energy Agency. Operation Research Reactors in the World [database]. available at: http://www.naweb.iaea.org/napc/physics/research_reactors/database/RR%20Data%20Base/datasets/foreword_home.html.
- K. I. Kim, S. J. Jang, J. H. Park, Y. J. Lee, T. S. Lee, K. S. Woo, H. Park, J. G. Choe, G. I. An and J. H. Kang, J. Nucl. Med., 2014, 55, 1692–1698 CrossRef CAS PubMed.
- F. Peng, X. Lu, J. Janisse, O. Muzik and A. F. Shields, J. Nucl. Med., 2006, 47, 1649–1652 CAS.
- F. Peng, O. Muzik, J. Gatson, S. G. Kernie and R. Diaz-Arrastia, J. Nucl. Med., 2015, 56, 1252–1257 CrossRef PubMed.
- C. Qin, H. Liu, K. Chen, X. Hu, X. Ma, X. Lan, Y. Zhang and Z. Cheng, J. Nucl. Med., 2014, 55, 812–817 CrossRef CAS PubMed.
- R. Chakravarty, S. Chakraborty, A. Dash and M. R. A. Pillai, Nucl. Med. Biol., 2013, 40, 197–205 CrossRef CAS PubMed.
- Y. Zhang, H. Hong, J. W. Engle, J. Bean, Y. Yang, B. R. Leigh, T. E. Barnhart and W. Cai, PLoS One, 2011, 6, e28005 CAS.
- D. P. Fairhurst and S. Prescott, Spectrosc. Eur., 2011, 23, 13–16 CAS.
- J. Cetnar, Ann. Nucl. Energy, 2006, 33, 640–645 CrossRef CAS PubMed.
- W. Bal, M. Sokołowska, E. Kurowska and P. Faller, Biochim. Biophys. Acta, 2013, 1830, 5444–5455 CrossRef CAS PubMed.
- P. A. Hassan, S. Rana and G. Verma, Langmuir, 2015, 31, 3–12 CrossRef CAS PubMed.
- R. Ferdania, D. J. Stigersb, A. L. Fiamengoa, L. Weia, B. T. Y. Lib, J. A. Golenc, A. L. Rheingoldd, G. R. Weismanb, E. H. Wong and C. J. Anderson, Dalton Trans., 2012, 41, 1938–1950 RSC.
- A. Duatti, Nucl. Med. Biol., 2015, 42, 215–218 CrossRef CAS PubMed.
- Isoflex product catalog (http://www.isoflex.com/nickel-64). accessed on September 28, 2015.
- Sigma-Aldrich product catalog. (http://www.sigmaaldrich.com/catalog/product/aldrich/450812?lang=en%26region=IN). accessed on September 28, 2015.
- J. Y. Uriu-Adams and C. L. Keen, Mol. Aspects Med., 2005, 26, 268–298 CrossRef CAS PubMed.
- M. Valko, C. J. Rhodes, J. Moncol, M. Izakovic and M. Mazur, Chem.–Biol. Interact., 2006, 160, 1–40 CrossRef CAS PubMed.
- A. Gupte and R. J. Mumper, Cancer Treat. Rev., 2009, 35, 32–46 CrossRef CAS PubMed.
- A. Pfeifer, U. Knigge, T. Binderup, J. Mortensen, P. Oturai, A. Loft, A. K. Berthelsen, S. W. Langer, P. Rasmussen, D. Elema, E. von Benzon, L. Hojgaard and A. Kjaer, J. Nucl. Med., 2015, 56, 847–854 CrossRef CAS PubMed.
- C. A. Grillo, M. A. Reigosa and M. A. de Mele, Contraception, 2010, 81, 343–349 CrossRef CAS PubMed.
- C. A. Grillo, M. A. Reigosa and M. F. Lorenzo de Mele, Mutat. Res., 2009, 672, 45–50 CAS.
- R. Chakravarty, S. Goel, H. Hong, F. Chen, H. F. Valdovinos, R. Hernandez, T. E. Barnhart and W. Cai, Nanomedicine, 2015, 10, 1233–1246 CrossRef CAS PubMed.
- R. Hernandez, A. Czerwinski, R. Chakravarty, S. A. Graves, Y. Yang, C. G. England, R. J. Nickles, F. Valenzuela and W. Cai, Eur. J. Nucl. Med. Mol. Imaging, 2015, 42, 1859–1868 CrossRef CAS PubMed.
Footnote |
† These authors contributed equally. |
|
This journal is © The Royal Society of Chemistry 2015 |
Click here to see how this site uses Cookies. View our privacy policy here.