DOI:
10.1039/C5RA16077D
(Paper)
RSC Adv., 2015,
5, 84746-84753
Preparation and characterization of PEG-g-MWCNTs/PSf nano-hybrid membranes with hydrophilicity and antifouling properties
Received
10th August 2015
, Accepted 29th September 2015
First published on 29th September 2015
Abstract
In this study, polysulfone (PSf) nano-hybrid membranes have been successfully fabricated by loading modified multi-walled carbon nanotubes (MWCNTs) as the dispersed phase using the phase inversion method. MWCNTs were chemically functionalized with polyethylene glycol (PEG) to achieve better dispersion in the polymer matrix. PEG-g-CNTs were confirmed by ATR-FTIR and XPS spectroscopy. The hybrid membranes showed better hydrophilicity, higher pure water flux and more excellent antifouling properties. The results demonstrated that the membrane containing 1.5 wt% PEG-g-MWCNTs showed the lowest contact angle (53.9°) and the highest water flux (71.26 L m−2 h−1) which was significantly higher than that of the pure PSf membrane and MWCNTs/PSf membrane. In BSA filtration analysis, the modified MWCNTs led to significant improvement of the fouling recovery ratio (FRR) and dramatic reduction of the adsorption amount of BSA. In addition, membranes exhibited excellent tensile strength after the addition of PEG-g-MWCNTs.
1. Introduction
Polysulfone (PSf), a widely used membrane material, has broad application prospects because of its excellent thermal stability, good chemical resistance and oxidation resistance,1,2 especially in ultrafiltration membrane separation processes. Nevertheless, the inherent hydrophobic characteristic of PSf ultrafiltration membranes makes them susceptible to irreversible fouling due to the nonspecific adsorption of proteins on the surface of the membranes in separation processes.3 In general, the favorable hydrophilicity provides better fouling resistance because lots of membrane foulants have hydrophobic properties. Therefore, many approaches were applied to improve hydrophilicity to membranes such as grafting of hydrophilic monomers onto the membrane surface,4 interfacial polymerization with hydrophilic monomers,5 embedding with nanoparticles6 or hydrophilic monomers and polymers.7
In recent years, nanoparticles have attracted considerable attention as advanced reinforcing fillers to enhance membrane properties,8–10 such as nanosilver, nanoclay, and nanosized Fe3O4, TiO2, SiO2.11–15 As novel carbon materials, carbon nanotubes (CNTs) have attracted worldwide scientific and technological interest.16 With unique properties such as excellent mechanical properties, high surface area and good hydrophilicity, CNTs has become the ideal component for reinforcing mechanical properties and increasing the porosities of composite membranes.17,18,26 However, there are many problems in preparing mixed matrix membranes using CNTs, such as bad dispersion of CNTs in organic solvents and different polymers as well as weak interface interaction between the nanofiller and the polymer matrix.19,20 Thus, chemical surface modification is a favorite application to improve the dispersion ability of CNTs to fabricate a homogeneous nanocomposite membrane. At the same time, CNTs can be easily modified and attached different hydrophilic functional groups by using chemical modification for uniform dispersion of nanotubes in the polymer matrix.21–23
Accordingly, many monomer (function group) are currently being investigated as host components such as polyacrylic acid (PAA), acrylic acid (AA), grafted on MWCNTs surface by in situ polymerization.24 Results showed that PAA modified MWCNTs/PES membrane had more efficient salt rejection and better antifouling properties. Mansourpanah et al.25 prepared polycaprolactone-modified multiwalled carbon nanotubes (PCL-MWCNTs) to fabricate PES/PCL-MWCNTs nanocomposite membranes. The results indicated that the porosity of nanocomposite membranes was increased, the fouling tendency was declined and the removal property of membranes relative to Cd2+ was significantly enhanced. Vatanpour et al.26 reported that the amine-functionalized multiwalled carbon nanotubes (NH2-MWCNTs)/PES membranes had a better hydrophilicity and water flux, which led to significant improvement of the fouling recovery ratio of the nanocomposite membranes.
In this research, via the “grafting to” method, MWCNTs were functionalized with PEG, which improve both the hydrophilicity of CNTs and the compatibility between CNTs and PSf matrix. Then the PEG-g-MWCNTs/PSf nanocomposite membranes were successfully fabricated by loading PEG-g-MWCNTs as the dispersed phase using immersion–precipitation phase transformation. A series of experiments was carried out for membrane characterization. The obtained PEG-g-MWCNTs/PSf hybrid membrane showed good hydrophilicity, antifouling capability and mechanical strength.
2. Materials and methods
2.1 Materials
Polysulfone was purchased from Dalian PSf Plastic Co. Ltd China. N,N-dimethylacetamide (DMAc, ≥99.5%, reagent) was purchased from Tianjin Kemiou Chemical Reagent Co. Ltd China. Bovine serum albumin (BSA) was purchased from Beijing Solarbio Science & Technology Co. Ltd China. HNO3 (67%) and H2SO4 (98%) were purchased from Tianjin Guangfu Fine Chemical Research Institute, China. The pristine multiwalled carbon nanotubes (p-CNTs) which have a diameter less than 8 nm and a length ranging from 10 to 30 μm were purchased from Chengdu institute of organic chemicals Co. Ltd China academy of science.
2.2 Functionalization of MWCNTs
1.5 g pristine MWCNTs were oxidized with a mixture of H2SO4/HNO3 (3
:
1 in vol%) in a 250 ml round bottom flask equipped with a condenser. The mixture was heated to 70 °C for 24 h with magnetic stirring. The solution was diluted with deionized water to reach neutral pH and filtered through a 0.22 μm membrane. Then the products MWCNTs-COOH were dried at 55 °C in vacuum for 12 h.
The next reaction converts carboxylic acid to an acyl chloride group. The resulted MWCNTs-COOH were refluxed with thionyl chloride (SOCl2) at 70 °C for 24 h to obtain more reactive intermediate products of MWCNTs-COCl. Afterwards, the precipitate was refluxed with polyethylene glycol (molecular weight of PEG chains = 400) at 120 °C for 48 h to generate the final product PEG-g-MWCNTs. A graphical illustration of PEG-g-MWCNTs preparation steps is depicted in Fig. 1.
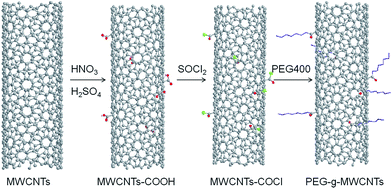 |
| Fig. 1 Schematic steps of preparation of PEG-g-MWCNTs. | |
2.3 Preparation of PEG-g-MWCNTs/PSf nano-hybrid membranes
All the PEG-g-MWCNTs/PSf hybrid membranes were prepared by the classical phase inversion method27 with DMAc as the solvent and distilled water at room temperature as the nonsolvent coagulation bath. A solution were prepared by dispersing different weight percentages of PEG-g-MWCNTs (0–3 wt%, the mass ratio, based on the weight of PSf) in DMAc and ultrasonicated (80 W) at room temperature for 1 h. Then PSf (15 wt%, the mass ratio) was dissolved in PEG-g-MWCNTs/DMAc solution at 70 °C and stirred for more than 6 h to obtain a uniform casting solution. After cooling to room temperature, the solution was degassed in the vacuum oven (25 °C) for at least 4 h to remove air bubbles before membrane casting. The cast solution was spread into liquid film on a glass plate with 300 μm gap and then the cast film was immersed into distilled water coagulation bath (at 25 °C). The formed membranes were peeled off from the glass plates, subsequently rinsed with distilled water to remove the residual solvent. The membranes were preserved in distilled water until they were used in the characterization tests. In addition, the pure PSf membrane, p-CNTs/PSf membrane and different percentages of MWCNTs-COOH/PSf hybrid membranes were prepared with the same method as control groups.
2.4 Characterization of PEG-g-MWCNTs
The morphologies of the p-CNTs and PEG-g-MWCNTs were observed using high resolution-transmission electron microscopy (HR-TEM; JEM-2010). Samples were dispersed by sonication in ethanol and then deposited on 200-mesh copper grids.
Fourier transform infrared spectroscopy (FTIR, TENSOR37, BRUKER, Germany) was used for validation of PEG formation on MWCNTs surface and the spectra of the p-CNTs and PEG-g-MWCNTs were compared.
X-ray photoelectron spectroscopy (XPS, K-Aepna, EDAX, America) was used for analyzing the changes of chemical species on the surface of MWCNTs before and after grafting.
2.5 Characterization of PEG-g-MWCNTs/PSf nano-hybrid membranes
2.5.1 Morphology observation. The cross-sectional structures of the membranes were examined by a field emission scanning electron microscope (FE-SEM, S-4800, HITACHI). Samples of each membranes were firstly frozen in liquid nitrogen and fractured, and then were coated with a thin layer of gold before being observed by FE-SEM.
2.5.2 Contact angle measurements. The hydrophilicity of membranes was investigated by measuring the water contact angle. The contact angle between water and membrane surface was measured with a contact-angle analysis device (DSA100, KRUSS, Germany). Each contact angle was measured three times at three different points of each sample and the average value was derived.
2.5.3 Permeation flux and rejection measurements. Water permeation flux and rejection of the membranes were measured at 25 °C by lab-made filtration experimental equipments. The rejection tests were carried out with an aqueous solution of BSA (molecular weight = 68
000) (1 g L−1). All experiments were conducted under the feed pressure of 0.1 MPa. The membranes were pre-pressured at 0.1 MPa for 30 min before measurement to get a steady water flux; then the water permeation and rejection for the BSA solution were measured (at least 5 readings were collected to obtain an average value.) The concentrations of BSA in the permeation and feed solution were determined by an UV-spectrophotometer (UV-2450, Shimadzu, Japan). The permeation flux and rejection were defined as formulae (1) and (2), respectively.28 |
 | (1) |
|
 | (2) |
where J is the permeation flux of the membrane for pure water (L m−2 h−1), V is the volume of permeate pure water (L), A is the effective area of the membrane (m2) and ΔT is the permeation time (h). R was the rejection to BSA (%). CP and CF were the concentrations of BSA in the permeate and feed solution, respectively (wt%).
2.5.4 Porosity and mean pore size calculation. The membrane porosity (ε) was measured by gravimetric method. The membrane soaked with distilled water was weighed after mopping superficial water with filter paper. Then, the wet membrane was dried in an oven at 60 °C before measuring the dry weight. The average pore radius [r (m)] is determined by the filtration velocity method, using Guerout–Elford–Ferry equation. The membrane porosity [ε (%)] and average pore radius [r (m)] were defined as formulae (3) and (4), respectively.29 |
 | (3) |
|
 | (4) |
where W1 is the weight of the wet membrane (g), W2 is the weight of the dry membrane (g), d is the membrane thickness (cm), A is the effective area of the membrane (cm2) and ρw is the water density (0.998 g cm−3). η is the water viscosity (8.9 × 10−4 Pa s), Q is the volume of the permeate pure water per unit time (m3 s−1) and ΔP is the operation pressure (0.1 MPa).
2.5.5 Analysis of membrane fouling. First, distilled water passed through the membrane for 120 min at an operation pressure of 0.2 MPa, and the water flux (Jw,1) reached a stable stage. Second, 1.0 mg ml−1 BSA solution (pH = 7.0 ± 0.1) were filtrated for 120 min at the same pressure (0.2 MPa), and the flux for protein solution (Jp) was determined. After ultrafiltration of BSA solution, the membranes were simply washed with deionized water for 20 min, and then the pure water flux of cleaned membranes (Jw,2) was measured again. The flux recovery ratio (FRR) was calculated by the following equation:30 |
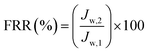 | (5) |
The protein adsorption experiment was carried out with BSA. The membrane samples (2 cm × 2 cm) were rinsed for 3 times with phosphate buffer solution (PBS) and then immersed into PBS solution containing BSA (1.0 mg ml−1) were incubated at 25 °C for 24 h. After the BSA adsorption process, the samples were removed. The protein concentrations before and after the adsorption were measured by UV-vis spectrophotometer (Shimadzu UV-2450, Japan) at 280 nm. The adsorption amounts of BSA were calculated by comparing the absorption intensity variation. The amounts of the adsorbed protein of membrane were calculated by the following equation:30
|
 | (6) |
where
M refers to the amount of the adsorbed protein on the membrane surface (μg cm
−2),
c0 and
c indicate the concentration of BSA in the solution before and after adsorption (mg ml
−1), respectively.
v denotes the volume of adsorption solution (ml) and
s is the external surface area of the membranes (cm
2).
2.5.6 Tensile strength measurements. The tensile strength of the membranes was determined at room temperature using an electronic single fiber strength tester (LLY-06, LAIZHOU ELECTRON INSTRUMENT Co. Ltd China) with the tensile rate: 10 mm min−1. For each specimen, five runs were performed and then averaged. The highest and lowest values were discarded, and the remaining measurements were averaged.
3. Results and discussion
3.1 Characterization of PEG-g-MWCNTs
The PEG-g-MWCNTs were first characterized by TEM, FTIR and XPS spectra. As shown in Fig. 2, the morphologies and structures of the p-CNTs and PEG-g-MWCNTs are observed by TEM. The results show that the p-CNTs have relatively smooth surfaces (Fig. 2a), while the PEG-g-MWCNTs have amorphous protuberances and particles (Fig. 2b).
 |
| Fig. 2 TEM images of (a) p-CNTs and (b) PEG-g-MWCNTs. | |
Fig. 3 presents the FTIR spectra of the p-CNTs and the PEG-g-MWCNTs. After surface functionalization, the peak at 1130 cm−1 and 1621 cm−1 corresponds to the C–O and C
O stretching vibration which attributed to the carboxyl groups of the MWCNTs. It is considered that the peak at 1725 cm−1 is attributed to the C
O stretching vibration of the ester carbonyl group, and the peak at 1400 cm−1 corresponds to the –OH stretching vibration of PEG for PEG-g-MWCNTs. Furthermore, the peak at 2860 and 2930 cm−1 are ascribed to the asymmetric and symmetric stretching of C–H deformation. These results preliminarily indicate the presence of significant carbonyl groups chemically attached to an ester bond, which can be confirmed that the PEG chains were chemically attached to the functional groups of MWCNTs.31,32
 |
| Fig. 3 FTIR spectra of p-CNTs and PEG-g- MWCNTs. | |
Fig. 4 displays the XPS survey of the p-CNTs and the PEG-g-MWCNTs. The XPS survey of the p-CNTs shows a strong C1s peak at 284.6 eV. Compared to the p-CNTs spectrum, PEG-g-MWCNTs shows notable changes in peak intensity and peak position which indicating the chemical reaction occurring in PEG molecules and nanotubes. The core level C1s spectra of the p-CNTs and the PEG-g-MWCNTs were shown in Fig. 5. The C1s peaks were obtained in a binding energy range from 279 to 298 eV. Fig. 5a showing two dominant peaks one centered at 284.6 eV and another at 285.2 eV can be seen in the spectrum. The peak at 287.4 eV corresponds to carbon bonded as in –C
O–. In addition, peak at 291.0 eV is attributed to the characteristic satellite for π–π* transition observed in MWCNTs materials.33 However, after grafting PEG, as shown in Fig. 5b, the intensity of peak at 285.2 eV was decreased and two new peak appeared at 286.1 eV and 288.9 eV. The two peaks correspond to carbon bonded as in –C–O– and –COO– respectively, which provide the strong evidence for the existence that the PEG molecules are covalently bound to the MWCNTs-COOH. At the same time, these results corroborate the FT-IR each other.
 |
| Fig. 4 Survey XPS spectra of p-CNTs and PEG-g-MWCNTs. | |
 |
| Fig. 5 C1 core level spectra of (a) p-CNTs and (b) PEG-g-MWCNTs. | |
3.2 Characterization of membranes
3.2.1 Cross-sectional morphological studies. According to Fig. 6, the pure PSf membrane had sponge-like structure (Fig. 6a), while all the modified membranes exhibited a very significant change on the pore structure due to the introduction of PEG-g-MWCNTs. All the membranes showed highly asymmetric structure with a dense layer formed at the top-layer. By the addition of small amount of PEG-g-MWCNTs (Fig. 6b–d), the hydrophilic nano-fillers made the exchange rate between solvent and nonsolvent rapidly which forming finger-like structure at the sub-layer because of thermodynamic and kinetic effects. In the phase inversion process, the exchange driving force of solvent and nonsolvent enhances owing to the increased hydrophilicity of the casting solution induced by hydrophilic functionalized MWCNTs.34,35 When the concentration of PEG-g-MWCNTs increased to 2.0 wt%, the viscosity of the casting solution was increased causing changes in the membranes structure, so that the finger-like pores development was suppressed, and the thickness of the sponge layer is increased(Fig. 6e and f).
 |
| Fig. 6 The cross-sectional SEM images of membranes prepared with varied weight fractions of PEG-g-MWCNTs: (a) pure PSf membrane (b) 0.5 wt% (c) 1.0 wt% (d) 1.5 wt% (e) 2.0 wt% (f) 3.0 wt%. | |
3.2.2 Contact angle. Contact angle measurements were widely used for the characterization of the hydrophilicity of membrane surfaces,36 and the results were presented in Fig. 7. The pure PSf membrane had the highest contact angle of 82.3°, and the contact angle of the p-CNTs/PSf membrane is 81.6°, which has changed a little compared with that of the pure PSf. After incorporation of hydrophilic low-dimensional carbon nanomaterials, the contact angles of the modified membranes were all decreased. It is obvious that after the addition of PEG-g-MWCNTs, the contact angle of the membranes is significantly decreased. When the content of PEG-g-MWCNTs is 1.5 wt%, a minimum contact angle of 53.9° is observed. The reason is that the large amount of hydrophilic groups on the membrane surface plays a favorable role in increasing the hydrophilicity of the membranes. However, when the PEG-g-MWCNTs content reaches 2.0 wt%, the contact angle value increased to 57.6° due to the aggregation of PEG-g-MWCNTs reducing effective surface of hydrophilic groups. In addition, compared to the membranes with MWCNTs-COOH, the membranes modified by different blend compositions of PEG-g-MWCNTs show the lower contact angle. Thus it can be confirmed that the PEG-g-MWCNTs has the better hydrophilicity.
 |
| Fig. 7 Contact angle of the pure PSf membrane, p-CNTs/PSf membrane MWNCTs-COOH/PSf membranes and PEG-g-MWCNTs/PSf membranes. | |
3.2.3 Pure water flux. Flux measurements are a convenient method for obtaining information about the permeation performance of membranes. As shown in Fig. 8, compared the flux of the pure PSf membrane (5.18 L m−2 h−1) and the p-CNTs/PSf hybrid film (8.85 L m−2 h−1), the water flux of modified membranes by MWCNTs-COOH and PEG-g-MWCNTs were enhanced respectively. When the content of the PEG-g-MWCNTs increased to 1.5 wt%, the membrane reached the highest water flux of 71.26 L m−2 h−1. The permeability improvement was attributed to membrane morphology and hydrophilicity. First, water flux has a direct relationship with the number of pores and the pore size on the membrane surface. The results presented in Table 1 revealed that the porosity of 1.5 wt% PEG-g-MWCNTs elevated to 85.68 area% from 56.35 area% for PSf and the pore size of 1.5 wt% PEG-g-MWCNTs elevated to 39.91 nm from 34.08 nm for PSf. In addition, by embedding of the nanofiller into the casting solution, the interaction between polymer chains and the nanofiller may disrupt the polymer chain packing, therefore improving water permeability due to introduction of free volumes between the polymer chains and nanofiller interface. Second, enhancement of hydrophilicity can be confirmed by the contact angle results. Therefore, the permeability of membranes enhances.
 |
| Fig. 8 Pure water flux of the pure PSf membrane, p-CNTs/PSf membrane, MWNCTs-COOH/PSf membranes and PEG-g-MWCNTs/PSf membranes. | |
Table 1 BSA rejection, porosity and pore size of different membranes
Type of membranes |
Rejection (%) |
Porosity (%) |
Pore size (nm) |
PSf |
99.9 |
56.35 |
34.08 |
p-CNTs |
98.9 |
58.82 |
34.32 |
0.5% MWCNTs-COOH |
97.3 |
61.28 |
36.99 |
1.0% MWCNTs-COOH |
95.8 |
70.79 |
37.72 |
1.5% MWCNTs-COOH |
94.1 |
76.85 |
38.55 |
2.0% MWCNTs-COOH |
95.6 |
68.98 |
36.17 |
3.0% MWCNTs-COOH |
95.2 |
68.81 |
34.65 |
0.5% PEG-g-MWCNTs |
96.8 |
69.64 |
36.29 |
1.0% PEG-g-MWCNTs |
93.1 |
78.86 |
38.28 |
1.5% PEG-g-MWCNTs |
92.2 |
85.68 |
39.91 |
2.0% PEG-g-MWCNTs |
94.7 |
73.48 |
37.25 |
3.0% PEG-g-MWCNTs |
95.7 |
62.48 |
36.86 |
3.2.4 Rejection, porosity and pore size of the membranes. BSA rejection ratio, porosity and pore size of different membranes were depicted in Table 1. Considering about BSA rejection ratio, all the membranes have more than 90% rejection ratio of BSA which indicate that the modified membranes can effectively retain the protein and organic molecules. However, when the content of PEG-g-MWCNTs increased, especially at 1.5 wt% of PEG-g-MWCNTs, the porosity and pore size of membrane were strongly increased, resulting in the decrease of BSA rejection. After the content of PEG-g-MWCNTs increased to 2.0 wt%, the rejection increased again. It is attributed to the high density of PEG-g-MWCNTs in the casting solution led to an increase in the viscosity of solution. This would prevent the exchange between solvent and non-solvent during the phase separation process and make the precipitation of the membranes slow down. As a result, a relative smaller porous membrane was formed. Moreover, the decline in hydrophobic interaction between BSA protein and hydrophilic surface of membrane might be a reason for slight increase in BSA rejection.
3.2.6 Mechanical properties of membranes. Mechanical properties of membranes were determined by the data on tensile strength of membranes. As showing in Fig. 12, the tensile strength increased from 3.51 to 5.08 MPa with the increase of PEG-g-MWCNTs content from 0.5 wt% to 2.0 wt%. This can be explained in terms of the suitability between PEG-g-MWCNTs and the polymer matrix, which is due to the excellent mechanical properties, high aspect ratio and high surface area of the nanofiller. On the other hand, the interface interaction existed between PEG-g-MWCNTs and PSf substrate, which greatly improved the compatibility between nano-filler and polymeric matrix, so that the tensile strengths of modified membranes were improved remarkably compared to the pure PSf membrane, p-CNTs/PSf membrane and the MWCNTs-COOH/PSf membranes. When the PEG-g-MWCNTs content was over 2.0 wt%, the large aggregates appeared with the increase of PEG-g-CNTs concentration, which would result in more defects and aggregation. The tensile strength decreased because there is no strong affinity between PSf and nanofiller due to the low-surface energy of PSf.
 |
| Fig. 12 Tensile strength of the pure PSf membrane, p-CNTs/PSf membrane, MWNCTs-COOH/PSf membranes and PEG-g-MWCNTs/PSf membranes. | |
4. Conclusions
PEG-g-MWCNTs were synthesized and used as nanofiller in the preparation of nano-hybrid polyethersulfone membranes. The chemical structure and surface morphology of the modified MWCNTs were characterized by ATR-FTIR, XPS and TEM. The hydrophilicity and antifouling performance of hybrid membranes were characterized using contact angle, pure water flux, BSA ultrafiltration and static protein adsorption. SEM images showed the membranes forming finger-like macrovoids in the sub-layer after the addition of PEG-g-MWCNTs. With PEG-g-MWCNTs from 0 to 1.5 wt%, contact angle of hybrid membranes declined from 82.3° to 53.9°, water flux enhanced from 5.18 L m−2 h−1 to 71.26 L m−2 h−1, flux recovery ratio improved from 41.6% to 94.1% and the adsorption amount of BSA decreased from 58.96 μg cm−2 to 41.63 μg cm−2. Moreover, the nano-hybrid membranes revealed better mechanical properties in comparison with unmodified PSf membranes. Therefore, the PEG-g-MWCNTs/PSf nano-hybrid membranes with effective hydrophilicity and antifouling performances will have potential applications on water purification.
Acknowledgements
The research was supported by the Nature Science Foundation of China (No. 21206120), the State Key Laboratory of Chemical Engineering (No. SKL-ChE-14A03).
References
- Y. Ma, F. Shi, Z. Wang, M. Wu, J. Ma and C. Gao, Desalination, 2012, 286, 131–137 CrossRef CAS PubMed.
- M. K. Sinha and M. K. Purkait, RSC Adv., 2015, 5, 22609–22619 RSC.
- R. Das, M. E. Ali, S. B. A. Hamid, S. Ramakrishna and Z. Z. Chowdhury, Desalination, 2014, 336, 97–109 CrossRef CAS PubMed.
- A. Venault, J.-R. Wu, Y. Chang and P. Aimar, J. Membr. Sci., 2014, 470, 18–29 CrossRef CAS PubMed.
- M. N. Abu Seman, M. Khayet and N. Hilal, Desalination, 2011, 273, 36–47 CrossRef CAS PubMed.
- Z. Rahimi, A. A. L. Zinatizadeh and S. Zinadini, J. Ind. Eng. Chem., 2015, 29, 366–374 CrossRef CAS PubMed.
- F. Liu, M. R. Moghareh Abed and K. Li, J. Membr. Sci., 2011, 366, 97–103 CrossRef CAS PubMed.
- A. Rahimpour and S. S. Madaeni, J. Membr. Sci., 2010, 360, 371–379 CrossRef CAS PubMed.
- C.-F. de Lannoy, E. Soyer and M. R. Wiesner, J. Membr. Sci., 2013, 447, 395–402 CrossRef CAS PubMed.
- J.-n. Shen, H.-m. Ruan, L.-g. Wu and C.-j. Gao, Chem. Eng. J., 2011, 168, 1272–1278 CrossRef CAS PubMed.
- X. Cao, M. Tang, F. Liu, Y. Nie and C. Zhao, Colloids Surf., B, 2010, 81, 555–562 CrossRef CAS PubMed.
- A. Casariego, B. W. S. Souza, M. A. Cerqueira, J. A. Teixeira, L. Cruz, R. Díaz and A. A. Vicente, Food Hydrocolloids, 2009, 23, 1895–1902 CrossRef CAS PubMed.
- P. Daraei, S. S. Madaeni, N. Ghaemi, E. Salehi, M. A. Khadivi, R. Moradian and B. Astinchap, J. Membr. Sci., 2012, 415–416, 250–259 CrossRef CAS PubMed.
- V. Moghimifar, A. E. Livari, A. Raisi and A. Aroujalian, RSC Adv., 2015, 5, 55964–55976 RSC.
- H. Wu, B. Tang and P. Wu, J. Membr. Sci., 2014, 451, 94–102 CrossRef CAS PubMed.
- E. Salehi, S. S. Madaeni, L. Rajabi, V. Vatanpour, A. A. Derakhshan, S. Zinadini, S. Ghorabi and H. Ahmadi Monfared, Sep. Purif. Technol., 2012, 89, 309–319 CrossRef CAS PubMed.
- R. Stoner, B. Brown and J. T. Glass, Diamond Relat. Mater., 2014, 42, 49–57 CrossRef PubMed.
- H. You, X. Li, Y. Yang, B. Wang, Z. Li, X. Wang, M. Zhu and B. S. Hsiao, Sep. Purif. Technol., 2013, 108, 143–151 CrossRef CAS PubMed.
- E. Celik, H. Park, H. Choi and H. Choi, Water Res., 2011, 45, 274–282 CrossRef CAS PubMed.
- Y. Shirazi, M. A. Tofighy and T. Mohammadi, J. Membr. Sci., 2011, 378, 551–561 CrossRef CAS PubMed.
- K. Balasubramanian and M. Burghard, Small, 2005, 1, 180–192 CrossRef CAS PubMed.
- S. Akbar, E. Beyou, P. Cassagnau, P. Chaumont and G. Farzi, Polymer, 2009, 50, 2535–2543 CrossRef CAS PubMed.
- S. Panahian, A. Raisi and A. Aroujalian, Desalination, 2015, 355, 45–55 CrossRef CAS PubMed.
- P. Daraei, S. S. Madaeni, N. Ghaemi, H. Ahmadi Monfared and M. A. Khadivi, Sep. Purif. Technol., 2013, 104, 32–44 CrossRef CAS PubMed.
- Y. Mansourpanah, S. S. Madaeni, A. Rahimpour, M. Adeli, M. Y. Hashemi and M. R. Moradian, Desalination, 2011, 277, 171–177 CrossRef CAS PubMed.
- V. Vatanpour, M. Esmaeili and M. H. D. A. Farahani, J. Membr. Sci., 2014, 466, 70–81 CrossRef CAS PubMed.
- J.-H. Choi, J. Jegal and W.-N. Kim, J. Membr. Sci., 2006, 284, 406–415 CrossRef CAS PubMed.
- X. Zhao, J. Ma, Z. Wang, G. Wen, J. Jiang, F. Shi and L. Sheng, Desalination, 2012, 303, 29–38 CrossRef CAS PubMed.
- M. Irfan, A. Idris, N. M. Yusof, N. F. M. Khairuddin and H. Akhmal, J. Membr. Sci., 2014, 467, 73–84 CrossRef CAS PubMed.
- L. Yu, Y. Zhang, B. Zhang, J. Liu, H. Zhang and C. Song, J. Membr. Sci., 2013, 447, 452–462 CrossRef CAS PubMed.
- Y.-S. Kim, J.-H. Cho, S. G. Ansari, H.-I. Kim, M. A. Dar, H.-K. Seo, G.-S. Kim, D.-S. Lee, G. Khang and H.-S. Shin, Synth. Met., 2006, 156, 938–943 CrossRef CAS PubMed.
- Y. Wen, H. Wu, S. Chen, Y. Lu, H. Shen and N. Jia, Electrochim. Acta, 2009, 54, 7078–7084 CrossRef CAS PubMed.
- T. I. T. Okpalugo, P. Papakonstantinou, H. Murphy, J. McLaughlin and N. M. D. Brown, Carbon, 2005, 43, 153–161 CrossRef CAS PubMed.
- V. Vatanpour, S. S. Madaeni, R. Moradian, S. Zinadini and B. Astinchap, J. Membr. Sci., 2011, 375, 284–294 CrossRef CAS PubMed.
- S. Majeed, D. Fierro, K. Buhr, J. Wind, B. Du, A. Boschetti-de-Fierro and V. Abetz, J. Membr. Sci., 2012, 403–404, 101–109 CrossRef CAS PubMed.
- J. Zhang, Z. Xu, M. Shan, B. Zhou, Y. Li, B. Li, J. Niu and X. Qian, J. Membr. Sci., 2013, 448, 81–92 CrossRef CAS PubMed.
- X. Chen, Y. Su, F. Shen and Y. Wan, J. Membr. Sci., 2011, 384, 44–51 CrossRef CAS PubMed.
|
This journal is © The Royal Society of Chemistry 2015 |