DOI:
10.1039/C5RA15656D
(Paper)
RSC Adv., 2015,
5, 77699-77705
New insights into synthesis and oligomerization of ε-lactams derived from the terpenoid ketone (−)-menthone†
Received
5th August 2015
, Accepted 4th September 2015
First published on 4th September 2015
Abstract
Two regioisomeric lactams, which are derived from terpenoid ketone (−)-menthone in two steps, are oligomerized in an easy acid-induced procedure to obtain oligoamides that contain alkyl groups and stereocenters; for this, a nucleophilic oligomerization via a non-ionic propagating site (neutral conditions) and a polycondensation are also possible. Furthermore, a regioselective synthesis is demonstrated where (−)-menthone is transformed into one of these lactams in a one-step procedure via Beckmann rearrangement without isolation of any oxime intermediate using the reagent hydroxylamine-O-sulfonic acid (HOSA). These concise oligoamide syntheses smooth the way to novel sustainable long-chain polyamides with highly interesting structures.
1. Introduction
A key task in modern macromolecular chemistry is the development of sustainable polymers from renewable resources.1,2 This is driven by depleting fossil resources and by the fact that nature can provide alternative feedstock3,4 and also – in addition – novel highly interesting building blocks for the synthesis of innovative polymer architectures for special applications.5 Famous examples for this fact are, for instance, the biobased, biodegradable and biocompatible polyester polylactide that has a lot of applications in material science and biomedicine,6 cellulose nanofibers,7 or the epoxy resins derived from epoxidized fatty acids of many plant oils.8 Furthermore, polyhydroxybutyrate (PHB), which is derived from microorganisms in its isotactic form,9 is an interesting biodegradable polymer, which meanwhile is also investigated synthetically.10 In general, a variety of polymerization methods and/or the modification of renewable starting materials can be applied for the preparation of novel sustainable polymers.11
In this context, terpenes are very interesting natural building blocks,1b,12,13 which are hydrocarbons that contain one or more carbon–carbon double bonds. Also, their synthesis and modification with novel synthetic catalytic14 and enzymatic15 methods, has became an important research field. These molecules have a carbon skeleton of isoprene (2-methyl-1,4-butadiene) units and a variety of structures and functions.16 Over the last decades, dozens of studies addressing terpene-based polymers have been published, including polymerization of myrcene,17 pinene,18 phellandrene19 or of D-limonene-derived monomers.20 Epoxidized limonene (limonene oxide) has been used for the synthesis of innovative polycarbonates,21 polyesters22 and polyurethanes.23 Terpenoid ketones L-menthone and L-carvone have been transformed to the corresponding lactones via Baeyer–Villiger oxidation and polymerized via ring-opening polymerization (ROP) to result in biorenewable polyesters with highly interesting structures.24,25
In this regard, an increasing research effort is also spent on developing new ways to sustainable polyamides. These important polymers are used for a wide range of applications from high-performance engineering thermoplasts and fibers to functional materials for biomedical applications.26 In addition to the “classical” polyamides like polyamide 66 or polyamide 6, novel biobased polyamides have been developed, based e.g. on sebacic acid,27 succinic acid,28 brassylic acid29 or abietic acid.30
Ring-opening polymerization (ROP) of ε-caprolactam for the synthesis of polyamide 6 has been investigated and applied for decades, where anionic, acid-induced or hydrolytic procedures can be used.31 Also some special procedures have been described, e.g. the initiation of polymerization by means of heterocyclic carbenes.32
ROP studies with more diversified and/or modified ε-lactams, which can be obtained from biobased terpenoid ketones, are still rare but have an immense potential: the resulting polyamides have a backbone containing side chains and stereocenters and thus have very interesting structure–properties-relationships and a high potential for different applications. Menthone is especially interesting in this context as it is – on the one hand – a renewable compound which contains alkyl residues and stereocenters, and – on the other hand – a compound whose precursor (−)-menthol can meanwhile be synthesized in a large-scale industrial process starting from the achiral and acyclic compound citral.33
The concept that also biobased terpenoid ketones can be used for lactam and polyamide synthesis provides a wide range of possibilities. Accordingly, the transformation of menthone (1) to the corresponding oxime 2 (ref. 34) and then to lactams 3a and 3b has been described some time ago;35 it was recently shown by our group that both lactams 3a and 3b can be oligomerized via anionic ROP.36,37 For this, the lactam mixture has to be purified in a time-consuming procedure, either via several crystallizations or by means of flash chromatography (FC). Therefore, the aim of this study was developing a remarkably improved lactam synthesis as well as the application of enhanced and straightforward oligomerization procedures (Scheme 1).
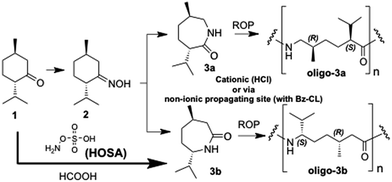 |
| Scheme 1 Synthesis of lactams 3a and 3b starting from menthone (1) via oxime 2 (top) or directly and regioselective by means of hydroxylamine-O-sulfonic acid (HOSA; bottom) and ring-opening polymerization (ROP) to oligo-3a and oligo-3b. Bz-CL = benzoylated caprolactam (initiator). | |
2. Experimental section
Materials and methods
All chemicals were purchased from commercial sources (Sigma-Aldrich, ABCR) and used as received unless otherwise noted (L-menthone from Sigma-Aldrich was obtained with 85% isomeric purity; for clarity, the impurities were isolated via FC and shown to be other stereoisomers; however, for this study menthone was used as received, and detailed purification was performed on the later lactam step). Deuterated solvents for NMR measurements were obtained from Deutero. NMR spectra were recorded on Bruker AVIII-300 and Bruker AVIII-500 NMR spectrometers in CDCl3 for monomers and in trifluoracetic acid (TFA-d) or – if required in CDCl3 after trifluoroacetylation of the amide bond – for polymers. Matrix assisted laser desorption/ionization-time of flight (MALDI-TOF) mass spectra were recorded on a Bruker Ultra Flex TOF/TOF mass spectrometer using α-cyano-4-hydroxycinammic acid or dithranol as the matrix. Gel permeation chromatography/size exclusion chromatography (GPC/SEC) measurements were performed on an Agilent 1200Series device with a HFIP gel column and hexafluoroisopropanol as the solvent and evaluated with PSS WinGPC software.
Synthesis of lactams 3a and 3b
A mixture of both lactams 3a and 3b could be obtained from menthone in two steps using a previously described procedure.36 Lactams were separated via FC (gradient hexane/ethyl acetate) to give pure 3a and 3b, respectively.
For the one-step approach to lactam 3b (“HOSA-route”, multigram scale) without isolation of oxime intermediates, the following procedure was applied:38 menthone 1 (1.1 g, 7.13 mmol) was dissolved in formic acid (3.6 mL), hydroxylamine-O-sulfonic acid (HOSA; 1.2 g, 10.6 mmol) was added and the mixture was heated under reflux for 6 h. Afterwards the reaction was quenched with H2O, neutralized with NaOH solution (5%), extracted with chloroform (three times) and dried over Na2SO4. The solvent was removed under reduced pressure, and the remaining residue was recrystallized in hexane and dried in vacuo (colorless solid; yield 93%).
3a. 1H-NMR (300 MHz, CDCl3): δ = 6.03 (bs, 1H), 3.15–2.91 (m, 2H), 2.20–2.13 (m, 2H), 2.12–1.93 (m, 1H), 1.88–1.74 (m, 1H), 1.74–1.52 (m, 1H), 1.51–1.15 (m, 2H), 0.95 (d, J = 6.4 Hz, 6H), 0.88 (d, J = 6.8 Hz, 3H); 13C-NMR (75 MHz, CDCl3): δ = 180.05, 49.71, 48.80, 38.53, 34.73, 28.04, 25.14, 21.50, 20.07, 18.94; MP = 101 °C; IR (cm−1): 3211.08, 2913.69, 1659.46.; calcd (C10H19NO): C 70.96, H 11.31, N 8.28, found: C 70.22, H 11.24 N 8.18. αD = −6.3°; 3b: 1H NMR (300 MHz, CDCl3): δ: 5.66 (bs, 1H), 3.17 (q, J = 9.5, 4.9 Hz, 1H), 2.45–2.21 (m, 2H), 2.01–1.88 (m, 1H), 1.84–1.68 (m, 3H), 1.44–1.17 (m, 2H), 0.99 (d, J = 6.7 Hz, 3H), 0.93 (dd, J = 6.9, 2.0 Hz, 6H); 13C NMR (75 MHz, CDCl3) δ: 58.88, 44.71, 39.07, 32.80, 32.36, 30.20, 24.61, 18.69, 17.90; MP 118 °C. IR (cm−1): 3212.46, 2950.58, 1655.79. Calcd (C10H19NO): C 70.96, H 11.31, N 8.28, found C 70.99, H 11.50, N 8.39. αD = −53.4.
Synthesis of amino acid 4
Following a procedure of Imoto et al.35c Mentholactam 3b (3.00 g, 17.7 mmol, 1.0 eq.) and activated charcoal (750 mg) were suspended in H2O (30 mL) and concentrated sulfuric acid (3.0 mL). The reaction mixture was stirred at 125 °C for three hours. After cooling down to room temperature, the mixture was neutralized with an excess of barium hydroxide and filtered. Removal of the solvent in vacuo provided the crude amino acid, which was first recrystallized from ethanol and then applied to a DOWEX 50 WX-2 100–200 ion exchange column. After ion exchange chromatography (H2O → 1 M NH4OH) the free amino acid (1.55 g, 8.28 mmol, 47%) was obtained as a colorless solid. 1H-NMR (300 MHz, D2O): 3.15–3.05 (m, 1H), 2.21–2.11 (dd, 1H), 2.05–1.91 (m, 2H), 1.86–1.65 (m, 2H), 1.61–1.45 (m, 1H), 1.44–1.30 (m, 1H), 1.25–1.11 (m, 1H), 1.01–0.83 (m, 9H). 13C-NMR (300 MHz, D2O): 182.91, 57.59, 45.13, 31.77, 30.76, 29.39, 26.63, 19.05, 17.33, 16.62. IR (cm−1): 3027.91, 2147.69, 1655.89, 1457.15, 1384.89. MP = 191 °C. Calcd C 64.13, H 11.30, N 7.48, found C 64.17, H 11.43, N 7.48.
Oligomerizations
For lactam oligomerization in vacuo, lactam (M = 169.26 g mol−1; 50 mg, 0.3 mmol or – for larger scale – 300 mg, 1.8 mmol) was placed in a glass ampule, and an initiator was added (for cationic polymerization: 1 μL or 3 μL of HCl from an Eppendorf pipette; for nucleophilic oligomerization via non-ionic propagating site: benzoylated caprolactam (M = 217.26 g mol−1; 3 mg; 15 μmol)). The ampule was subsequently evacuated and sealed with a torch flame and heated to 250 °C in a sand bath. After a certain time (4 h or 8 h, respectively), the ampule was opened and the monomer was washed out several times with a 1
:
1 hexane/ethyl acetate mixture. For oligomerization under argon, the equivalent procedure was performed in a sealed scintillation vial in a heating block. The remaining residue was analyzed by GPC/SEC, NMR and MALDI-MS.
For polycondensation, amino acid 4 was heated in a sealed glass ampule for 250 °C for 4 h. The monomer was washed out as described above and the remaining residue was analyzed. For copolymerization, ML and CL were mixed in the described ratios, polymerization was started with 1 mg of aminocaproic acid and work-up was performed as described for the homopolymers.
Oligo-3a. 1H-NMR (300 MHz, TFA-d): 8.01 (s, 1H), 6.61–6.82 (bs, 1H), 3.62–3.02 (m, 2H, repeating unit), 2.62–2.01 (m, 2H, repeating unit), 2.01–1.51 (m, 4H, repeating unit), 1.49–1.31 (m, 1H, repeating unit), 1.25–1.13 (m, 1H, repeating unit), 1.11–0.86 (m, 10H, repeating unit). 13C-NMR (500 MHz, TFA-d): 187.35, 56.01, 54.94, 52.89, 51.13, 33.61, 33.10, 28.36, 20.96, 20.56, 20.14, 19.80.
Oligo-3b. 1H-NMR (300 MHz, TFA-d): 6.71–6.31 (m, 1H), 3.82–4.03 (m, 1H, repeating unit), 3.50–3.41 (m, 1H, repeating unit), 2.72–2.30 (m, 1H, repeating unit), 2.22–1.75 (m, 3H, repeating unit), 1.62–1.21 (m, 3H, repeating unit), 1.10–0.75 (m, 8H, repeating unit). 13C-NMR (500 MHz, CDCl3 after trifluoroacetylation): 45.41, 32.27, 29.86, 27.39, 20.83, 19.80, 17.78; GPC/SEC data and MALDI-MS spectra are discussed in the main text.
Copolymers oligo-3b-CL (relative signal intensity depends on the copolymer composition). 1H-NMR (300 MHz, TFA-d): 3.60–3.41 (m), 2.75–2.59 (m), 2.51–2.25 (m), 1.81–1.61 (m), 1.51–1.31 (m), 1.31–1.20 (m), 1.01–0.81 (m).
3. Results and discussion
We could now show that a very fast access to lactam 3b can be accomplished using the reagent hydroxylamine-O-sulfonic acid (HOSA). This has been shown to be a reliable reagent for the synthesis of unsubstituted lactams from ketones and especially of ε-caprolactam from cyclohexanone.38 Remarkably, this transformation in formic acid leads to pure 3b directly from 1 without the necessity to isolate any oxime intermediate and/or to purify a lactam mixture. It is – to the best of our knowledge – the first time that this terpenoid ketone is directly converted in such a direct way into its corresponding lactam (Scheme 1). The 1H-NMR spectrum of 3b obtained via this fast route (“HOSA-route”) equals the one obtained via the two-step synthesis, where the oximes are isolated first and then transformed to the lactams with polyphosphoric acid (“polyphosphoric acid-route”).35c,36 Complete characterization data of all monomers are described in the ESI.†
With the aim to use these lactams for a “green” and easy synthesis of structurally significant oligoamides, we focused on a cationic (acid-induced with HCl) and a neutral (nucleophilic via non-ionic propagating site; initiated with N-benzoyl-caprolactam) procedure (Scheme 1). It is obvious that each of these procedures by itself provides matter for an own extensive study with variation of all parameters; however, the aim of this study was not to cover an extensive number of different experimental setups, but to elucidate and to emphasize the potential of these procedures and to evaluate them as alternatives to the previously described anionic procedure.36
The oligomerizations described in Scheme 1 resulted in oligoamides, while no oligomers were obtained in control experiments without adding an initiator to the lactams. The acid-induced oligomerization of 3a and 3b was performed with conc. HCl at 250 °C in vacuum and sealed glass ampules (Table 1, entry 1 and 4, which worked better than an acid-induced Schlenk procedure36) and – additionally – under argon in a sealed vial in a heating block (Table 1, entry 2 and 5). Further reactions were initiated only using benzoyl-caprolactam as the initiator in vacuum ampules (entry 3 and 6). After reaction, the remaining monomer was washed out with hexane/ethyl acetate in several washing steps and the remaining oligoamides (beige residues) were analyzed by gel-permeation chromatography (GPC/SEC), NMR and matrix-assisted laser desorption ionization mass spectrometry (MALDI-MS).
Table 1 Results of the oligomerization of mentholactams 3a and 3b via different procedures
Entry |
Lactam |
Conditions |
Mn/Mw |
Monomer conversion/yield (%) |
1 |
3a |
HCl, 4 h, vac., |
1400/1700 |
52 |
2 |
3a |
HCl, 4 h, ar. |
2400/3600 |
43 |
3 |
3a |
Bz-CL, 4 h, vac. |
1000/1100 |
26 |
4 |
3b |
HCl, 4 h, vac. |
2300/2500 |
34 |
5 |
3b |
HCl, 4 h, ar. |
1800/3400 |
31 |
6 |
3b |
Bz-CL, 4 h, vac. |
Only monomer |
— |
An increased reaction time did not result in a remarkably change of yield and oligomerization degree (data not shown). This indicates that an equilibrium between monomers and oligomers is reached after a certain time. Thus, enhancement of yield and molecular weight would require a different experimental setup (e.g. addition of monomer during reaction), which is not possible with the sealed glass ampoules described here and thus topic of ongoing investigations.
GPC/SEC measurements were performed for the analysis of the molecular weight distribution, where – due to the poor solubility of oligo-/polyamides in common solvents – hexafluoroisopropanol (HFIP) was used as the eluent. The peaks were then evaluated against a calibration curve to determine the Mn and Mw values of the oligomers (see Table 1 for the values and ESI (Fig. S1†) for elugrams).
In all entries, the GPC traces show a main peak that can be assigned to the oligo-/polymer. This peak was sometimes accompanied by a much smaller, low molecular weight peak, which can be assigned to monomer traces (see elugrams of monomers for comparison in the ESI†) or to the cyclic dimer which is known to be formed in polymerization of substituted ε-lactams and which is difficult to be removed despite of extensive washing.39 It is important to mention the difficulty of work-up in this case: sometimes this low molecular weight peak could only be removed by washing out by means of sonication, which also solved partially the oligomers (consider that these oligomers are better soluble in hexane/ethyl acetate than “normal” polyamide 6, due to their isopropyl and methyl groups in the backbone). Therefore, a “compromise” had to be found between number of washing steps and obtained yield of oligomers. With additional numbers of washing steps (optionally sonication), this low molecular weight peak could be successively minimized and/or removed (Fig. 1).
 |
| Fig. 1 GPC/SEC elugram of oligoamides (Table 1, entry 4) after three washing steps (left) and after additional three washing steps (by means of sonication; right). | |
While lactam 3a could be shown to oligomerize easier than 3b with an anionic polymerization procedure,36 this fact could not necessarily be confirmed with the acid-induced polymerization procedures described herein, as it can be seen comparing the molar mass distributions of oligo-3a and oligo-3b (Table 1). Polymerization of 3a under argon works – in terms of the oligomer length – a bit better than under vacuum (entries 1 and 2), while – at this stage – there is only a marginal difference for lactam 3b (entries 4 and 5). Interestingly, in both cases it was not possible to increase yield by increasing reaction time to 8 h (data not shown). As mentioned, also a nucleophilic oligomerization procedure via non-ionic propagating site was applied, where benzoylated caprolactam was used as initiator, which was prepared according to a literature known procedure.40 Here, a nucleophilic attack of the lactam at the endocyclic imide carbonyl carbon occurs, without formation of an ionic intermediate, followed by stepwise lactam addition.40 Applying this procedure, sterically more hindered lactam 3b (isopropyl group besides NH) could not be oligomerized under these conditions, while lactam 3a gave oligomers with Mn values of around 1000 (entry 5) when the reaction was performed in an evacuated ampule. The bimodal character of corresponding GPC trace (Fig. S1,† entry 3) is currently topic of ongoing investigations. Interestingly, neither lactam 3a nor 3b could be oligomerized so far via this neutral procedure under an argon atmosphere.
For further analysis, matrix-assisted laser desorption ionization mass spectrometry (MALDI-MS) spectra of the oligoamides were recorded (Fig. 2 and S2†). The spectra show a characteristic peak distance of the monomer unit which is 169.26 and a maximum oligomer lengths of n = 20, as well as a similar molar mass distribution than the GPC/SEC data, but also with little differences. Sometimes the GPC/SEC experiments show slightly higher MWs than the MALDI-MS spectra, which can be explained with the fact that GPC/SEC is a relative method, but also with the fact that polyamides with high MW are sometimes hard to detect in the mass spectrometer (due to unfavorable “flying behavior”), which is also known from spectra of classical polyamide 6.41
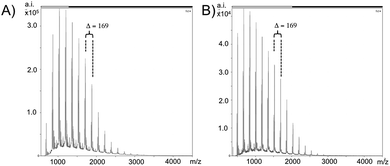 |
| Fig. 2 MALDI-MS spectra of (A) oligo-3a and (B) oligo-3b (obtained in entry 1 and 4, Table 1). | |
The NMR spectra of these oligoamides show characteristic peaks that can be assigned to the defined positions in the oligomer backbone, as exemplarily shown for entry 2 in Fig. 3 (for further NMR spectra see ESI†). Due to the poor solubility in common NMR solvents, TFA-d was used here. In comparison to the spectra of the monomers, the signals of the oligomers blur a bit due to the different chain lengths present. For determination of the oligomerization degree, the spectra have limited significance, as the oligomers have only exchangeable H-atoms (NH and OH) as the “characteristic end-groups”. These can only roughly be used for end group analysis, when the measurement is done quickly after solving in solvent TFA-d (these peaks decrease by time). For the spectrum shown in Fig. 3, integration leads to an average oligomerization degree of ca. 10. Nevertheless, the GPC data described above are more reliable for the oligomer length determination in this case.
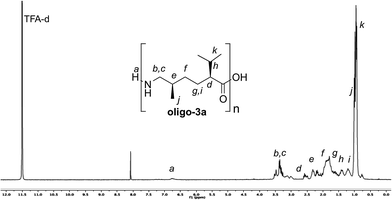 |
| Fig. 3 1H-NMR spectrum of oligo-3a (obtained in entry 2, Table 1). | |
The corresponding 13C spectra also show all significant peaks of the oligoamides (see Fig. S3–S16† for all NMR data). It is worth to mention that the tacticity of these oligomers is pre-defined by the stereochemistry of the monomers; however, further comments on tacticity based on 13C spectra cannot be made at this stage with these 13C NMR measurements in TFA-d.
The successful acid-induced ring-opening oligomerization of 3b raises the question whether – alternatively – this lactam can be efficiently ring-opened to the corresponding amino acid 4 and transformed to oligoamides/polyamides via polycondensation (Scheme 2).
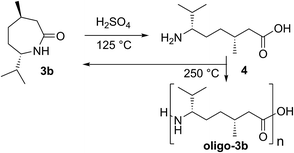 |
| Scheme 2 Ring-opening of 3b to 4 and polycondensation of 4 to oligo-3b. | |
According to a literature known procedure,35c we obtained free amino acid 4 by treatment of 3b with concentrated sulfuric acid/H2O and charcoal. After recrystallization, pure 4 was isolated via an ion exchange column and fully characterized (ESI†). Indeed, heating 4 to 250 °C in a sealed glass ampule resulted in formation of oligoamides, as determined by GPC/SEC and MALDI. However, the main part of 4 (ca. 90%) was shown to recyclize to lactam 3b, as determined by comparing the corresponding NMR spectra. A series of polycondensation experiments with 4 applying different reaction times showed that the low yield and an oligomer lengths of approx. Mn/Mw = 2500/2900 do not change with time; thus, as expected, improvement here would also require a modified experimental setup (maybe also for removing the water formed during reaction) (Fig. 4).
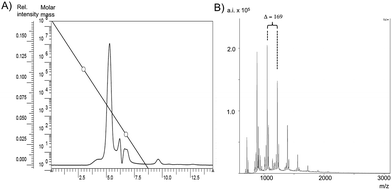 |
| Fig. 4 (A) GPC/SEC elugram and (B) MALDI-MS spectrum of oligo-3b obtained via the polycondensation experiment described in text. Diagonal line in (A) shows calibration masses and limits of calibration curve (white circles). | |
Copolymerization behavior of lactams with different polymerization abilities is another important issue in this context.37 Thus, we have performed further experiments addressing the ability of key compound 3b to be (random) copolymerized with caprolactam (CL; Scheme 3).
 |
| Scheme 3 Copolymerization of 3b and CL. | |
After acid-induced copolymerization, remaining monomers were washed out several times as described above, and the film-like beige residue was analyzed. As expected, 3b shows lower polymerization ability than CL,37 which could be demonstrated impressively by the corresponding NMR spectra. For instance, its copolymerization in a ratio 3b
:
CL 3
:
7 leads to a polymer with Mn/Mw = 5200/14
300 (PDI = 2.7; Fig. 5A). Here, only 10 mol% of 3b are incorporated into the polymer backbone, as determined by relative integration of the peaks in the corresponding spectrum (Fig. 5B; for this, CL-specific signal at 3.55 (representing 2H) and 3b-specific signal at 0.9 (representing 9H) were used). Decreasing the feed ratio to 3b
:
CL 1
:
9 leads to a copolymer with only 4 mol% 3b incorporated, while a feed ratio of 1
:
1 leads to 22 mol% of incorporated 3b (Fig. S20†).
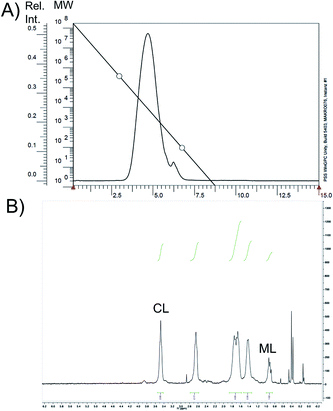 |
| Fig. 5 (A) Elugram and (B) 1H-NMR spectrum of copolymer oligo-3b-CL (feed 3 : 7). ML = menthone-derived lactam 3b. | |
4. Conclusion
In this study, the two regioisomeric substituted ε-lactams, which are derived from the terpenoid ketone (−)-menthone, could be successfully oligomerized via both acid-induced and neutral ROP procedures. We have also demonstrated that one of these lactams can be regioselectively synthesized directly from L-menthone using reagent HOSA. Thus, concepts that are used for the synthesis of “classical” polyamide 6 (polycaprolactam, Perlon®) were successfully transferred to highly interesting biobased lactams which are derived from a terpenoid precursor. Oligoamides could be obtained via ROP and polycondensation. A relation was shown between the applied conditions and the oligomerization behavior. The polymerizations stopped on the “oligo-level” despite monomer present, which is assumed to be caused e.g. by precipitation from bulk. Thus, variations of the monomer/initiator ratio, polymerizations in solution, optimizations of these procedures for the synthesis of higher MW menthone-based polyamides and corresponding copolymers as well as determination of the thermoanalytical data and the exact role of the side groups and stereocenters within the polymer backbone are topics of ongoing investigations. For the future, the investigation of kinetic effects in terms of hydrolysis and polymerization of these modified lactams will also be an important issue.
Notes and references
-
(a) R. Mülhaupt, Macromol. Chem. Phys., 2013, 214, 159–174 CrossRef PubMed;
(b) P. A. Wilbon, F. Chu and C. Tang, Macromol. Rapid Commun., 2013, 34, 8–37 CrossRef CAS PubMed;
(c) K. Yao and C. Tang, Macromolecules, 2013, 46, 1689–1712 CrossRef CAS;
(d) A. Gandini, Macromolecules, 2008, 41, 9491–9504 CrossRef CAS;
(e) C. K. Williams and M. A. Hillmyer, Polym. Rev., 2008, 48, 1–10 CrossRef CAS PubMed;
(f) M. A. R. Meier, J. O. Metzger and U. S. Schubert, Chem. Soc. Rev., 2007, 36, 1788–1802 RSC.
- A. Gandini, Green Chem., 2011, 13, 1061–1083 RSC.
- P. T. Anastas and N. Eghbali, Chem. Soc. Rev., 2010, 39, 301–312 RSC; P. T. Anastas and M. M. Kirchhoff, Acc. Chem. Res., 2002, 35, 686–694 CrossRef CAS PubMed.
- R. A. Sheldon, Chem. Commun., 2008, 3352–3365 RSC.
-
(a) S. A. Miller, ACS Macro Lett., 2013, 2, 550–554 CrossRef CAS;
(b) U. Biermann, U. Bornscheuer, M. A. R. Meier, J. O. Metzger and H. J. Schäfer, Angew. Chem., Int. Ed., 2011, 50, 3854–3871 CrossRef CAS PubMed.
-
(a) A. P. Gupta and V. Kumar, Eur. Polym. J., 2007, 43, 4053–4074 CrossRef CAS PubMed;
(b) A.-C. Albertsson and I. K. Varma, Biomacromolecules, 2003, 4, 1466–1486 CrossRef CAS PubMed.
- S. J. Eichhorn, A. Dufresne, M. Aranguren, N. E. Marcovich, J. R. Capadona, S. J. Rowan, C. Weder, W. Thielemans, M. Roman, S. Renneckar, W. Gindl, S. Veigel, J. Keckes, H. Yano, K. Abe, M. Nogi, A. N. Nakagaito, A. Mangalam, J. Simonsen, A. S. Benight, A. Bismarck, L. A. Berglund and T. Peijs, J. Mater. Sci., 2010, 45, 1–33 CrossRef CAS.
-
(a) U. Biermann, W. Friedt, S. Lang, W. Lühs, G. Machmüller, J. O. Metzger, M. Rüsch gen Klaas, H. J. Schäfer and M. P. Schneider, Angew. Chem., Int. Ed., 2000, 39, 2206–2224 CrossRef CAS;
(b) H. Baumann, M. Bühler, H. Fochem, F. Hirsinger, H. Zoebelein and J. Falbe, Angew. Chem., Int. Ed., 1988, 27, 41–62 CrossRef PubMed.
-
(a) Y. Doi, Microbial Polyesters, Wiley-VCH, Weinheim, Germany, 1990 Search PubMed;
(b) G. A. M. van der Walle, G. J. M. de Koning, R. A. Weusthuis and G. Eggink, Properties, Modifications and Applications of Biopolyesters, Advances in Biochemical Engineering/Biotechnology, ed. T. Scheper, Springer, Berlin, Germany, 2001, vol. 71, p. 263 Search PubMed.
-
(a) R. Reichardt and B. Rieger, Adv. Polym. Sci., 2012, 245, 49–90 CrossRef CAS (chapter in: Synthetic Biodegradable Polymers, Springer ISBN 978-3-642-27153-3);
(b) M. Zintl, F. Molnar, T. Urban, V. Bernhart, P. Preishuber-Pflügl and B. Rieger, Angew. Chem., Int. Ed., 2008, 47, 3458–3460 CrossRef CAS PubMed.
-
(a) K. Schröder, K. Matyjaszewski, K. J. T. Noonan and R. T. Mathers, Green Chem., 2014, 16, 1673–1686 RSC;
(b) R. T. Mathers and M. A. R. Meier, Green Polymerization Methods:
Renewable Starting Materials Catalysis and Waste Reduction, Wiley-VCH, Weinheim, 2011 Search PubMed.
- M. Winnacker and B. Rieger, ChemSusChem, 2015, 8, 2455–2471 CrossRef CAS PubMed.
- J. Zhao and H. Schlaad, Adv. Polym. Sci., 2013, 253, 151–190 CrossRef CAS.
- Q. Zhang and K. Tiefenbacher, Nat. Chem., 2015, 7, 197–202 CrossRef CAS PubMed.
- M. Hofer, H. Strittmatter and V. Sieber, ChemCatChem, 2013, 5, 3351–3357 CrossRef CAS PubMed; L. O. Wiemann, C. Faltl and V. Sieber, Z. Naturforsch., B: J. Chem. Sci., 2012, 67, 1056–1060 Search PubMed.
- A. Corma, S. Iborra and A. Velty, Chem. Rev., 2007, 107, 2411–2502 CrossRef CAS PubMed.
- J. M. Bolton, M. A. Hillmyer and T. R. Hoye, ACS Macro Lett., 2014, 3, 717–720 CrossRef CAS.
-
(a) N. A. Kukhta, I. V. Vasilenko and S. V. Kostjuk, Green Chem., 2011, 13, 2362–2364 RSC;
(b) For pinene-derived lactam, see H. K. Hall Jr, J. Org. Chem., 1963, 28, 3213–3214 CrossRef.
- D. Dakshinamoorthy, A. K. Weinstock, K. Damodaran, D. F. Iwig and R. T. Mathers, ChemSusChem, 2014, 7, 2923–2929 CrossRef CAS PubMed.
-
(a) M. Firdaus and M. A. R. Meier, Green Chem., 2013, 15, 370–380 RSC;
(b) M. Firdaus, L. M. de Espinosa and M. A. R. Meier, Macromolecules, 2011, 44, 7253–7262 CrossRef CAS.
-
(a) F. Auriemma, C. de Rosa, M. R. Di Caprio, R. Di Girolamo, W. Chadwick Ellis and G. W. Coates, Angew. Chem., Int. Ed., 2015, 54, 1215–1218 CrossRef CAS PubMed;
(b) C. M. Byrne, S. D. Allen, E. B. Lobkovsky and G. W. Coates, J. Am. Chem. Soc., 2004, 126, 11404–11405 CrossRef CAS PubMed.
- C. Robert, F. de Montigny and C. M. Thomas, Nat. Commun., 2011, 2, 586, DOI:10.1038/ncomms1596.
- M. Bähr, A. Bitto and R. Mülhaupt, Green Chem., 2012, 14, 1447–1454 RSC.
-
(a) J. Shin, Y. Lee, W. B. Tolman and M. A. Hillmyer, Biomacromolecules, 2012, 13, 3833–3840 CrossRef CAS PubMed;
(b) J. Shin, M. T. Martello, M. Shrestha, J. E. Wissinger, W. B. Tolman and M. A. Hillmyer, Macromolecules, 2011, 44, 87–94 CrossRef CAS;
(c) C. L. Wanamaker, L. E. O'Leary, N. A. Lynd, M. A. Hillmyer and W. B. Tolman, Biomacromolecules, 2007, 8, 3634–3640 CrossRef CAS PubMed;
(d) D. Zhang, M. A. Hillmyer and W. B. Tolman, Biomacromolecules, 2005, 6, 2091–2095 CrossRef CAS PubMed.
-
(a) J. R. Lowe, M. T. Martello, W. B. Tolman and M. A. Hillmyer, Polym. Chem., 2011, 2, 702–708 RSC;
(b) J. R. Lowe, W. B. Tolman and M. A. Hillmyer, Biomacromolecules, 2009, 10, 2003–2008 CrossRef CAS PubMed.
- For a good overview see:
(a) G. W. Becker, D. Braun, L. Bottenbruch and R. Binsack, Polyamide (Kunstoff Handbuch; 3/4), Hanser Verlag, Munich, Germany, 1998 Search PubMed;
(b) R. S. Lenk, J. Polym. Sci., Part D: Macromol. Rev., 1978, 13, 355–387 CrossRef CAS PubMed.
- L. Jasinska, M. Villani, J. Wu, D. van Es, E. Klop, S. Rastogi and C. E. Koning, Macromolecules, 2011, 44, 3458–3466 CrossRef CAS.
- I. Bechthold, K. Bretz, S. Kabasci, R. Kopitzky and A. Springer, Chem. Eng. Technol., 2008, 31, 647–654 CrossRef CAS PubMed.
- A. Prieto, I. Iribarren and S. Muñoz-Guerra, J. Mater. Sci., 1993, 28, 4059 CrossRef CAS.
- S. J. Kim, B. J. Kim, D. W. Jang, S. H. Kim, S. Y. Park, J.-H. Lee, S.-D. Lee and D. H. Choi, J. Appl. Polym. Sci., 2001, 79, 687–695 CrossRef CAS.
-
(a) S. Russo, E. Casazza, Polymer Science: A Comprehensive Reference, 2012, vol. 4, pp. 331–396 Search PubMed;
(b) W. E. Hanford, US Patent 2241322, 1941.
-
(a) S. Naumann, S. Epple, C. Bonten and M. R. Buchmeiser, ACS Macro Lett., 2013, 2, 609–612 CrossRef CAS;
(b) S. Naumann, F. G. Schmidt, M. Speiser, M. Böhl, S. Epple, C. Bonten and M. R. Buchmeiser, Macromolecules, 2013, 46, 8426–8433 CrossRef CAS.
-
(a) Press release: https://www.basf.com/group/corporate/en/news-and-media-relations/science-around-us/menthol/story, accessed: July 2014;
(b) Press release: BASF adds L-menthol to product range, http://www.aroma-ingredients.basf.com/About_us/AboutLmenthol.aspx, accessed: May 2014.
- N. Kumar, K. Nepali, S. Sapra, K. R. V. Bijjem, R. Kumar, O. P. Suri and K. L. Dhar, Med. Chem. Res., 2012, 21, 531–537 CrossRef CAS.
-
(a) S. Lochyński, J. Kułdo, B. Frackowiak, J. Holband and G. Wójcik, Tetrahedron: Asymmetry, 2000, 11, 1295–1302 CrossRef;
(b) N. Komatsu, S. Simizu and T. Sugita, Synth. Commun., 1992, 22, 277–279 CrossRef CAS PubMed;
(c) M. Imoto, H. Sakurai and T. Kono, J. Polym. Sci., 1961, 50, 467 CrossRef CAS PubMed;
(d) For a related lactam, see also H. Strittmatter, C. Falcke, M. Wölbing and V. Sieber, Fraunhofer IGB Annual Report 2014/2015, pp. 92–93.
- M. Winnacker, S. Vagin, V. Auer and B. Rieger, Macromol. Chem. Phys., 2014, 215, 1654–1660 CrossRef CAS PubMed.
- For a copolymerization study see:
(a) J. Kondelíková, J. Šejba, Z. Černý and J. Králiček, Makromol. Chem., Rapid Commun., 1980, 1, 35–39 CrossRef PubMed;
(b) J. Kondelíková, J. Šejba, J. Králiček and Z. Smrčková, Die Angewandte Makromolekulare Chemie, 1980, 88, 103–111 CrossRef PubMed.
- G. A. Olah and A. P. Fung, Synthesis, 1979, 537–538 CrossRef CAS.
- H. K. Hall Jr, J. Am. Chem. Soc., 1958, 80, 6404–6409 CrossRef.
- M. Rothe and E. W. Reinold, Makromol. Chem., Rapid Commun., 1991, 12, 313–317 CrossRef CAS PubMed.
- For mass spectrometry data of conventional nylon-6 oligomers in general see:
(a) L. Shan, R. Murgasova, D. M. Hercules and M. Houalla, J. Mass Spectrom., 2001, 36, 140–144 CrossRef CAS PubMed;
(b) H. Choi, E. K. Choe, E. K. Yang, S. Jang and C. R. Park, Bull. Korean Chem. Soc., 2007, 28, 2354–2358 CrossRef CAS.
Footnote |
† Electronic supplementary information (ESI) available. See DOI: 10.1039/c5ra15656d |
|
This journal is © The Royal Society of Chemistry 2015 |
Click here to see how this site uses Cookies. View our privacy policy here.