DOI:
10.1039/C5RA09499B
(Paper)
RSC Adv., 2015,
5, 55252-55261
Ultrasonic-assisted biodiesel production from waste cooking oil over novel sulfonic functionalized carbon spheres derived from cyclodextrin via one-step: a way to produce biodiesel at short reaction time†
Received
20th May 2015
, Accepted 11th June 2015
First published on 11th June 2015
Abstract
In this study, a novel sulfonated carbon catalyst was synthesized via the one-step hydrothermal carbonization of cyclodextrin, hydroxyethylsulfonic acid and citric acid. Ultrasonic-assisted biodiesel production from waste cooking oil in the presence of the catalyst was investigated. The novel catalyst was characterized by BET, XRD, PSD, SEM-EDS, TGA, FT-IR, XPS and TPD. The catalyst exhibited a high acidity of up to 1.87 mmol g−1. 2k factorial and Box–Behnken designs were applied to find the optimum conditions to obtain a maximum fatty acid methyl ester (FAME) yield. The results of the optimization imply that a catalyst loading of 11.5 wt%, a reaction time of 8.8 min and a reaction temperature of 117 °C provide a maximum FAME yield of up to 90.8% in ultrasonic-assisted biodiesel production. The reusability of the catalyst was studied for 4 cycles under the optimum conditions and the results showed that the regenerated catalyst can be reused without any serious reduction of the FAME yield. Kinetic studies showed that the reaction followed first order reaction kinetics with an activation energy of 11.64 kJ mol−1.
1 Introduction
The current declining reserves of fossil fuels are a concern due to growing environmental and technological needs. Recently, biofuels are considered to be one of the next generation of alternative fuels because they can generally be found around the world, and also utilized easily.1,2 Biodiesel is a kind of renewable energy for diesel engines, which are required for transportation. The advantages of biodiesel are that it is a biodegradable, non-toxic, and eco-friendly fuel.3 The potential feedstocks include edible oils and non-edible oils.4 The conventional edible oils have been identified as palm oil, soybean oil and sunflower oil. However, waste cooking oil and non-edible oils such as Jatropha oil and rubber seed oil are more attractive as their use does not affect food consumption.5 For example, about 7% of edible oil supplies were applied for biodiesel production in 2007, leading to a food versus fuel issue. As a result, waste cooking oil was used in this work as the raw material for biodiesel production as its use does not rely on the food supply, unlike the reported literature.6–8 Unfortunately, because waste cooking oil has a high amount of free fatty acids (FFAs), it is not compatible with a wide range of base catalysts. It is well known that saponification can occur between FFAs and alkali catalysts to form soap and water.9 To solve this problem, acid catalysts should be used. The conventional homogeneous acid catalysts that are mostly used for biodiesel production are HCl and H2SO4. However, the use of these catalysts causes many problems: (1) reactor corrosion, (2) a large amount of waste water and (3) difficulty reusing the catalysts, resulting in an increase of the overall cost of biodiesel production.10,11 Recently, Lee and Yoo12 reported that heterogeneous catalysis was the most important technology in chemical industry as well as other environmental, energy applications, etc. Heterogeneous acid catalysts are offered as an optimum solution because they can eliminate issues such as corrosion, toxicity and separation. They can also be reused. Recently, heterogeneous acid catalysts have been reported such as sulfonated zirconia, aminophosphonic acid resin D418, Amberlyst-15, SO3H-SBA-15 and heteropolyacid catalysts.13–17 Some catalysts are not suitable due to their low catalytic activity, low stability and high cost. Acid activated carbons derived from sugar and synthesized by sulfonation are regarded to have good catalytic performance for esterification. They contain soft aggregates of polycyclic aromatic hydrocarbons compared to carbon materials which are directly obtained from lignocellulose.18 Usually, the sulfonic groups can be easily leached from the structure when the reaction is carried out at high temperature (>100 °C). For these reasons, glucose was used as the carbon precursor for the preparation of the acid carbon catalyst. It was investigated and found that carbon material derived from glucose can form as a rigid structure composed of small polycyclic aromatic carbons (a 3D sp3-bonded structure) by hydrothermal carbonization. The hydrothermal carbonization method has many advantages such as it is very cheap, mild, and absolutely “green” as it involves no catalyst, surfactant or organic solvent. Cyclodextrin (CD), which has the cyclic oligosaccharide structure of R-D-glucopyranose, is a new carbon precursor and catalyst to catalyze the transesterification. It can be transformed into aromatic carbon spheres via hydrothermal carbonization.19 The conventional surface modifications of carbon materials involve treatment with acids or ozone, thereby generating functionalities such as carboxylic acids, sulfuric acid and hydrochloric acid. In general, sulfonated carbon–glucose is synthesized via two steps. In the first step, glucose is incompletely carbonized at high temperature for a long time. Then, sulfonation is used to introduce sulfonic acid groups in the second step. However, this method requires harsh conditions for the inactive surface, is environmentally unfriendly, has a low product yield and results in numerous harmful wastes. Here, a novel catalyst was synthesized via the one-step hydrothermal carbonization of CD, hydroxyethylsulfonic acid and citric acid. The sulfonic groups can be directly connected to the surface of the carbon during the carbonization process. Based on the structure of the CD precursor, we believed that it would have a higher catalytic activity and stability than the conventional carbon catalysts used for transesterification.
Ultrasonic irradiation technique has been shown to increase the reaction rate of transesterification by an order of magnitude due to a drastic increase in the interfacial area and improved heat/mass transfer phenomena and thermal/specific of mixing.20,21 Ultrasound waves generate cavitation bubbles as they pass through the liquid. Ji et al.22 investigated the influences of sonication, mechanical stirring and hydrodynamic cavitation on biodiesel production from soybean oil catalyzed by KOH. They found that the ultrasonic method provided a shorter reaction time and a higher biodiesel yield when compared with conventional mechanical stirring. Stavarache et al.23 also reported that the ultrasonic method gave better results such as lower reaction time, lower reaction temperature, lower catalyst loading and higher FAME yield, resulting from the immiscible liquids and intensified reaction. Moreover, Choedkiatsakul et al.24 studied a further improvement for the transesterification of palm oil by the incorporation of mechanical stirring in the ultrasonic reactor. Recently, many researchers have studied biodiesel production from edible-oils and non-edible oils by using heterogeneous catalysts with the sonication method.25 Shahraki et al.26 reported that the sono-synthesis of biodiesel from soybean oil was achieved with catalysis by KF/γ-Al2O3. Pukale et al.27 studied the transesterification of waste cooking oil in the presence of a heterogeneous solid catalyst. The optimum conditions with a catalyst concentration of 3 wt% K3PO4 and a reaction time of 90 min gave the highest FAME yield of up to 92.0%. Kumar et al.28 reported that the ultrasonic-assisted transesterification of Jatropha curcus oil using a Na/SiO2 catalyst was an environmentally, ecologically and economically friendly process. To date, very few studies have focused on ultrasonic-assisted biodiesel production from waste cooking oil, especially using heterogeneous catalysts.29 No study has been reported on biodiesel production over a sulfonated solid carbon derived from cyclodextrin (SO3H-CD) with an ultrasonic system. The main objective of this work was to optimize the ultrasonic-assisted biodiesel production via a single-step process using a SO3H-CD catalyst. The experimental design was investigated.30 A 2k factorial design was used to screen the significant factors. Response surface methodology (RSM) was applied to find the levels of optimum conditions using a Box–Behnken design. The SO3H-CD catalyst was characterized by BET, XRD, PSD, SEM-EDX, TGA, FT-IR, XPS, NH3-TPD and titration. The catalyst reusability was studied. Moreover, a kinetic model was investigated and the kinetic parameters were determined by fitting the model with the experimental results.
2 Experimental
2.1 Materials
Waste cooking oil was obtained from local restaurants in Thailand. The properties of the waste cooking oil are shown in Table S1.† Hydroxyethylsulfonic acid was obtained from the synthesis of mercaptoethanol and 5 vol% H2O2 via oxidation reaction. Cyclodextrin (≥98% reagent grade), methanol (99.8% reagent grade), citric acid (≥99.5% reagent grade), sulfuric acid (95–98% AR grade) and methyl heptadecanoate (≥99% reagent grade) were purchased from Sigma-Aldrich.
2.2 Catalyst preparation
Firstly, 10 g of CD, 3 g of hydroxyethylsulfonic acid, 5 g of citric acid and 80 mL of DI water were mixed and stirred at ambient temperature for 1 h. The mixture solution was added to a Teflon-lined stainless steel autoclave and heated at 180 °C for 4 h. Then, the black slurry was filtered, washed with 1000 mL of DI water and dried at 105 °C to obtain the sulfonated solid carbon. The sulfonated solid carbon derived from CD was initialized as SO3H-CD catalyst.
2.3 Characterization of the catalyst
The Brunauer–Emmett–Teller (BET) surface area and the Barrett–Joyner–Hallenda (BJH) pore size of the catalyst were measured by N2 adsorption and desorption at the liquid nitrogen temperature of −196 °C. The crystalline structure of the catalyst was analyzed by powder X-ray diffraction (XRD). The particle-size distribution (PSD) of the catalyst was measured by a particle size analyzer (Malvern/Mastersizer X). The morphology of the catalyst was characterized by a scanning electron microscope equipped with energy dispersive spectroscopy (EDS). The thermal stability of the catalyst was determined by thermo-gravimetric analysis (TGA). The FT-IR spectra of the sulfate group in the catalyst were measured using a PerkinElmer Spectrum 100 FTIR spectrometer. The graphitization degree was accessed by X-ray photoelectron spectroscopy (XPS). The acid density of the catalyst was analyzed by NH3-temperature programmed desorption (NH3-TPD) and titration.
2.4 Experimental procedure
The transesterification reaction was performed in a 250 mL three-neck round bottom flask equipped with an ultrasonic probe, a condenser and a thermocouple thermometer (Fig. S1†). In each experiment, the frequency of the ultrasonic probe and the stirring speed were fixed at 25 kHz and 800 rpm, respectively. The effects of catalyst loading (5–15 wt%), reaction time (2–14 min), reaction temperature (50–150 °C) and molar ratio of methanol to oil (20
:
1–40
:
1) on the FAME yield were investigated using statistical analysis design. After the transesterification reaction was finished, the spent catalyst was separated from the mixture by centrifugation. The filtrate was separated into two layers using a separation funnel. Rotary evaporation was performed on the upper layer or FAME to remove the remaining methanol and water. Finally, the obtained FAME was filtered with sodium sulfate before gas chromatography (GC) analysis. The FAME product was determined using GC (Agilent 7820A), equipped with a flame ionization detector and a capillary column (DB-WAX, 30 m × 0.25 mm × 0.25 μm), following the standard method of EN 14103 with the use of methyl heptadecanoate (C17:0) as an internal standard.
The percentage of FAME yield was calculated as follows in eqn (1):
|
 | (1) |
where Σ
A is the total peak area of the FAME product,
AEI is the peak area of C17:0,
CEI is the concentration of C17:0 (mg mL
−1),
VEI is the amount of C17:0 (mL),
Ws is the weight of the sample (mg),
WP is the weight of the produced FAME (mg) and
WO is the weight of used oil.
2.5 Catalyst reusability
After the reaction was finished, the spent catalyst was washed with acetone to remove glycerin and any undesired materials attached on the surface. The spent catalyst was regenerated by soaking with conc. H2SO4. To study the long-term stability of the catalyst, it was reused for up to 4 cycles. The regenerated catalyst was compared with the catalyst without regeneration using the response of the FAME yield.
2.6 Experimental design
The purpose of the design was to investigate the significance of process factors and to optimize the biodiesel production over the SO3H-CD catalyst by employing a single-step process under ultrasonic conditions.
A 24 factorial design was used to screen the significant factors and to increase the model accuracy. Four factors such as catalyst loading (X1), reaction time (X2), reaction temperature (X3) and the molar ratio of methanol to oil (X4) were designed consisting of 16 experiments with their low and high values. The ranges of full factorial design of each factor are shown in Table 1. The regression model for screening the factors was expressed as follows in eqn (2):
|
 | (2) |
where
Y is the FAME yield (%),
β0 is the constant coefficient,
βi is the coefficient for the linear effect,
βij is the coefficient for the interaction effect and
Xi and
Xj are the factor codes.
Table 1 24 factorial design analysis of each factor and the obtained FAME yielda
Run |
X1 (wt%) |
X2 (min) |
X3 (°C) |
X4 (mol/mol) |
FAME yield (%) |
The factors are coded as follows: X1 = catalyst loading (wt%), X2 = reaction time (min), X3 = reaction temperature (°C) and X4 = molar ratio of methanol to oil (mol/mol). |
1 |
5 (−1) |
2 (−1) |
50 (−1) |
20 : 1 (−1) |
20.9 |
2 |
15 (1) |
2 (−1) |
50 (−1) |
20 : 1 (−1) |
49.7 |
3 |
5 (−1) |
14 (1) |
50 (−1) |
20 : 1 (−1) |
54.1 |
4 |
15 (1) |
14 (1) |
50 (−1) |
20 : 1 (−1) |
59.9 |
5 |
5 (−1) |
2 (−1) |
150 (1) |
20 : 1 (−1) |
69.7 |
6 |
15 (1) |
2 (−1) |
150 (1) |
20 : 1 (−1) |
84.6 |
7 |
5 (−1) |
14 (1) |
150 (1) |
20 : 1 (−1) |
87.8 |
8 |
15 (1) |
14 (1) |
150 (1) |
20 : 1 (−1) |
85.5 |
9 |
5 (−1) |
2 (−1) |
50 (−1) |
40 : 1 (1) |
20.2 |
10 |
15 (1) |
2 (−1) |
50 (−1) |
40 : 1 (1) |
49.1 |
11 |
5 (−1) |
14 (1) |
50 (−1) |
40 : 1 (1) |
56.5 |
12 |
15 (1) |
14 (1) |
50 (−1) |
40 : 1 (1) |
60.4 |
13 |
5 (−1) |
2 (−1) |
150 (1) |
40 : 1 (1) |
69.6 |
14 |
15 (1) |
2 (−1) |
150 (1) |
40 : 1 (1) |
84.4 |
15 |
5 (−1) |
14 (1) |
150 (1) |
40 : 1 (1) |
86.9 |
16 |
15 (1) |
14 (1) |
150 (1) |
40 : 1 (1) |
84.4 |
Investigating a Box–Behnken design required 15 experiments with three factors in this research (Table 2). The response pattern of this design was studied to find the optimum conditions together with the maximum FAME yield. Table 2 shows the ranges and levels of the factors. The Box–Behnken model for optimizing the factors was expressed as follows in eqn (3):
|
Y = β0 + β1X1 + β2X2 + β3X3 + β11X12 + β22X22 + β33X32 + β12X1X2 + β13X1X3 + β23X2X3
| (3) |
where
Y is the FAME yield (%),
β0 is the constant coefficient,
β1,
β2 and
β3 are coefficients for the linear effects,
β11,
β22 and
β33 are the coefficients for the quadratic effects,
β12,
β13 and
β23 are the coefficients for the interaction effects and
X1,
X2 and
X3 are the factor codes.
Table 2 Box–Behnken design for 3 factors with experimental and predicted FAME yieldsa
Run |
X1 (wt%) |
X2 (min) |
X3 (°C) |
FAME yield (%) |
Experimental |
Predicted |
The factors are coded as follows: X1 = catalyst loading (wt%), X2 = reaction time (min) and X3 = reaction temperature (°C). |
1 |
5 (−1) |
2 (−1) |
100 (0) |
55.6 |
58.8 |
2 |
15 (1) |
2 (−1) |
100 (0) |
72.1 |
71.5 |
3 |
5 (−1) |
14 (1) |
100 (0) |
70.5 |
71.1 |
4 |
15 (1) |
14 (1) |
100 (0) |
83.7 |
80.5 |
5 |
5 (−1) |
8 (0) |
50 (−1) |
53.2 |
51.7 |
6 |
15 (1) |
8 (0) |
50 (−1) |
65.9 |
68.1 |
7 |
5 (−1) |
8 (0) |
150 (1) |
78.3 |
76.1 |
8 |
15 (1) |
8 (0) |
150 (1) |
80.3 |
81.8 |
9 |
10 (0) |
2 (−1) |
50 (−1) |
63.2 |
61.5 |
10 |
10 (0) |
14 (1) |
50 (−1) |
75.4 |
76.4 |
11 |
10 (0) |
2 (−1) |
150 (1) |
85.7 |
84.8 |
12 |
10 (0) |
14 (1) |
150 (1) |
89.5 |
91.2 |
13 |
10 (0) |
8 (0) |
100 (0) |
85.3 |
85.6 |
14 |
10 (0) |
8 (0) |
100 (0) |
85.5 |
85.6 |
15 |
10 (0) |
8 (0) |
100 (0) |
85.9 |
85.6 |
2.7 Kinetic modeling
After optimization were achieved, a kinetic study on the transesterification reaction was continuously investigated by fixing of constant catalyst loading and molar ratio of methanol to oil with varying of different reaction temperatures and reaction times. The stoichiometry of the three steps and the overall transesterification reaction can be written as follows in eqn (4) and (5): |
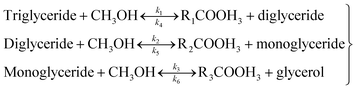 | (4) |
|
 | (5) |
From the above stoichiometry, the conversion of waste cooking oil into FAME was determined as the total mol of RCOOH3 or FAME divided by the total mol of triglycerides (eqn (6)).
|
Conversion (%) = [total mol of FAME produced/3 × (total mol of triglyceride)] × 100
| (6) |
The reaction rate was determined as follows in eqn (7):
|
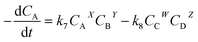 | (7) |
where
CA,
CB,
CC and
CD are the concentrations of triglyceride, methanol, glycerol and FAME, respectively.
W,
X,
Y and
Z refer to their reaction order.
k7 and
k8 are the kinetic constants for the forward and backward reaction, respectively.
From eqn (7), it can be reduced to:
|
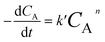 | (8) |
where
k′ =
k7CBY and/or
k′ is the modified rate constant. As the used molar ratio of methanol to oil was much higher than other components, therefore,
k7CBY can be constant. Moreover,
k7 is also much higher than
k8.
31where
CA0 and
X are the initial concentration of triglyceride and the conversion of oil or triglyceride into FAME, respectively. Therefore, the overall reaction rate can be summarized and expressed as follows in
eqn (10):
|
 | (10) |
When k′′ = k′/CA0 and n = 1, thus, eqn (10) can be rearranged and integrated to obtain the following eqn (11):
In the case that n ≠ 1, rearranging and integrating eqn (10) can obtain eqn (12):
|
(1 − X)(1−n) = 1 + (n − 1)k′′CA0nt
| (12) |
From the above equation, the best straight line was determined from the line based on the highest R2 close to 1. According to the Arrhenius equation, the reaction rate can be expressed as a function of temperature in the following eqn (13):
|
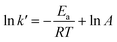 | (13) |
where
Ea is the activation energy (kJ mol
−1),
A is the pre-exponential factor (min
−1),
R is the gas constant (8.314 J K
−1 mol
−1) and
T is the reaction temperature (K).
3 Results and discussion
3.1 Characterization of the catalyst
Fig. 1A shows the XRD patterns of the catalyst. The broad diffraction peak at 10–30° was assigned to the (002) plane of the carbon in CD. After sulfonation, the mentioned (002) diffraction peak was shifted to the larger 2θ angle, suggesting that carbonization may be occurring during the sulfonation process with concentrated acid resulting in a smaller polycyclic carbon ring.19 Fig. 1B shows the particle-size distribution of the catalyst. It was found to be in the range of 0–15 μm. This result was related to the SEM image in Fig. 1C. Moreover, it was found to be a regular particle shape of spherical carbon with the existence of S element, suggesting that rich sulfonic groups attached on the surface of the catalyst. Fig. 1D shows the TGA patterns of the CD-carbon and SO3H-CD catalyst. The initial weight loss in the temperature range of 0–120 °C was due to the loss of water. The gradual weight loss of SO3H-CD at higher temperature ≥ 280 °C shows the decomposition of the sulfonic group. It should be noted that the SO3H-CD catalyst had thermal stability at temperatures close to 280 °C under oxygen-free conditions. The FTIR spectrum of the catalyst is shown in Fig. 1E. The strong bands at 1040 cm−1 and 940 cm−1 could be identified as the stretching modes of sulfate groups. This evidence indicates that the sulfuric acid resulted in extensive covalent sulfonation of the carbonized CD catalyst. Parthiban and Perumalsamy4 reported that a sulfonic acid group coupled with a carbon ring can form a stable covalent structure, leading to a good stability which is maintained at high reaction temperature. The XPS spectra are presented in Fig. 1F. The S2p photoelectron peak at 168 eV and the O1s photoelectron peak at 531.6 eV were attributed to the carbon material containing sulfonic groups. Fig. 1G shows the NH3-TPD profile of the catalyst. From the NH3-TPD profile three acid sites, a weak acid (70–150 °C), a medium acid (170–240 °C) and a strong acid (270–320 °C) were observed. The BET surface area and pore size of the SO3H-CD catalyst were determined to be 8.2 m2 g−1 and 22.1 nm, respectively (Table 3). The titration result found that the –SO3H density of the fresh catalyst was 1.87 mmol g−1. It should be noted that low surface area and large pore size can increase the accessibility of sulfuric acid into the carbon bulk, which would give a higher concentration of covalently bonded carbon with a sulfonic group. Moreover, due to the large pore size obtained, reactants such as oil and methanol can easily diffuse into the interior of the catalyst structure, resulting in an increase of catalytic activity.32–34 Shuit et al.35 reported that solid catalysts with a low surface area and narrow pore size exhibit mass transfer resistance because of the presence of a three phase system of oil, methanol and solid catalyst in the reaction mixture that limits the pore diffusion process and reduces the active sites available for the catalytic reaction, thereby decreasing the reaction rate.
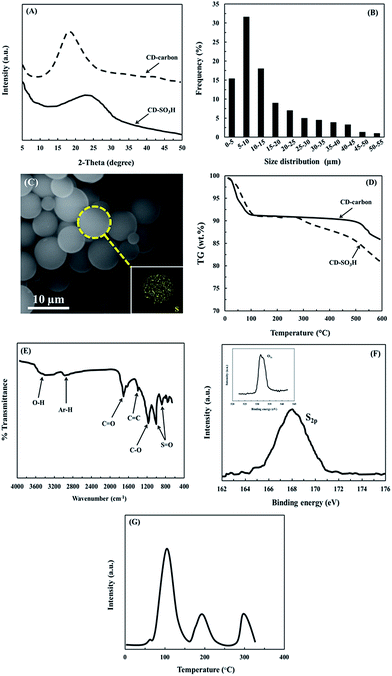 |
| Fig. 1 (A) XRD patterns, (B) particle-size distribution, (C) SEM-EDS image, (D) TGA patterns, (E) FT-IR spectrum, (F) XPS spectra and (G) NH3-TPD profiles of the catalyst. | |
Table 3 Physicochemical properties of the catalyst
CD-SO3H |
BET surface area (m2 g−1) |
Pore size (nm) |
Acidity (mmol g−1) |
S (wt%) |
C (wt%) |
O (wt%) |
After regeneration (4th reuse). Without regeneration (4th reuse). |
Fresh catalyst |
8.2 |
22.1 |
1.87 |
5.98 |
85.80 |
8.22 |
Spent catalysta |
8.4 |
18.5 |
1.85 |
5.91 |
86.23 |
7.86 |
Spent catalystb |
7.8 |
17.4 |
1.51 |
4.13 |
88.9 |
6.97 |
3.2 Ultrasonic-assisted biodiesel production with statistical analysis
Using a 24 factorial design, 16 experiments were carried out and provided the responses as experimental FAME yields (Table 1). To screen the significant factors, estimate values were plotted versus normal probability (Fig. 2). As shown in Fig. 2, it was clearly seen that catalyst loading, reaction time, reaction temperature and their interactions had a significant effect on ultrasonic-assisted biodiesel production, while the ranges of molar ratio of methanol to oil had no effect on the FAME yield. One can see that this work used the range of molar ratio of methanol to oil of 20
:
1–40
:
1, which is an excess amount, shifting the equilibrium forward to the FAME product. It should be noted that the remaining amount of methanol can be recovered from the mixture by evaporation. The X3 factor or reaction temperature had the most effect on biodiesel production, suggesting that the transesterification reaction required a high temperature due to its endothermic nature. Table S2† shows the analysis of variance (ANOVA) for the 24 factorial design. The confidence level for all stages was determined at 95%. The F-value of more than 5.32 indicated the model terms are significant. The summary of ANOVA provided a result according with the normal probability graph in Fig. 2. The regression model was determined using a significant coefficient, and the model can be expressed in terms of a linear equation as follows in eqn (14): |
Y = 63.98 + 5.77X1 + 7.955X2 + 17.63X3 − 6.42X1X2 − 2.655X1X3 − 3.42X2X3 + 0.845X1X2X3
| (14) |
where Y is the FAME yield (%), X1 is the catalyst loading (wt%), X2 is the reaction time (min) and X3 is the reaction temperature (°C). The regression model was also applied for the calculation of the predicted values. Fig. 3A shows the normal probability of residuals. It was found to be well fitted with R2 close to 1 (R2 = 0.9590). Moreover, Fig. 3B also shows that the residual distribution of the model did not follow a particular trend with respect to the predicted values, indicating that this model is dependable and acceptable. Here, the lowest molar ratio of methanol to oil (20
:
1) was selected and fixed for the optimization and kinetic process.
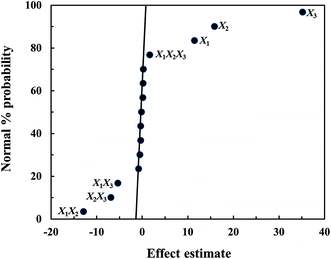 |
| Fig. 2 Normal probability plot of effect estimate for the 24 factorial design. | |
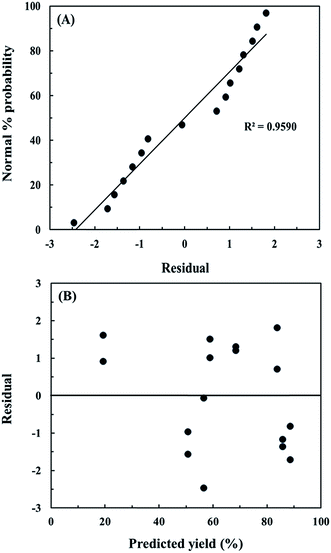 |
| Fig. 3 (A) Normal probability plot of residual and (B) distribution plot of residual versus predicted FAME yield for 24 factorial design. | |
The experimental design for the optimization of the ultrasonic-assisted biodiesel production from waste cooking oil over SO3H-CD was a Box–Behnken design. In this work, the Box–Behnken design is a combination between 2k factorial design with an incomplete block design, which the number of experiments were defined as N = 2k(k − 1) + C0 where N is the number of experiments, k is the number of parameters and C0 is the number of central points, providing to 15 experiments. The experimental results obtained based on this design are shown in Table 2. The quadratic regression model for the determination of the predicted values is given as follows in eqn (15):
|
Y = 87.7 + 7.675X1 + 3.938X2 + 6.513X3 − 9.25X12 − 6.225X22 − 6.525X32 − 2.575X1X2 − 5.175X1X3 − 3.1X2X3
| (15) |
where
Y is the FAME yield (%),
X1 is the catalyst loading (wt%),
X2 is the reaction time (min) and
X3 is the reaction temperature (°C).
Fig. 4 shows the plot between the experimental and predicted FAME yields. As expected, the predicted values are close to the experimental values, providing a value of R2 = 0.9770, indicating that the fitted model could explain 97.70% of the variability. The effects of catalyst loading and reaction time on the ultrasonic-assisted biodiesel production at a constant reaction temperature of 100 °C are presented in Fig. 5A. As expected, increasing the catalyst amount enhances the FAME yield because there is an increase in the number of active sites available for reaction. Moreover, it may be due to the fact that the addition of excess catalyst loading results in faster esterification and transesterification reactions which increases the FAME yield. However, the trend was reversed when the catalyst loading reached a certain amount, as a result of over catalyst loading making the esterification of free fatty acid progress faster and therefore more water could be easily formed in a shorter time. It is well known that an excess water amount can lead to the deactivation of the catalyst due to hydration occurring. The sulfonic acid-catalyzed esterification mechanism consists of protonation of the free fatty acid with the catalyst. The accessibility of alcohol on the carbocation leads to the occurrence of a tetrahedral intermediate and the release of water (Fig. 6). The mechanism was also related to the theoretical alcohol adsorption mechanisms of Langmuir–Hinshelwood and Eley–Rideal.36,37 In addition, it is possible that the increase of catalyst amount led to some problems such as phase separation and increased glycerol amount, which presented unsuitable conditions. The reaction time had a positive influence on the FAME yield but had less effect than catalyst loading, as can be observed in Fig. 5A. At too long reaction time, it could promote the backward reaction with a decreased FAME yield. This promotion was explained by the influence of ultrasonic energy increasing the interfacial area and activity of the microscopic and macroscopic bubbles formed when ultrasonic waves were applied in the three-phase reaction system.38 The bubbles will form and undergo breakdown after an approximate period at 400 ms, resulting in small hotspots that can offer energy for some chemical reactions (Fig. S2†). The effects of catalyst loading and reaction temperature on the ultrasonic-assisted biodiesel production at a constant reaction time of 8 min are presented in Fig. 5B. The FAME yield was increased significantly when the catalyst loading and reaction temperature were increased to the optimum conditions of 12 wt% and 120 °C, respectively, providing a maximum FAME yield (90%) as can be observed in Fig. 5B. Fig. 5C shows the effects of reaction time and reaction temperature at a constant catalyst loading of 10 wt%. As shown in Fig. 5C, a maximum FAME yield of 89.6% was obtained with a reaction time and a reaction temperature of 9.2 min and 120 °C, respectively. One can see in Fig. 5B and C that the reaction temperature was fixed at 120 °C. It should be noted that, at higher temperatures, the frying process and/or some chemical reactions such as thermolytic, hydrotic and oxidative reactions could occur. Moreover, it can lead to the evaporation of methanol as vapor phase in the reactor system, leading to less methanol available in the reaction environment.31 Based on the quadratic regression model, a predicted maximum FAME yield of 90.3% could be achieved under the optimum conditions: catalyst loading of 11.5 wt%, reaction time of 8.8 min and reaction temperature of 117 °C. To confirm the model’s prediction, the optimum conditions were tested up to three replicates for biodiesel production. The average FAME yield from the actual experiments was 90.8% indicating that the experimental value was close to the predicted value. For comparison, the optimum experimental conditions were compared to that using heating and stirring methods without the ultrasonic system. The FAME yield was reduced to about 56% without the ultrasonic system, suggesting that a longer reaction time was necessary. As a result, the use of the ultrasonic system is beneficial in terms of both the FAME yield as well as the rate of reaction.
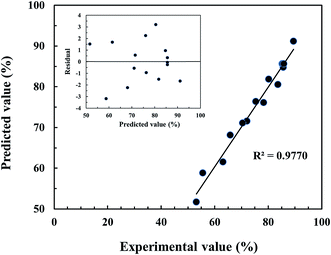 |
| Fig. 4 Plot of experimental versus predicted values of the FAME yield. The inset shows the distribution plot of residual versus predicted FAME yield for the Box–Behnken design. | |
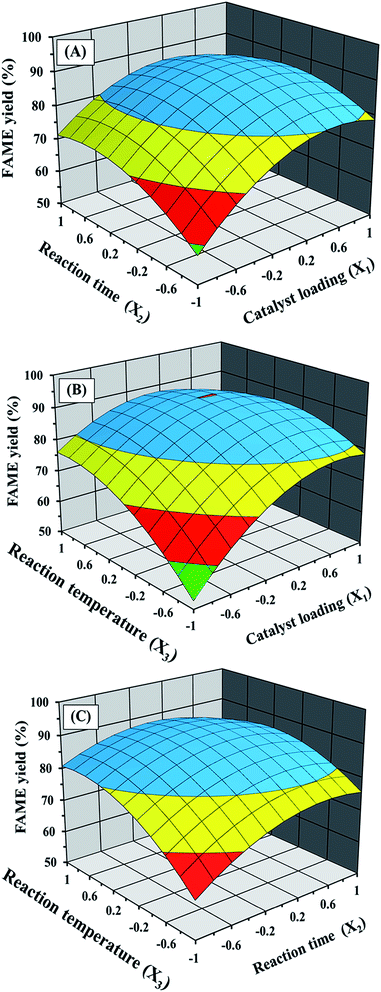 |
| Fig. 5 The response surface plot of the FAME yield: (A) based on catalyst loading and reaction time, (B) based on catalyst loading and reaction temperature, and (C) based on reaction time and reaction temperature. | |
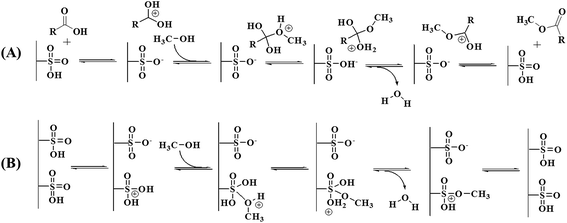 |
| Fig. 6 Water molecule obtained from the esterification of free fatty acid with SO3H-CD. | |
3.3 Catalyst reusability
The influence of catalyst regeneration on the ultrasonic-assisted biodiesel production is illustrated in Fig. 7. One can see that a significant reduction in catalytic activity was detected for the catalyst without regeneration. The reduction of the FAME yield in each cycle could be explained by different possibilities: (1) the deactivation of active sites due to their poisoning by some molecules present in the reaction mixture such as ion-exchange between alkali cations with protons and natural adsorption of reactants and products in the system and (2) the dependency of acid loss with temperature and sonic suggests that the acid sites are reacting, for instance with alcohol to form methyl sulfonates (Fig. S3†). However, in this case, a regeneration process via repeated sulfonation could be applied to solve the catalyst deactivation problems. As shown in Fig. 7, for the catalyst after regeneration, no serious reduction on FAME yield was observed in each cycle, suggesting that catalyst after regeneration could exist in the presence of sulfonic group, which was confirmed by titration and elemental analysis results in Table 3. Moreover, the presence of Na element on the surface of the spent catalyst (4th reuse) without regeneration can be clearly observed in the SEM-EDS result in Fig. S4.† However, with the spent catalyst (4th reuse) after regeneration, one can see that a very small amount of Na element together with an increasing amount of S were observed, resulting from the sulfonation with ion-exchange nature. For a comparison of the catalytic activity, commercial catalysts such as Amberlyst-15 (4.5 mmol g−1 of acidity and 45 m2 g−1 of surface area), zeolite (HY) (0.33 mmol g−1 of acidity and 425 m2 g−1 of surface area), γ-Al2O3 (0.31 mmol g−1 of acidity and 221 m2 g−1 of surface area) and CD-carbon derived from the hydrothermal carbonization of CD and water (0.02 mmol g−1 of acidity and 11 m2 g−1 of surface area) were tested for the transesterification of waste cooking oil. As shown in Fig. S5,† SO3H-CD exhibited better catalytic activity than the other catalysts. The CD-carbon obtained from single cyclodextrin showed almost no catalytic activity, resulting from its low acidity. This is probably due to their acidity promoting the activity for transesterification. To confirm the influence of acidity, SO3H-CD was synthesized with different acid densities, and the effect of acid density on the catalyst reusability result was studied. As shown in Fig. S6,† it can be seen that increasing FAME yield was related with increasing acid density. This result corresponded with the catalyst reusability result. Moreover, it is also believed that the outer layer of the SO3H-CD carbon sphere consists of an active site and hydrophilic groups such as sulfonic groups, carboxylic groups and hydroxyl groups while the inner layer consists of hydrophobic groups such as polyaromatics, which could promote the effect of mass transfer, leading to the higher catalytic performance.
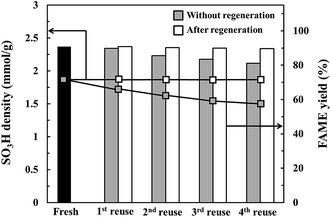 |
| Fig. 7 Catalyst reusability in biodiesel production from waste cooking oil. Reaction conditions: catalyst loading of 11.5 wt%, reaction time of 8.8 min and reaction temperature of 117 °C. | |
3.4 Kinetics of the transesterification process
In the present study, the kinetics of the transesterification reaction was investigated as a function of temperature and time in the range of 60 to 120 °C and 2 to 10 min, respectively. The optimum catalyst loading (11.5 wt%) was fixed. As shown in Fig. 8, it was indicated that the transesterification catalyzed by CD-SO3H and assisted by the ultrasonic system was a first order reaction as the kinetic data curves at each temperature were fitted with R2 > 0.99. According to the previous report by Freedman et al.,39 they found that the acid catalyzed transesterification process was a first order reaction. After fitting the data in Fig. 8, the reaction rate constants at different temperatures are given in Table 4. Based on the data in Table 4, given the considerable effect of reaction temperature on the rate constant, the relationship between them obeyed the Arrhenius law (eqn (13)). Fig. 9 shows the graph plotted between the natural logarithm of reaction rate constant (ln
k′) versus the inverse of temperature (1/T). The activation energy (Ea = 11.64 kJ mol−1) and pre-exponential factor (A = 3.14 min−1) were obtained from the slope and intercept of the Arrhenius plot, respectively. Activation energy values in the range of 33–84 kJ mol−1 have been reported for the acid–base catalyzed homogeneous transesterification of edible oils.39,40 Uzun et al.41 reported that the activation energy of the alkali-catalyzed transesterification of waste frying oils (WFO) was 11.7 kJ mol−1. The activation energy was reported as 70.6 kJ mol−1 for the alkaline ethanolysis of castor oil.42 For a heterogeneous catalyst, Kumar and Ali43 reported that the activation energy of transesterification using a nanocrystalline K–CaO catalyst was 54 kJ mol−1. The activation energies for the Zr/CaO catalyzed methanolysis and ethanolysis were found to be 29.8 kJ mol−1 and 42.5 kJ mol−1, respectively.44 Kansedo and Lee45 reported that an activation energy of 36.03 kJ mol−1 and pre-exponential factor of 5.56 × 102 min−1 were obtained using a sulfated zirconia catalyst for biodiesel production from non-edible sea mango oil. It should be noted that a range of activation energies (26–84 kJ mol−1) have been reported for heterogeneous catalysts for transesterification reactions. This evidence indicates that the activation energy obtained in this work is outside the range of reported values. This is due to the effect of the reduction of the mass transfer resistance which was helped by the cavitation process of intense turbulence and micro-scale liquid circulation. Based on the fitting eqn (16), it was clearly confirmed that ln
k changed linearly with 1/T in the studied temperature range as expected for a single rate-limited thermally activated process. |
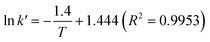 | (16) |
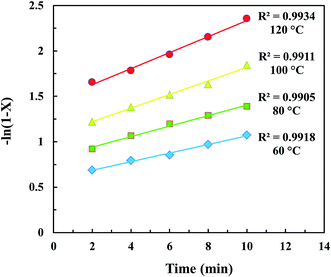 |
| Fig. 8 The first order reaction plot between time versus −ln(1 − X). | |
Table 4 Reaction rate constants for the ultrasonic-assisted transesterification of waste cooking oil and methanol using the CD-SO3H catalyst at different temperatures
Temperature (°C) |
Reaction rate constant (min−1) |
R2 |
60 |
0.0472 |
0.9918 |
80 |
0.0581 |
0.9905 |
100 |
0.0751 |
0.9911 |
120 |
0.0884 |
0.9934 |
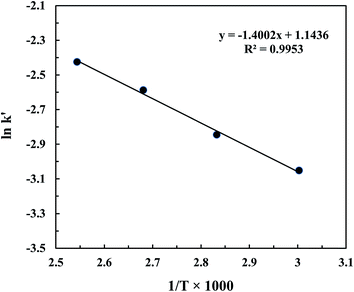 |
| Fig. 9 Arrhenius plot 1/T versus ln k′. | |
Moreover, the reaction temperature was proved to have a positive effect which was closely related to the conversion efficiency of the transesterification process. The FAME or biodiesel product was tested by comparing with the American ASTM D 6751 standard (Table 5). The properties of the biodiesel produced in this work met the criteria of the ASTM standard. From this result, it could be rated as a realistic alternative to petroleum diesel.
Table 5 The properties of biodiesel in this work with ASTM D 6751 standard
Properties |
Unit |
Value |
Biodiesel (ASTM 6751) |
Biodiesel (this work) |
Density@15 °C |
g cm−3 |
0.86–0.89 |
0.8766 |
Viscosity@15 °C |
mm2 s−1 |
1.9–6.0 |
4.15 |
Oxidative stability@110 °C |
h |
≥3 |
2.6 |
Pour point |
°C |
−10 to 12 |
2 |
Flash point |
°C |
≥130 |
194 |
Heating value |
cal g−1 |
9940 |
9912 |
Cetane number |
|
≥47 |
50 |
Acid value |
(KOH mg kg−1) |
≤0.5 |
0.45 |
Sulfur content |
(%, w/w) |
≤0.05 |
0.003 |
Water content |
(mg kg−1) |
≤0.05 |
0.0045 |
4 Conclusions
In the present study, the ultrasonic-assisted transesterification process catalyzed by SO3H-CD was proved to be a high performance technology for biodiesel production. The catalyst was characterized by BET, XRD, PSD, SEM-EDS, TGA, FT-IR, XPS and TPD. The statistical analyses were clarified by R2 > 0.95. The maximum FAME yield was 90.8% at the optimum conditions: catalyst loading of 11.5 wt%, reaction time of 8.8 min and reaction temperature of 117 °C. The stability and performance of the catalyst were excellent after regeneration. The SO3H-CD showed better catalytic performance compared to commercial catalysts. Kinetic studies showed that the reaction followed first order reaction kinetics with an activation energy of 11.64 kJ mol−1 and a pre-exponential factor of 3.14 min−1.
Acknowledgements
The authors wish to acknowledge the Department of Chemistry, Faculty of Science, Rangsit University for supporting all instruments and chemicals.
References
- D. Simionato, S. Basso, G. M. Giacometti and T. Morosinotto, Biophys. Chem., 2013, 182, 71–78 CrossRef CAS PubMed.
- I. M. R. Fattah, M. A. Kalam, H. H. Masjuki and M. A. Wakil, RSC Adv., 2014, 4, 17787–17796 RSC.
- S. B. Lee, K. H. Han, J. D. Lee and I. K. Hong, J. Ind. Eng. Chem., 2010, 16, 1006–1010 CrossRef CAS PubMed.
- K. S. Parthiban and M. Perumalsamy, RSC Adv., 2015, 5, 11180–11187 RSC.
- V. G. Gude and G. E. Grant, Appl. Energy, 2013, 109, 135–144 CrossRef CAS PubMed.
- N. Kaur and A. Ali, Appl. Catal., A, 2015, 489, 193–202 CrossRef CAS PubMed.
- N. Kaur and A. Ali, RSC Adv., 2015, 5, 13285–13295 RSC.
- M. Kaur and A. Ali, Eur. J. Lipid Sci. Technol., 2015, 117, 550–560 CrossRef CAS PubMed.
- W. Y. Lou, M. H. Zong and Z. Q. Duan, Bioresour. Technol., 2008, 99, 8752–8758 CrossRef CAS PubMed.
- J. Chen, L. Jia, X. Guo, L. Xiang and S. Lou, RSC Adv., 2014, 4, 60025–60033 RSC.
- I. M. Atadashi, M. K. Aroua, A. R. A. Aziz and N. M. N. Sulaiman, J. Ind. Eng. Chem., 2013, 19, 14–26 CrossRef CAS PubMed.
- D. W. Lee and B. R. Yoo, J. Ind. Eng. Chem., 2014, 20, 3947–3959 CrossRef CAS PubMed.
- Y. Zhang, W. T. Wong and K. F. Yung, Appl. Energy, 2014, 116, 191–198 CrossRef CAS PubMed.
- P. Yin, L. Chen, Z. Wang, R. Qu, X. Liu, Q. Xu and S. Ren, Fuel, 2012, 102, 499–505 CrossRef CAS PubMed.
- M. Li, Y. Zheng, Y. Chen and X. Zhu, Bioresour. Technol., 2014, 154, 345–348 CrossRef CAS PubMed.
- D. Zuo, J. Lane, D. Culy, M. Schultz, A. Pullar and M. Waxman, Appl. Catal., B, 2013, 129, 342–350 CrossRef CAS PubMed.
- A. S. Badday, A. Z. Abdullah and K. T. Lee, Appl. Energy, 2013, 105, 380–388 CrossRef CAS PubMed.
- M. Hara, Top. Catal., 2010, 53, 805–810 CrossRef CAS.
- X. B. Fu, J. Chen, X. L. Song, Y. M. Zhang, Y. Zhu, J. Yang and C. W. Zhang, J. Am. Oil Chem. Soc., 2015, 92, 495–502 CrossRef CAS PubMed.
- G. Chen, R. Shan, J. Shi and B. Yan, Bioresour. Technol., 2014, 171, 428–432 CrossRef CAS PubMed.
- M. Maghami, S. M. Sadrameli and B. Ghobadian, Appl. Therm. Eng., 2015, 75, 575–579 CrossRef CAS PubMed.
- J. Ji, J. Wang, Y. Li, Y. Yu and Z. Xu, Ultrason. Sonochem., 2006, 44, e411–e414 CrossRef PubMed.
- C. Stavarache, M. Vinatoru, R. Nishimura and Y. Maeda, Ultrason. Sonochem., 2005, 12, 367–372 CrossRef CAS PubMed.
- I. Choedkiatsakul, K. Ngaosuwan, G. Cravotto and S. Assabumrungrat, Ultrason. Sonochem., 2014, 21, 1585–1591 CrossRef CAS PubMed.
- M. Takase, Y. Chen, H. Liu, T. Zhao, L. Yang and X. Wu, Ultrason. Sonochem., 2014, 21, 1752–1762 CrossRef CAS PubMed.
- H. Shahraki, M. H. Entezari and E. K. Goharshadi, Ultrason. Sonochem., 2015, 23, 266–274 CrossRef CAS PubMed.
- D. D. Pukale, G. L. Maddikeri, P. R. Gogate, A. B. Pandit and A. P. Pratap, Ultrason. Sonochem., 2015, 22, 278–286 CrossRef CAS PubMed.
- D. Kumar, G. Kumar, Poonam and C. P. Singh, Ultrason. Sonochem., 2010, 17, 839–844 CrossRef CAS PubMed.
- S. M. Hingu, P. R. Gogate and V. K. Rathod, Ultrason. Sonochem., 2010, 17, 827–832 CrossRef CAS PubMed.
- P. Kumar, M. Aslam, N. Singh, S. Mittal, A. Bansal, M. K. Jha and A. K. Sarma, RSC Adv., 2015, 5, 9946–9954 RSC.
- A. Talebian-Kiakalaieh, N. A. S. Amin, A. Zarei and I. Noshadi, Appl. Energy, 2013, 102, 283–292 CrossRef CAS PubMed.
- Q. Shu, Q. Zhang, G. Xu, Z. Nawaz, D. Wang and J. Wang, Fuel Process. Technol., 2009, 90, 1002–1008 CrossRef CAS PubMed.
- Q. Shu, Z. Nawaz, J. Gao, Y. Liao, Q. Zhang, D. Wang and J. Wang, Bioresour. Technol., 2010, 101, 5374–5384 CrossRef CAS PubMed.
- Q. Shu, J. Gao, Z. Nawaz, Y. Liao, D. Wang and J. Wang, Appl. Energy, 2010, 87, 2589–2596 CrossRef CAS PubMed.
- S. H. Shuit, K. F. Yee, K. T. Lee, B. Subhash and S. H. Tan, RSC Adv., 2013, 3, 9070–9094 RSC.
- S. Miao and B. H. Shanks, J. Catal., 2011, 279, 136–143 CrossRef CAS PubMed.
- Y. Liu, E. Lotero and J. G. Goodwin Jr, J. Catal., 2006, 242, 278–286 CrossRef CAS PubMed.
- H. Mootabadi, B. Salamatinia, S. Bhatia and A. Z. Abdullah, Fuel, 2010, 89, 1818–1825 CrossRef CAS PubMed.
- B. Freedman, R. O. Butterfield and E. H. Pryde, J. Am. Oil Chem. Soc., 1986, 63, 1375–1380 CrossRef CAS.
- H. Noureddini and D. Zhu, J. Am. Oil Chem. Soc., 1997, 74, 1457–1463 CrossRef CAS.
- B. B. Uzun, M. Kılıç, N. Özbay, A. E. Pütün and E. Pütün, Energy, 2012, 44, 347–351 CrossRef CAS PubMed.
- N. D. L. da Silva, C. B. Batistella, R. M. Filho and M. R. W. Maciel, Energy Fuels, 2009, 23, 5636–5642 CrossRef.
- D. Kumar and A. Ali, Biomass Bioenergy, 2012, 46, 459–468 CrossRef CAS PubMed.
- N. Kaur and A. Ali, Fuel Process. Technol., 2014, 119, 173–184 CrossRef CAS PubMed.
- J. Kansedo and K. T. Lee, Chem. Eng. J., 2013, 214, 157–164 CrossRef CAS PubMed.
Footnote |
† Electronic supplementary information (ESI) available. See DOI: 10.1039/c5ra09499b |
|
This journal is © The Royal Society of Chemistry 2015 |