DOI:
10.1039/C5RA08222F
(Paper)
RSC Adv., 2015,
5, 48720-48728
A thixotropic polyglycerol sebacate-based supramolecular hydrogel showing UCST behavior
Received
4th May 2015
, Accepted 27th May 2015
First published on 27th May 2015
Abstract
Polyglycerol sebacate (PGS) is a relatively new biodegradable and elastomeric material that exhibits superior biocompatibility, a modulus that is comparable to human soft tissue, and linear biodegradation. However, the high hydrophobicity, low water uptake percentage and extreme synthesis conditions of PGS pose as a hindrance to its use in cellular biology, biomedical and other applications. We were able to modify PGS using atom transfer radical polymerization (ATRP) by synthesizing a PGS macroinitiator, allowing us to improve PGS's properties or introduce new properties to PGS. This macroinitiator would enable the building of a robust platform of PGS-based copolymers with monomers in the methacrylates, styrenes and methacrylamide families. We demonstrated the feasibility of this macroinitiator by using PEGMEMA as the monomer to synthesize PGS–PEGMEMA and created a PGS–PEGMEMA/αCD supramolecular hydrogel system. This hydrogel exhibited a tunable, low UCST of less than 90 °C, low minimum gelation concentration of 5.2%, rapid gelation and rapid self-healing ability with relatively high modulus (∼100 kPa) that is comparable to that of human soft tissue. This hydrogel system is injectable yet strong enough to provide support – features, making it a very suitable candidate as a vehicle for injectable sustained-release drug delivery, cell delivery, tissue engineering scaffold as well as for cosmetics and skincare applications.
Introduction
Biodegradable polymers have been widely explored for a wide variety of potential biomedical applications.1–5 Polyglycerol sebacate (PGS) is a relatively new biodegradable and elastomeric material that was first reported in 2002. It is synthesized in a two-step process – synthesis of the prepolymer, and the thermal curing of the prepolymer into a tough elastomer. Both steps happen at a high temperature of 120 °C and may take up to 5 days to synthesize completely.6,7 Fully cured PGS has a modulus of 0.28 MPa (ref. 8) which is comparable to some human soft tissue such as ligaments.7 PGS is a hydrophobic polymer with alternating units of glycerol and sebacic acid and has been shown to biodegrade in vitro, with increased biodegradation rates in the presence of enzymes such as lipases in vivo.6,9 The degradation profile of PGS described by previous literature is linear as compared to conventional biodegradable materials such as poly-L-lactic acid or polyglycolic acid, which swell and degrade drastically.6,9,10 PGS also exhibits excellent biocompatibility11,12 and hence can potentially be a suitable material for numerous biological and biomedical applications. Due to all the above properties of PGS, it has been widely explored for applications such as drug delivery, biocompatible coatings on implantable biomedical devices and tissue engineering (such as cardiac, vascular and nervous tissues).8,13–18
However, the high hydrophobicity, low water uptake percentage and extreme synthesis conditions of PGS pose as a hindrance to its use in cellular biology, biomedical and other applications. Various chemical modifications have been developed to improve the synthesis technique, as well as to enhance the material properties of PGS for various applications. Acrylation, for instance, was used to replace thermal curing with ultraviolet (UV) curing. This reduces the curing time of PGS from one to two days to a few hours.19 Urethane cross-linking was adopted to improve the ease of synthesis20 and co-esterification with polyethylene glycol (PEG) was performed to improve the swelling ratio and water uptake percentage of PGS.21
Atom transfer radical polymerization (ATRP) is a robust, highly efficient polymerization reaction that was developed by Krzysztof Matyjaszewski in 1995.22 This polymerization method has been commonly used on various substrates for the polymerization of methacrylate, styrenes and (meth)acrylamides monomers by our group and others.23–27 The initiator used for ATRP can vary from a simple ethyl α-bromoisobutyrate26 and 4-toluenesulfonyl chloride,28 to a macromolecule such as poly(arylene oxindole)29 and poly(p-chloromethyl styrene),30 to graphene31 and even a silicon wafer surface.32 However, to date, there had been no ATRP performed with PGS as an initiator.
In this paper, we outline the synthesis of a PGS macroinitiator for ATRP, which enables the building of a robust platform of PGS-based copolymers. Such a PGS macroinitiator allows for the copolymerization of PGS with a wide range of monomers, including methacrylates, styrenes and methacrylamide families. We demonstrated the feasibility of this macroinitiator by using PEGMEMA as the monomer to synthesize PGS–PEGMEMA. This PGS–PEGMEMA confers a brush-like structure on the PGS core molecule, with the chains of PEGMEMA extending out (Fig. 1).
 |
| Fig. 1 Schematic of PGS–PEGMEMA/αCD hydrogel system. The multi-armed polyglycerol sebacate (PGS) cores were copolymerized with the brush-like PEGMEMA via atom transfer radical polymerization (ATRP). α-Cyclodextrin (αCD) were threaded onto each PEG segment of PEGMEMA to form columns of inclusion complexes (shown in the inset). These brush-like columns of PEGMEMA/αCD inclusion complexes interact with each other via hydrogen bonding to form the hydrogel matrix that was able to hold water. | |
It has been long known that PEG forms inclusion complexes with α-cyclodextrin.33,34 Supramolecular constructs have been reported to form hydrogels in several reviews.34–40 Hydrogel composites are also useful for biomedical applications.41 With this novel PGS–PEGMEMA, we created a PGS–PEGMEMA/αCD supramolecular hydrogel system that exhibited a tunable, low UCST of less than 90 °C, low minimum gelation concentration of about 5%, rapid gelation and rapid self-healing ability with a relatively high modulus that is comparable to that of human soft tissue. This hydrogel system is injectable yet strong enough to provide support – features that make it a very suitable candidate for use in cell delivery, tissue engineering scaffolds, cosmetics and skincare applications, and as a vehicle for injectable sustained-release drug delivery.
Methods
Materials and chemicals
Glycerol, sebacic acid, triethylamine (TEA), anhydrous tetrahydrofuran (THF), bromoisobutyryl bromide (BIBB), polyethylene glycol methyl ether methacrylate (PEGMEMA) (Mn = ∼1100 g mol−1), 1,1,4,7,10,10-hexamethyltriethylenetetramine (HMTETA), copper(I) bromide, doxorubicin, ethyl α-bromoisobutyrate (EBIB) and α-cyclodextrin (αCD) were purchased from Sigma-Aldrich.
Synthesis
Polyglycerol sebacate (PGS) prepolymer was synthesized using the method as previously described.7 An equimolar of sebacic acid and glycerol was weighed and mixed at 120 °C under N2 for 24 hours. The mixture was reacted at 120 °C under 30 mTorr vacuum for 48 hours to yield the PGS prepolymer.
The PGS prepolymer was dried at 50 °C under vacuum overnight and purged with N2 for 1 hour, before it was in anhydrous THF. TEA of 1.2 molar times to BIBB was added. BIBB at 5 molar ratio of the number of moles of hydroxyl groups was added and reacted overnight at room temperature for complete bromination (Fig. 2). The degree of bromination (number of hydroxyl groups converted to the bromoisobutyrate groups/total number of hydroxyl groups present) could be controlled by varying the amount of BIBB. A sample of PGS with partial bromination was prepared with 0.5 molar equivalent of the number of hydroxyl groups present in PGS prepolymer.
 |
| Fig. 2 The synthesis of PGS-Br macroinitiator by bromination and the ATRP of PGS-Br with PEGMEMA as the monomer. | |
The reaction mixture was centrifuged and the supernatant was precipitated in ether. PEGMEMA was added to PGS-Br (Table 1) with HMTETA as the initiator, and dissolved in 10 mL of isopropanol (Fig. 2). The mixture was bubbled with nitrogen for 45 min before copper(I) bromide was added as the catalyst. The mixture was reacted overnight at room temperature. The reaction mixture was diluted in THF before it was purified in an aluminum oxide column (about 5 cm) to remove the catalyst and the filtrate was concentrated under vacuum. The concentrated polymer was precipitated in hexane.
Table 1 Ratio of PGS-Br initiator and PEGMEMA monomer added for synthesis of PGS–PEGMEMA
|
PGS-Br (g) |
PEGMEMA monomer (g) |
PGS–PEGMEMA1 |
0.013 |
8 |
PGS–PEGMEMA2 |
0.16 |
8 |
PGS–PEGMEMA3 |
0.2 |
0.5 |
PGS–PEGMEMA characterization
All polymers and hydrogels were characterized by measuring the melting temperature (Tm), crystallization temperature (Tc) and glass transition (Tg), if any, on a differential scanning calorimetry (DSC) (TA Q100), the thermal degradation temperature (Td) on a thermogravimetric analysis (TGA) (TA Q500), and the molecular weight (Mw) on a gel permeation chromatography (GPC) (Waters 2690).
Preparation of hydrogel
The hydrogels were prepared by mixing the stock solution of 20% αCD and the stock solution of 10% PGS–PEGMEMA (w/v%) and letting the mixture set overnight to form the supramolecular hydrogel. All hydrogels were prepared with 2% polymer and a varying amount of αCD at 5%, 9%, and 13% (w/v%) in PBS, unless stated otherwise.
Gel characterization
Gelation concentration. PGS–PEGMEMA and α-CD of a range of weight percentage (wt%) were mixed and their physical form was observed after the mixture was set overnight. A phase diagram was generated.
Gelation transition temperature. With a constant PGS–PEGMA concentration of 2% (w/v), hydrogels were prepared with varying α-CD concentration of 5, 7, 9, 11, and 13% (w/v). The gel to solution transition temperature was measured by two methods: physical observation and by tracking the transmission on a UV spectrometer. A control gel of 2% PEG20 kDa + 9% αCD was prepared to compare as a frame of reference.In the physical observation method, 250 µL of hydrogels prepared were left in a water bath where its temperature was stepped from 25 to 80 °C at 5 °C intervals. The hydrogel was left at each temperature for 5 minutes before the vial was observed visually for phase transition. The temperature at which the solution turned clear was determined to be the transition temperature.
For the UV spectrometer method, 3 mL of hydrogel was prepared in a cuvette and analyzed on a temperature-regulated UV spectrometer (Shimadzu Corporation UV-2501PC). The spectrometer was first calibrated with PBS. The transmission at 600 nm was recorded over time as the temperature of the sample was increased from 25 °C to 90 °C. The percentage transmission was plotted over temperature.
Gelation speed. The gelation speed between PGS–PEGMEMA and α-CD was determined using the inversion method. 1 mL of the gels of 2% PGS–PEGMEMA and 5, 9, 13% α-CD were prepared. Control gels of 2% PEG20 kDa + 9% αCD and 13% αCD were prepared as frames of reference. These gels were first left at 80 °C for 15 min to allow them to turn into solutions before they were placed in a 25 °C water bath. The gels were inverted at different time intervals to determine the gel formation. The minimum time required for the solution to stop flowing upon inversion was determined to be the gelation time.
X-ray diffraction. Hydrogels of 2% PGS–PEGMEMA2 and 5, 9, 13% αCD were prepared and frozen dry overnight. PGS–PEGMEMA2, αCD in their dry state and the freeze-dried hydrogels were measured on the X-ray diffraction machine (Bruker Discover D8 General Area Detector Diffraction System).
Rheology. A rheometer (TA Discovery HR-3) was used to apply the gels to cycles of high (10%, 120 seconds) and low (0.01%, 600 seconds) shear strain percentages. The high shear strain sheared the gel into a liquid while the period of low shear strain allowed the gel to recover or ‘heal’ itself to return to gel. Ten cycles were applied to each gel at a frequency of 0.1 Hz.
Results
Synthesis
The series of reactions was successful, as demonstrated by the NMR spectrum (Fig. 3). PGS had the characteristic peaks attributed by the protons attached to the carbons in the sebacic acid component (peaks labeled d, e and f). There were some signals displayed by the glycerol protons as well in the range of 3.7–4.3 ppm (not labeled). After reacting PGS with BIBB, there was an addition of the distinctive peak at about 1.9 ppm (peak g) that corresponded to the bromoisobutyrate group added to PGS.
 |
| Fig. 3 The NMR spectrum of PGS, PGS-Br (partial bromination), PGS-Br, PGS–PEGMEMA3 (where there was a high PGS-Br : PEGMEMA monomer ratio), and PGS–PEGMEMA1 and 2 were shown. This NMR spectrum outlined the flow of reactions from PGS all the way to PGS–PEGMEMA. The degree of conversion of hydroxyl groups to bromoisobutyrate groups could be adjusted by controlling the number of moles of BIBB added to the reaction with respect to the number of moles of hydroxyl groups present. PGS–PEGMEMA demonstrated the ability of ATRP to co-polymerize PGS (peaks d, e and f) and PEGMEMA (peaks a, b and c). For higher monomer ratios of PGS–PEGMEMA1 and 2, only peaks corresponding to PEGMEMA could be observed as the PGS peaks were too low to be seen. | |
The percentage conversion from hydroxyl groups to bromoisobutyrate groups could be calculated through the equation below.
The integral of peak g varied with the ratio of BIBB
:
PGS prepolymer added. Full conversion (about 94%) was achieved consistently when an excess of 5 molar equivalents was added to PGS prepolymer. With 0.5 molar equivalent of BIBB added, the calculated conversion percentage was 11%. This demonstrated that the degree of conversion could be adjusted by controlling the amount of BIBB added to the bromination reaction.
PGS-Br was then reacted with monomer PEGMEMA via atom transfer radical polymerization (ATRP). As complete formation of PGS–PEGMEMA1 and 2 would lead to strong PEG peaks that would overwhelm the PGS peaks in the NMR spectrum, PGS–PEGMEMA3 was prepared with a high PEGMEMA/PGS ratio to demonstrate the incorporation of PGS in the final PGS–PEGMEMA polymer (both groups of peaks (d), (e) and (f) representing PGS and peaks (a), (b) and (c) representing PEGMEMA could be seen). Complete reactions of PGS–PEGMEMA1 and 2 exhibited primarily the PEGMEMA peaks.
Polymer characterization
The results of the characterizations for the PGS–PEGMEMA samples were shown in Table 2. The molecular weights of the polymers were measured and their polydispersity index shown. The melting temperature (Tm), crystallization temperature (Tc) and thermal decomposition temperatures (Td) were listed. The number of repeating units for each co-polymer was also included, with n representing the number of repeating units of glycerol and sebacic acid pair in PGS and m representing the number of repeating units of PEGMEMA monomer.
Table 2 The melting temperature (Tm), crystallization temperature (Tc) and decomposition temperature (Td) of PGS, PGS–PEGMEMA1 and 2 were shown. The number average molecular weight (Mn), weight average molecular weight (Mw), polydispersity index (PDI) and the number of repeating units for each co-polymer were also listed (n representing the number of repeating units of PGS and m the number of repeating units of PEGMEMA)
Sample |
Mn (g mol−1) |
Mw (g mol−1) |
PDI |
Number of repeating units (n and m) |
Tm |
Tc |
Td |
PGS prepolymer |
4080 |
17 700 |
4.34 |
n = 15 |
8.3 °C |
−11.8 °C |
416 °C |
PGS–PEGMEMA1 |
37 000 |
118 000 |
3.17 |
n = 15 |
39.3 °C |
−9.0 °C |
412 °C |
m = 30 |
PGS–PEGMEMA2 |
35 000 |
123 000 |
3.46 |
n = 15 |
38.1 °C |
−6.7 °C |
398 °C |
m = 28 |
Hydrogel characterization
The PGS–PEGMEMA/αCD supramolecular hydrogel exhibited some interesting properties (Fig. 4). This hydrogel had a shear thinning capability as it changed from a turbid gel to a turbid solution upon mechanical disturbance such as stirring or shaking. It was able to ‘self-heal’ after a mechanical disturbance as it settled back into a turbid gel that no longer flows after a period of time. This supramolecular hydrogel also demonstrated upper critical solution temperature (UCST) behavior as it transited from a solid white turbid gel state to a turbid solution and finally to a clear solution as it was heated above its transition temperature. Various experiments were performed to characterize this hydrogel.
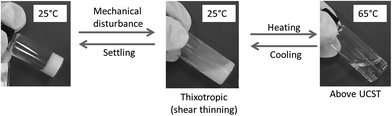 |
| Fig. 4 Picture showing how the 2% PGS–PEGMEMA1 + 5% αCD gel demonstrated its ability to shear thin and exhibited the characteristics of an UCST system. | |
Gelation concentration
The gelation concentration study showed that the minimum gelation concentration was 0.2% polymer + 5% αCD for PGS–PEGMEMA1 and 0.4% polymer + 5% αCD for PGS–PEGMEMA2 (Fig. 5A and B). These minimum gelation concentrations were very low compared to 1.5% polymer + 7% αCD in the PEG control gel (Fig. 5C).
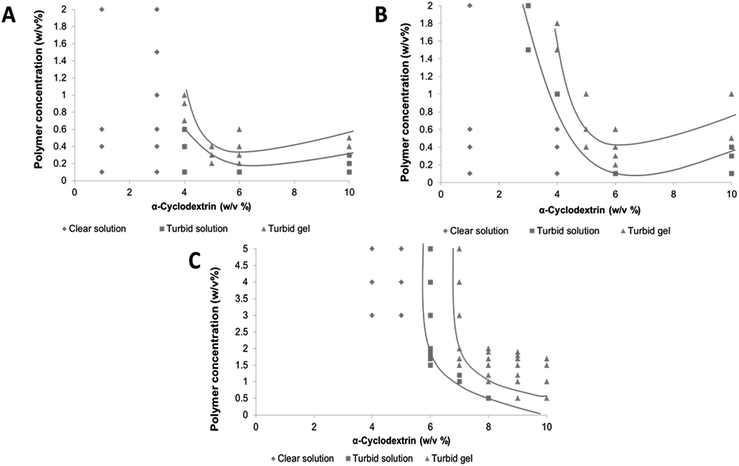 |
| Fig. 5 The phase diagram of PGS–PEGMEMA1 (A) and PGS–PEGMEMA2 (B). The minimum gelation concentration is 0.2% polymer + 5% αCD for PGS–PEGMEMA1 and 0.4% polymer + 5% αCD for PGS–PEGMEMA2. The minimum gelation concentration of the PGS–PEGMEMA is very low compared to the PEG/αCD hydrogel system (C) which had a minimum gelation concentration of 8.5%. | |
α-Cyclodextrin have the ability to form inclusion complexes with polyethylene glycol (PEG) chains, which then aggregate and stack themselves into columns. These columns interact with each other via hydrogen bonding to form a matrix that will retain water, forming a physical gel.42,43 Under the same mechanism, the PEG segments of PGS–PEGMEMA form a supramolecular hydrogel with α-cyclodextrin (α-CD) as well.
The very low minimum gelation concentration was likely attributed by the brush-like structure of the PGS–PEGMEMA. In this PGS–PEGMEMA/αCD hydrogel system, αCD molecules threaded themselves onto each of the PEG segment of PGS–PEGMEMA, forming numerous columns. The brush-like structure confined the PEGMEMA chains to be in close proximity to each other in a regular structure and hence enhanced the interactions and hydrogen bonding between these columns of threaded αCD, compared to columns of free floating threaded PEG chains in the PEG control gel. Moreover, the multi-armed PGS segment held all the PEGMEMA chains together on a branched core, further facilitating the hydrogen bonding between the columns of αCD. This ease of stacking and supramolecular cross-linking resulted in the low minimum gelation concentrations of only 5.2–5.4% for gel formation.
Gelation speed
The time it took for the solution of PGS–PEGMEMA and αCD to form a gel was investigated for PGS–PEGMEMA of 2% and αCD concentrations from 5% to 13%. The gelation speed increased when the concentration of αCD was increased because more αCD became available for threading onto each PEGMEMA chain to form columns and there was a stronger interaction between columns to form the gel. At an αCD concentration of 13%, it only required 3 minutes for the formation of an opaque hydrogel while the 2% PEG + 13% αCD control gel required 10 min to gel while the 2% PEG + 9% αCD control gel took 23 min to gel (Table 3).
Table 3 The table listing the time it takes for the gel to form. The gelation speed increases with increasing concentration of αCD. The control gel of 2% PEG + 13% αCD required 10 min to gel and the 2% PEG + 9% αCD control gel took 23 min to gel
Concentration of α-CD (%w/v) |
PGS–PEGMEMA1 |
PGS–PEGMEMA2 |
5 |
>1 hour |
> 1 hour |
7 |
15 min |
11 min |
9 |
9 min |
9 min |
11 |
8 min |
8 min |
13 |
5 min |
3 min |
Gelation transition temperature
The PGS–PEGMEMA/αCD gels exhibited the interesting property of having an upper critical solution temperature (UCST). The gels transited from a solid white turbid gel state to a turbid solution and to a clear solution as they were heated above their respective transition temperatures. This transition temperature could be adjusted by altering the αCD concentrations. Fig. 6 showed the measurements of the UCST temperatures of the PGS–PEGMEMA1 and 2 supramolecular gels at 2% PGS–PEGMEMA and a range of αCD concentrations from 5% to 13%. The measurements were performed with two different methods: by observing the physical state change and by using a spectrometer to measure the change in transmittance as the gels turns clear. The transition temperature was recorded as the temperature at which the gel had attained 50% transmittance. The tables below each of the plots showed the UCST measured with each method. Both methods converged to a similar temperature trend as shown in the table below each of the spectrometer plots (Fig. 6A and B). The UCST increased linearly with the concentration of αCD (Fig. 6C). This temperature response was due to the unstacking and the subsequent unthreading of the αCD from the PEGMEMA chains. As energy was applied to the PGS–PEGMEMA/αCD system, the columns of PEGMEMA/αCD gained mobility and started to free themselves from the stacked conformation, turning the gel from a solid state to a liquid state. As even more energy was applied, the αCD were unthreaded from each of the PEGMEMA chains and the inclusion complexes came apart, turning the turbid solution clear. This PGS–PEGMEMA/αCD gel system was therefore able to unbind its water-holding matrix and release its contents at its transition temperature, which could be easily tuned with the concentration of αCD. As a frame of reference, the gel to sol transition temperature for the 2% PEG + 9% αCD was 95 °C and the solution did not turn clear below 100 °C.
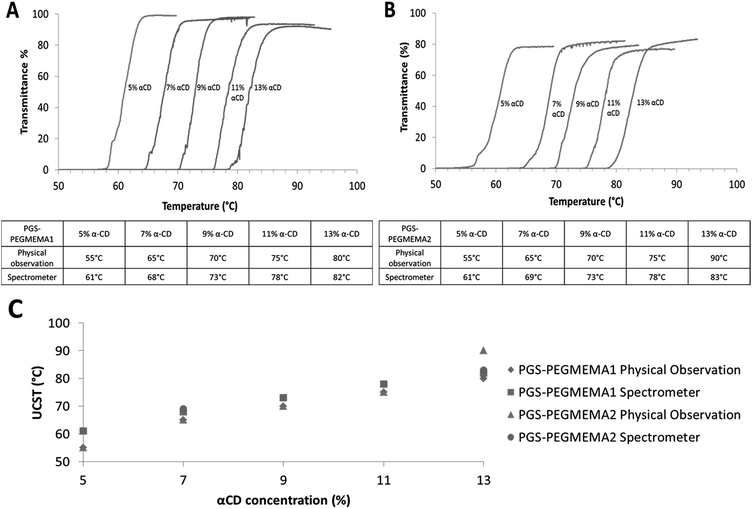 |
| Fig. 6 The upper critical solution temperature (UCST) behaviour of the PGS–PEGMEMA/αCD gel systems at 2% PGS–PEGMEMA. The UCST of PGS–PEGMEMA1 (A) and PGS–PEGMEMA2 (B) measured using the physical observation method and the spectrometer methods were shown in the tables below the spectrometer plots. The UCSTs varies linearly with the αCD concentration (C). The control PEG/αCD gel did not result in a transmittance change on the spectrometer, but physical observation of the 2% PEG + 9% αCD control gel exhibited a turbid gel to turbid sol transition at 95 °C. The turbid solution did not turn clear below 100 °C. | |
X-ray diffraction
The X-ray diffraction pattern of the PGS–PEGMEMA2/αCD gels, PGS–PEGMEMA2 only and αCD only samples were shown in Fig. 7. The presence of multiple broad peaks implicated the random alignment structure of the αCD powder. In contrast, after interacting with PGS–PEGMEMA2, the appearance of the sharp and distinct peak at 2θ of 19.9° reflected the formation of the stacked column structure of the PGS–PEGMEMA2/αCD inclusion complexes, similar to the inclusion complexes formed between αCD and PEG as reported in previous literature.43,44 The PGS–PEGMEMA1/αCD system showed a similar diffraction pattern and was not included.
 |
| Fig. 7 X-ray diffraction pattern of the freeze-dried hydrogels at 2% PGS–PEGMEMA2 + 5% αCD, 2% PGS–PEGMEMA2 + 9% αCD, 2% PGS–PEGMEMA2 + 13% αCD, PGS–PEGMEMA2 only and αCD only. The presence of the strong peak at 19.9° reflects the formation of the regular column structure of the inclusion complexes of αCD molecules threaded on each of the PEG chains in PGS–PEGMEMA. | |
Rheology
The PGS–PEGMEMA/αCD supramolecular hydrogel has the ability to self-heal after it had been sheared with a high strain of 10%. Fig. 8 showed the change in the storage and loss modulus of the 2% PGS–PEGMEMA1 hydrogels at 5%, 9% and 13% αCD. The moduli increased with increasing αCD as more hydrogen bonding between columns of PEG/αCD was expected. At a high strain of 10%, the moduli dropped by one order of magnitude for the 5% αCD gel and approximately two orders of magnitude for the 9% and 13% αCD hydrogels as they shear thinned. The storage and loss moduli were also reversed, indicating the phase transition from a gel to a solution. Upon the application of a low strain of 0.01%, the moduli reversed back to having a higher storage modulus than the loss modulus with the same magnitude as before the shear. This demonstrated its ability to heal back from a solution into a gel. The healing time was relatively short and increased with decreasing αCD concentration – instantaneous at 13% αCD down to about 1 minute at 5% αCD. At a high αCD concentration, the hydrogel was able to achieve high moduli of ∼100 kPa. By reducing the αCD concentration from 13% to 5%, the modulus of the gel could be decreased by about 3 folds of magnitude. Therefore, hydrogels of a desirable modulus could be tuned easily by controlling the αCD concentration.
 |
| Fig. 8 The storage and loss moduli as measured by a rheometer on 2% PGS–PEGMEMA1 with αCD of 5%, 9% and 13%. The moduli increased with increasing αCD concentration. With every cycle of high strain of 10%, the storage and loss moduli dropped by at least one order of magnitude and reversed their magnitude indicating a gel to solution transition. Upon reducing the strain to 0.01%, the moduli reversed back to having a higher storage modulus than the loss modulus, demonstrating its healing back into a gel. | |
Discussion
We have demonstrated a method of synthesizing PGS-Br, a novel macroinitiator for the initiation of the robust ATRP reaction. Such a macroinitiator could be used for synthesis of a wide variety of PGS-based materials via ATRP with most ATRP-compatible monomers such as (meth)acrylates and (meth)acrylamides. Supramolecular assemblies based on macrocycles such as cyclodextrins34,36,45 and cucurbiturils46–49 have been reported previously. In this paper, we justified the feasibility of this PGS-Br macroinitiator with the synthesis of PGS–PEGMEMA as a component to the formation of a PGS–PEGMEMA/αCD biodegradable supramolecular hydrogel. This hydrogel had exhibited several exceptional properties.
This hydrogel system had a low minimum gelation concentration of only 5.2% while the control PEG/αCD hydrogel system had a minimum gelation concentration of 8.5%. This minimum gel concentration is comparable to the properties of thermogelling polymers previously reported by our group.37,50–53 This would necessarily imply a highly ordered and effective water-trapping matrix. This hydrogel system was also efficient as it took a short time for gel formation. By increasing the αCD concentration, the gelation time decreased steeply. From an industrial standpoint, a lower gelation concentration and faster gelation and therefore a shorter processing time would be considerably advantageous due to cost reduction in manufacturing.
The PGS–PEGMEMA hydrogel exhibited a low UCST temperature that could be easily tuned by varying the αCD concentration. By altering the αCD concentration in a range of 5–13% (w/v), the UCST of this current hydrogel system could be varied for a range as wide as 35 °C (from 55 °C to 90 °C). Further studies on modulating the PEGMEMA monomer chain length, molecular weight of the PGS–PEGMEMA co-polymer, ratio of PGS
:
PEGMEMA seeks to achieve an even wider range of UCST.
Lastly, the PGS–PEGMEMA/αCD hydrogel system was able to achieve moduli as high as 100 kPa with a short self-healing time. The moduli of a few kPa to 100 kPa is biologically relevant as the moduli of human soft tissue and cells are in the same range with cells in the range of a few kPa and tougher tissue such as muscles and arteries in the range of 100 kPa.54,55 Hydrogels with such a range of moduli would be very appropriate for further development in areas of cell encapsulation, tissue engineering, drug delivery and other biomedical and related applications.
Conclusion
In summary, PGS was modified at the hydroxyl handles using atom transfer radical polymerization (ATRP) by synthesizing a PGS macroinitiator. This macroinitiator was used to synthesize PGS–PEGMEMA. By mixing this water soluble polymer with α-CD, a PGS–PEGMEMA/αCD supramolecular hydrogel system is prepared. This hydrogel exhibited a tunable, low UCST of less than 90 °C, low minimum gelation concentration of 5.2%, rapid gelation and rapid self-healing ability with relatively high modulus (∼100 kPa) that is comparable to that of human soft tissue. This hydrogel system is injectable yet strong enough to provide support features, making it a very suitable candidate as a vehicle for biomedical related applications.
Acknowledgements
The authors would like to acknowledge the A*STAR Personal Care Grant (Project no. 1325400026) for support of this project.
References
- D. Kai, S. S. Liow and X. J. Loh, Mater. Sci. Eng., C, 2014, 45, 659–670 CrossRef CAS PubMed.
- D. Kai and X. J. Loh, ACS Sustainable Chem. Eng., 2014, 2, 106–119 CrossRef CAS.
- X. J. Loh, W. C. D. Cheong, J. Li and Y. Ito, Soft Matter, 2009, 5, 2937–2946 RSC.
- X. J. Loh, J. S. Gong, M. Sakuragi, T. Kitajima, M. Z. Liu, J. Li and Y. Ito, Macromol. Biosci., 2009, 9, 1069–1079 CrossRef CAS PubMed.
- Z. Li and X. J. Loh, Chem. Soc. Rev., 2015, 44, 2865–2879 RSC.
- Y. D. Wang, Y. M. Kim and R. Langer, J. Biomed. Mater. Res., Part A, 2003, 66, 192–197 CrossRef PubMed.
- Y. D. Wang, G. A. Ameer, B. J. Sheppard and R. Langer, Nat. Biotechnol., 2002, 20, 602–606 CrossRef CAS PubMed.
- C. A. Sundback, J. Y. Shyu, Y. D. Wang, W. C. Faquin, R. S. Langer, J. P. Vacanti and T. A. Hadlock, Biomaterials, 2005, 26, 5454–5464 CrossRef CAS PubMed.
- I. Pomerantseva, N. Krebs, A. Hart, C. M. Neville, A. Y. Huang and C. A. Sundback, J. Biomed. Mater. Res., Part A, 2009, 91, 1038–1047 CrossRef PubMed.
- Q. Q. Dou, S. S. Liow, E. Y. Ye, R. Lakshminarayanan and X. J. Loh, Adv. Healthcare Mater., 2014, 3, 977–988 CrossRef CAS PubMed.
- S.-L. Liang, W. D. Cook, G. A. Thouas and Q.-Z. Chen, Biomaterials, 2010, 31, 8516–8529 CrossRef CAS PubMed.
- Y. Li, W. D. Cook, C. Moorhoff, W.-C. Huang and Q.-Z. Chen, Polym. Int., 2013, 62, 534–547 CrossRef CAS PubMed.
- Q.-Z. Chen, A. Bismarck, U. Hansen, S. Junaid, M. Q. Tran, S. E. Harding, N. N. Ali and A. R. Boccaccini, Biomaterials, 2008, 29, 47–57 CrossRef CAS PubMed.
- G. C. Engelmayr Jr, M. Cheng, C. J. Bettinger, J. T. Borenstein, R. Langer and L. E. Freed, Nat. Mater., 2008, 7, 1003–1010 CrossRef PubMed.
- C. A. Sundback, J. Y. Shyu, A. J. Wu, T. P. Sheahan, Y. D. Wang, W. C. Faquin, R. S. Langer, J. P. Vacanti and T. A. Hadlock, in Architecture and Application of Biomaterials and Biomolecular Materials, ed. J. Y. Wong, A. L. Plant, C. E. Schmidt, L. Shea, A. J. Coury, C. S. Chen, A. E. Barron, H. A. Klok, W. M. Saltzman, A. Chilkoti, D. Luo and K. Uhrich, 2004, vol. 1, pp. 37–39 Search PubMed.
- M. J. Kim, M. Y. Hwang, J. Kim and D. J. Chung, BioMed Res. Int., 2014, 956952 Search PubMed.
- Y. D. Wang, B. J. Sheppard and R. Langer, in Biological Biomimetic Materials-Properties to Function, ed. J. Aizenberg, J. M. McKittrick and C. A. Orme, 2002, vol. 724, pp. 223–227 Search PubMed.
- A. Mahdavi, L. Ferreira, C. Sundback, J. W. Nichol, E. P. Chan, D. J. D. Carter, C. J. Bettinger, S. Patanavanich, L. Chignozha, E. Ben-Joseph, A. Galakatos, H. Pryor, I. Pomerantseva, P. T. Masiakos, W. Faquin, A. Zumbuehl, S. Hong, J. Borenstein, J. Vacanti, R. Langer and J. M. Karp, Proc. Natl. Acad. Sci. U. S. A., 2008, 105, 2307–2312 CrossRef CAS PubMed.
- C. L. E. Nijst, J. P. Bruggeman, J. M. Karp, L. Ferreira, A. Zumbuehl, C. J. Bettinger and R. Langer, Biomacromolecules, 2007, 8, 3067–3073 CrossRef CAS PubMed.
- M. J. N. Pereira, B. Ouyang, C. A. Sundback, N. Lang, I. Friehs, S. Mureli, I. Pomerantseva, J. McFadden, M. C. Mochel, O. Mwizerwa, P. del Nido, D. Sarkar, P. T. Masiakos, R. Langer, L. S. Ferreira and J. M. Karp, Adv. Mater., 2013, 25, 1209–1215 CrossRef CAS PubMed.
- A. Patel, A. K. Gaharwar, G. Iviglia, H. Zhang, S. Mukundan, S. M. Mihaila, D. Demarchi and A. Khademhosseini, Biomaterials, 2013, 34, 3970–3983 CrossRef CAS PubMed.
- J.-S. Wang and K. Matyjaszewski, J. Am. Chem. Soc., 1995, 117, 5614–5615 CrossRef CAS.
- X. J. Loh and Y.-L. Wu, Chem. Commun., 2015 10.1039/c5cc03686k.
- E. A. Appel, J. del Barrio, X. J. Loh, J. Dyson and O. A. Scherman, J. Polym. Sci., Part A: Polym. Chem., 2012, 50, 181–186 CrossRef CAS PubMed.
- X. J. Loh, J. Appl. Polym. Sci., 2013, 127, 992–1000 CrossRef CAS PubMed.
- X. J. Loh, S. J. Ong, Y. T. Tung and H. T. Choo, Macromol. Biosci., 2013, 13, 1092–1099 CrossRef CAS PubMed.
- X. J. Loh, S. J. Ong, Y. T. Tung and H. T. Choo, Polym. Chem., 2013, 4, 2564–2574 RSC.
- D. Linton, P. Driva, B. Sumpter, I. Ivanov, D. Geohegan, C. Feigerle and M. D. Dadmun, Soft Matter, 2010, 6, 2801–2814 RSC.
- B. Mendrek, L. Sieron, M. Libera, M. Smet, B. Trzebicka, A. L. Sieron, A. Dworak and A. Kowalczuk, Polymer, 2014, 55, 4551–4562 CrossRef CAS PubMed.
- S. Banerjee, T. Maji and T. K. Mandal, Colloid Polym. Sci., 2014, 292, 2217–2226 CAS.
- B. Ou, Z. Zhou, Q. Liu, B. Liao, S. Yi, Y. Ou, X. Zhang and D. Li, Polym. Chem., 2012, 3, 2768–2775 RSC.
- H. Liu, W. Z. Zhou, H. Q. Ye, K. Han, S. C. Hou and X. Y. Zhang, J. Cent. South Univ., 2014, 21, 3049–3056 CrossRef CAS.
- C. Travelet, G. Schlatter, P. Hébraud, C. Brochon, A. Lapp and G. Hadziioannou, Langmuir, 2009, 25, 8723–8734 CrossRef CAS PubMed.
- P. L. Chee, A. Prasad, X. T. Fang, C. Owh, V. J. J. Yeo and X. J. Loh, Mater. Sci. Eng., C, 2014, 39, 6–12 CrossRef CAS PubMed.
- X. J. Loh, Mater. Sci. Eng., C, 2014, 45, 599 CrossRef CAS PubMed.
- X. J. Loh, Mater. Horiz., 2014, 1, 185–195 RSC.
- X. J. Loh, H. X. Gan, H. Wang, S. J. E. Tan, K. Y. Neoh, S. S. J. Tan, H. F. Diong, J. J. Kim, W. L. S. Lee, X. T. Fang, O. Cally, S. S. Yap, K. P. Liong and K. H. Chan, J. Appl. Polym. Sci., 2014, 131, 39924 CrossRef PubMed.
- E. A. Appel, J. del Barrio, X. J. Loh and O. A. Scherman, Chem. Soc. Rev., 2012, 41, 6195–6214 RSC.
- E. A. Appel, X. J. Loh, S. T. Jones, F. Biedermann, C. A. Dreiss and O. A. Scherman, J. Am. Chem. Soc., 2012, 134, 11767–11773 CrossRef CAS PubMed.
- E. A. Appel, X. J. Loh, S. T. Jones, C. A. Dreiss and O. A. Scherman, Biomaterials, 2012, 33, 4646–4652 CrossRef CAS PubMed.
- P. Thoniyot, M. J. Tan, A. A. Karim, D. J. Young and X. J. Loh, Advanced Science, 2015, 2, 1400010 CrossRef PubMed.
- J. Li, NPG Asia Mater., 2010, 2, 112–118 CrossRef PubMed.
- J. Li, A. Harada and M. Kamachi, Polym. J., 1994, 26, 1019–1026 CrossRef CAS.
- A. Harada, J. Li and M. Kamachi, Nature, 1992, 356, 325–327 CrossRef CAS PubMed.
- J. Li and X. J. Loh, Adv. Drug Delivery Rev., 2008, 60, 1000–1017 CrossRef CAS PubMed.
- E. A. Appel, M. J. Rowland, X. J. Loh, R. M. Heywood, C. Watts and O. A. Scherman, Chem. Commun., 2012, 48, 9843–9845 RSC.
- X. J. Loh, J. del Barrio, T. C. Lee and O. A. Scherman, Chem. Commun., 2014, 50, 3033–3035 RSC.
- X. J. Loh, J. del Barrio, P. P. C. Toh, T. C. Lee, D. Z. Jiao, U. Rauwald, E. A. Appel and O. A. Scherman, Biomacromolecules, 2012, 13, 84–91 CrossRef CAS PubMed.
- U. Rauwald, J. del Barrio, X. J. Loh and O. A. Scherman, Chem. Commun., 2011, 47, 6000–6002 RSC.
- X. J. Loh, L. W. I. Cheng and J. Li, in Modern Trends in Polymer Science-Epf 09, ed. F. Stelzer and E. Wiesbrock, 2010, vol. 296, pp. 161–169 Search PubMed.
- X. J. Loh, W. Guerin and S. M. Guillaume, J. Mater. Chem., 2012, 22, 21249–21256 RSC.
- X. J. Loh, P. N. N. Vu, N. Y. Kuo and J. Li, J. Mater. Chem., 2011, 21, 2246–2254 RSC.
- V. P. N. Nguyen, N. Y. Kuo and X. J. Loh, Soft Matter, 2011, 7, 2150–2159 RSC.
- M. N. S. Tatyana, G. Kuznetsova, N. I. Yegorenkov, S. A. Chizhik and R. I. Zhdanov, Micron, 2007, 38, 824–833 CrossRef PubMed.
- R. Akhtar, M. J. Sherratt, J. K. Cruickshank and B. Derby, Mater. Today, 2011, 14, 96–105 CrossRef CAS.
|
This journal is © The Royal Society of Chemistry 2015 |