DOI:
10.1039/C5RA07616A
(Paper)
RSC Adv., 2015,
5, 58504-58513
New photocatalyst for allylic aliphatic C–H bond activation and degradation of organic pollutants: Schiff base Ti(IV) complexes†
Received
26th April 2015
, Accepted 17th June 2015
First published on 17th June 2015
Abstract
Schiff base metal complexes have attracted significant attention due to their alternative applications in the environment, such as the mineralization of organic pollutants to less harmful byproducts and oxygen generation via such processes as photosynthesis. The Ti4+ complexes obtained from Schiff base ligands reacted with donor atoms such as S and N under solvothermal conditions. These complexes were characterized using microanalysis, conductivity studies, and different spectral techniques. These data reveal that the compounds show distorted octahedral geometries with ligand coordination via azomethine nitrogen and thiol sulfur atoms. Oxidation of the allylic methyl group examined the photocatalytic activity of [Ti(L)O] under ambient conditions and oxidative cyclization under visible light irradiation. The Ti(DCMPPT)O complex is a very efficient catalyst due to the very short span of time it takes to produce aldehydes from allylic compounds. Aldehydes readily react with 2-aminophenol or 2-aminobenzenethiol to produce (E)-2-styrylbenzo[d]oxazoles and (E)-2-styrylbenzo[d]thiazoles due to the suitability of the bandgap energy to the generation of ˙OH radicals during the catalytic reaction. This results in a higher oxidation rate. [Ti(DCMPPT)O] is a very efficient photocatalyst for the degradation of MB, due to its large surface area which suggests a lower recombination energy mechanism.
Introduction
Metal–organic oxides are very prominent and efficient catalysts for both oxidative coupling and C–H activation of organic compounds in organic synthesis.1 Schiff base complexes were used as catalysts for oxidation, whereas they are rarely used for the photodegradation of organic pollutants.2 The photooxidation processes of a broad range of organic pollutants over binary and ternary metal oxides have been studied throughout the past two decades3 due to the fact that large amounts of pollutants that are discharged from pharmaceutical or textile industrial effluents spoil the natural ecosystems in the surroundings of industrial areas and cause perturbations in the aquatic life.4 Most of these dyes are highly toxic and cause severe environmental pollution by discharging dangerous byproducts into the aqueous phase. The purification of industrial waste water is a challenging task for reusable techniques such as biological procedures, physical and chemical methods, and photocatalytic oxidation.5 However, these methods are highly expensive and not sensitive to the degradation of the toxic substances. Substantial work has been carried out on the photodegradation of dyes in the presence of metal oxides. However, this depends on various factors such as the phase purity, surface area, crystallite size, amount of catalyst, nature of the dopants and the method of preparation.6 Even though intrinsic semiconductor metal oxides exhibit poor efficiency due to their high bandgap energy, non-homogenous size of particles and high recombination energy.7 However, anionic or cationic doped metal oxides exhibit moderate to high photodegradation of dyes under visible light irradiation.8 In this mechanism the degradation of organic pollutants depends on the stability and non-selectivity of ˙OH radicals. Frequently, the methods used for the purification of water are advanced oxidation processes (AOPs) due to produce ˙OH radicals under UV light irradiation, bicarbonate ions are present in water sample ˙OH radicals are unstable and hence this process is very expensive. For this reason, alteration of the method is required for the purification of water under visible light conditions.
Heterocyclic moieties contain conjugated double bonds, hence complexation of transition metal ions and heteroatoms naturally occurs. This is due to the flexibility in oxidation state and the presence of unpaired electrons coordinated to the metal ions through an oxygenation process.9 Therefore, for this reason, transition metal complexes play an important role in influencing the biological, medicinal, chemical, photophysical and photochemical properties of the organic ligands. Hence, metal complexes for the degradation of dyes and C–H activation of organic compounds have been published from time to time.10 From the earlier reports, it is noted that in most cases hydrogen peroxide played a vital role by producing sensitive species such as ˙OH radicals which were used for the mineralization of organic pollutants. However, a high concentration of H2O2 is unsafe, and it is injurious to the eyes, lungs and skin. Recently, some Ni(II) Schiff base complexes have been used as photocatalysts to degrade MB and rhodamine-B in the absence of H2O2 under visible light irradiation.2,11 These Schiff base complexes are pH dependent catalysts and in higher pH solution which becomes strongly basic, the catalysts are unstable and not reusable. The oxidation of benzhydrol to benzophenone and photodegradation of rhodamine-B in the presence of zeolite captured Ni(II) and Cu(II) complexes with a N2O2 donor system of Schiff base ligands under visible light irradiation has been reported.12 A recently published article revealed photocatalytic hydrogen evolution by a cobalt complex based on a tripodal iminopyridine ligand under visible light irradiation.13 Some research is focused on metal-oxide frameworks which are prepared and converted into respective metal oxide nanoparticles. Finally, the metal oxide nanoparticles are used for the photodegradation of dyes.14
In general, titanium oxides (such as binary and ternary) have shown significant contribution to the field of photocatalysis.15 TiO2 and its doped materials (anionic and cationic) are semiconducting photocatalysts that are very frequently used for the degradation of organic pollutants under UV and visible light irradiation conditions.16–18 However, the actual applications of TiO2, which has a bandgap of 3.2 eV (for anatase), in the visible light region are very specific due to its wide bandgap energy. Recently, O
Ti containing complexes have been proposed as photocatalysts for water splitting in a computational study.19 The ligand part acts as a probe (antenna) under visible light and the O
Ti group exhibits the redox properties needed for the oxidation of water. A few studies on the electronic nature and structural properties of O
Ti porphyrins in water have been reported.20 Ti(IV) complexes have been used for polymerization reactions,21–23 but there are a few gaps found in the field such as the degradation of dyes and C–H activation studies.23 For this reason, Ti(IV) complexes are used towards the advancement of visible-light-sensitive photocatalysts. Hence, the pursuit of higher stability, recyclability, and an affordable methodology still occupies researchers. Photocatalysis using metal–organic complexes under visible light has attracted significant attention due to its prospective applications in eco-friendly remediation by degrading organic pollutants to very safe byproducts due to the absorption of toxic gasses.24–26 In this study, new Schiff base Ti(IV) complexes synthesized from N, S atoms coordinated and modification of absorption bands of shifted towards higher wavelength regions.
Experimental
All reagents were purchased from Sigma-Aldrich and used without further purification, such as tetraisopropoxytitanium, 2-aminobenzenethiol, 2-iodo-4,5-dimethylaniline, 4,5-dichloro-2-iodoaniline, toluene and other solvents. 2-Thioxo-1,2-dihydropyridine-3-carbaldehyde was obtained from Synocule Research Lab Pvt. Hyderabad, India. 2-Iodo-4,5-dimethylaniline and 4,5-dichloro-2-iodoaniline were converted into 2-amino-4,5-dimethylbenzenethiol and 2-amino-4,5-dichlorobenzenethiol as per the procedures reported in the literature.27,28
In our attempts to synthesise Schiff base ligands using aldehyde and amines, the prepared new ligands, i.e. (E)-3-(((2-mercaptophenyl)imino)methyl)pyridine-2-thiol (MPPT), (E)-3-(((2-mercapto-4,5-dimethylphenyl)imino)methyl)pyridine-2-thiol (DMPMPT) and (E)-3-(((4,5-dichloro-2-mercaptophenyl)imino)methyl)pyridine-2-thiol (DCMPPT), containing two thiols and one imine group were characterized by mass, SEM, IR, Raman, UV and NMR spectral investigations. The Ti(IV) complexes were prepared using Schiff base ligands and were characterized. The photocatalytic oxidative cyclization and photodegradation of MB were studied, and all of the experimental procedures are presented in the ESI.†
Synthesis of Schiff base ligands (Scheme 1)
Preparation of Schiff base ligands. 2-Aminobenzenethiol/2-amino-4,5-dimethylbenzenethiol/2-amino-4,5-dichloro-benzenethiol (25 mmol) was added to a solution of 2-mercaptonicotinaldehyde (25 mmol) and piperidine (5 mmol) in toluene (100 ml) at room temperature. The resulting solution was allowed to reflux for 16 h. The reaction mixture was cooled and the solvent removed to give a residue, followed by a standard aqueous workup affording the corresponding ligands above, Scheme 1.
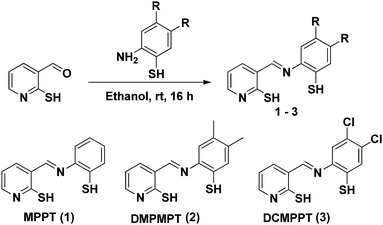 |
| Scheme 1 Synthesis of Schiff base ligands. | |
Preparation of Ti(IV) complexes (Scheme 2). The Schiff base ligand (MPPT, DMPMPT and DCMPPT) (1.00 mmol) and Ti(OiPr)4 (0.30 ml, 1.00 mmol) were dissolved in toluene (30 ml) then heated at 80 °C under solvothermal conditions for 8 h. The solvent was evaporated under reduced pressure and recrystallized to yield microcrystalline materials 4–6 with yields of the materials of 48–54%.
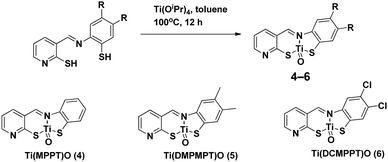 |
| Scheme 2 Preparation of Ti(IV) complexes. | |
Characterization of photocatalysts
CHN analyses are estimated by using an Elemental Analyzer Flash EA 1112. The conductance of the metal complexes was measured on a Digisun digital conductivity meter model DI-909. Mass spectra data were collected by HR-EI systems on a JMS-700 double focusing mass spectrometer (JEOL, Tokyo, Japan). Thermograms of all of the samples were obtained using a Shimadzu differential thermal analyzer (DTG-60H) at a heating rate of 10 °C min−1. The SEM-EDS images were obtained on a HITACHI SU-1500 variable pressure scanning electron microscope (VP-SEM). X-ray photoelectron spectroscopy (XPS) was performed on a KRATOS AXIS165 X-ray photoelectron spectrometer using an excitation energy of 1253.6 eV (Mg Kα) and a pass energy of 80 eV. FT-IR spectra were recorded using a Shimadzu spectrometer with samples prepared on KBr pellets. Infrared spectra (IR; KBr disc) was registered with a PerkinElmer BX series; Fourier transform infrared spectrophotometer. Raman spectra were recorded using a 632.81 nm line from a He–Ne laser and the scattered light was analyzed using a HORIBA JOBIN YVON HR800. The laser was focused on a spot of ∼3 lm, and a 109 lens was used for the collection of backscattered Raman signal. 1H and 13C{1H} NMR spectra were recorded on a Bruker AV400 MHz Spectrometer with chemical shifts referenced using the 1H resonance of residual CHCl3. Melting points were verified on a Cintex apparatus. The electronic spectra were obtained in chloroform solutions on a JASCO V-650 UV-Vis spectrophotometer. Brunauer–Emmett–Teller (BET) surface areas were determined by nitrogen adsorption–desorption isotherm measurements at 77 K on a Quantachrome autosorb automated gas sorption system. The photoluminescence (PL) spectra of the catalysts were recorded on a Cary Eclipse Fluorescence Spectrophotometer and the samples were excited at their respective absorption maxima.
Results and discussion
All of the analytical data for the ligands and complexes are presented in Table 1 and the data agree with the calculated values. The ligands MPPT, DMPMPT, and DCMPPT synthesized from the aldehyde react with various amino-thiol compounds in a methanol media and the high yields indicate that the reaction conditions were correct. The ligands are highly stable and fairly soluble in polar organic solvents such as EtOH, MeOH, and CH3CN due to the presence of thiol and azomethine groups. The synthesized metal complexes are stable at room temperature and soluble in DMSO and DMF. The morphologies of all ligands and Ti-complexes were acquired by scanning electron microscopy (SEM). This technique enables determination of the discrete morphology of the ligands and Ti(IV) complexes. The SEM images of the ligands and complexes (Ti(MPPT)O, Ti(DMPMPT)O and Ti(DCMPPT)O) are shown in Fig. S1† and 1, respectively. The surface morphology of the parent Ti(MPPT)O, Ti(DMPMPT)O and Ti(DCMPPT)O samples is characterized by densely packed particles with considerable agglomeration, whereas the Ti4+ ion complexation to the produced complexes was smaller than that of the respective ligands.29 The different morphologies of the Ti(IV) complexes is noticeable in the manifestation of the new product. The morphologies of the Ti(IV) complexes are established in distinct dimensions and profiles. Nevertheless, they are synthesized from a single ligand. The morphology of the Ti(MPPT)O complex (Fig. 1a and S1†) is found to be a cube type form as well as appearing like a stack of blocks, whereas the MPPT ligand exists in mixed forms. Similarly, the Ti(DMPMPT)O and Ti(DCMPPT)O morphologies are cubes and hexagonal type profiles (Fig. 1b and c). However, the ligands DMPMPT and DCMPPT are found as mixture shapes and plate type profiles (Fig. S1†).
Table 1 Analytical and physicochemical data of the Ti(IV) complexes
Compound |
Mol. wt |
m/z [M+ + 1] |
% yield |
Surface area (m2 g−1)/bandgap energy (eV) |
Mp/Dc. (°C) ± 2 |
% C |
% H |
% N |
% Cl |
S% |
% Ti |
Λm (ohm−1 mol−1 cm2) |
MPPT |
246.35 |
247.3 |
85 |
16/3.26 |
142 |
58.46 (58.51) |
4.06 (4.09) |
11.42 (11.37) |
— |
23.28 (23.37) |
— |
12.4 |
DMPMPT |
270.40 |
271.4 |
78 |
11/3.39 |
163 |
61.25 (61.28) |
5.12 (5.14) |
10.27 (10.21) |
— |
20.26 (20.37) |
— |
11.6 |
DCMPPT |
315.24 |
316.2 |
81 |
21/3.17 |
186 |
45.66 (45.72) |
2.51 (2.56) |
8.91 (8.89) |
22.53 (22.49) |
20.29 (20.34) |
— |
10.1 |
Ti(MPPT)O |
308.20 |
309.1 |
48 |
31/2.99 |
252 |
63.13 (46.76) |
2.58 (2.62) |
9.17 (9.09) |
— |
20.73 (20.81) |
15.39 (15.53) |
10.9 |
Ti(DMPMPT)O |
336.26 |
337.26 |
51 |
23/3.12 |
318 |
49.96 (50.01) |
5.54 (3.60) |
8.23 (8.33) |
— |
18.98 (19.07) |
14.06 (14.24) |
11.1 |
Ti(DCMPPT)O |
377.09 |
378.09 |
54 |
43/2.64 |
334 |
38.15 (38.22) |
1.63 (1.60) |
7.36 (7.43) |
18.59 (18.80) |
16.94 (17.01) |
12.49 (12.69) |
10.8 |
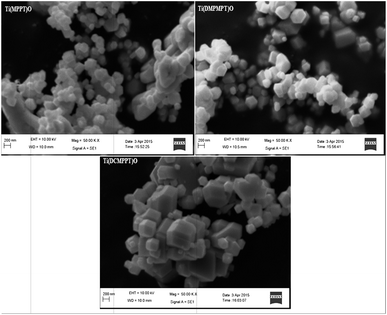 |
| Fig. 1 SEM images of Ti(MPPT)O, Ti(DMPMPT)O and Ti(DCMPPT)O. | |
The molar conductance of the metal complexes was measured for 10−3 M solutions in DMF and the results are presented in Table 1. The complexes have Ωm values in the range 10.0–12.0 ohm−1 cm2 mol−1 that fall within the range of non-electrolytes.30 All of the Ti(IV) complexes were dried under vacuum and heated at 120 °C for two hours. All of the complexes were thermally stable up to 250 °C and underwent decomposition in one step (Fig. S2†). The single step decomposition (endothermic) ensues between 250 and 580 °C with a weight loss that corresponds to the loss of the ligand molecule.31,32 The weight of the residue remaining in the crucible signified the formation of TiOS. The residue obtained from TGA is examined by XPS.
The XPS data for all of the residues in the complexes reveal the same pattern, as shown in Fig. 2. The peaks present in the spectrum of each complex have the same positions. XPS reveals that the Ti(IV) complexes give significant information for complex formation through S, N and O atoms. Ti 2p, O 1s, C 1s and S 2p peaks are shown. The XPS spectrum of Ti 2p is unchanged with the varying of ligands but a very small change in the S 2p peak position as compared with the Ti(MPPT)O complex as shown in Fig. 2 is due to both the donating and weakly withdrawing groups present in the ligands of the other two complexes.33 The spectral data gives significant information about the complexes, i.e. a 1
:
1 ratio of ligand and Ti-metal ion, and supports the TGA and elemental analysis data. The mass spectral studies of the ligands and Ti(IV) complexes were recorded and the obtained data correlate with the calculated data, given in the ESI (Fig. S3†).
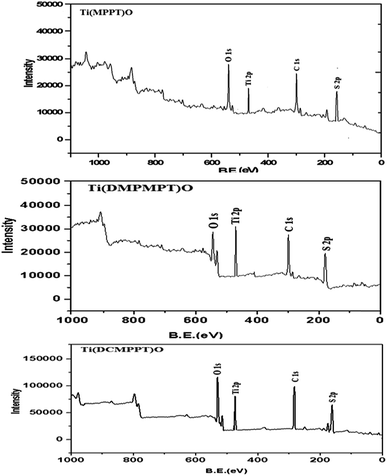 |
| Fig. 2 XPS images of Ti(MPPT)O, Ti(DMPMPT)O and Ti(DCMPPT)O. | |
The high-resolution mass spectra of the ligands show molecular ion peaks as well as a base peak at 246 (MPPT), 274 (DMPMPT) and 315 (DCMPPT) (M)⌉+. This confirms that the formulae of the ligands are C12H10N2S2 (MPPT), C14H14N2S2 (DMPMPT) and C12H8Cl2N2S2 (DCMPPT). The peaks shown in the mass spectra are equivalent to the ligands and their metal complexes to Schiff base fragmentation. The mass spectra of the complexes show molecular ion peaks ([M]+, 100%) at m/z 308.1 [Ti(MPPT)O], 336.26 [Ti(DMPMPT)O] and 377.32 [Ti(DCMPPT)O]. For this reason, all of the complexes exist with 1
:
1 ratios of Ti and ligands.30 The surface areas of the ligands and complexes were measured and are presented in Table 1. The order of surface area is DMPMPT < MPPT < DCMPPT < Ti(DMPMPT)O < TI(MPPT)O < TI(DCMPPT)O. Therefore, Ti(DCMPPT)O exhibits better photocatalytic activity compared to the other two complexes. The IR spectra of all of the ligands show the presence of two common functional groups, azomethine and thiol. The spectra of the ligands exhibit two significant bands at approximately 3090 and 1614 cm−1 which are due to the stretching vibration of the –SH group and azomethine v(C
N) group for the ligands MPPT, DMPMPT, and DCMPPT. The IR spectra of the complexes were equated with those of the ligands to define the coordination positions that may be involved in chelation. There are some significant peaks in the spectra of the ligands, which were cooperative in achieving this complexation. The location and/or intensities of these peaks are expected to change upon chelation. The bands shifted to lower a wavenumber region by a range of 10 to 15 cm−1 in all of the complexes.34 This represents participation of the azomethine nitrogen. The new bands that appeared in the spectra of all of the complexes are v(Ti–N) and (Ti–S). The most characteristic bands appeared in the spectra of the complexes in the areas 392, 388 and 374 (thiophenylic S). These new absorption peaks are assigned to the v(Ti–S) stretching vibration modes for the Ti(IV) complexes. The other prominent bands at 422, 416 and 412 in MPPT, DMPMPT and DCMPPT of the Ti(IV) complexes,35 respectively, have been consigned to the v(M–N) mode. For this reason, from the IR spectra it is established that MPPT, DMPMPT, and DCMPPT behave as tridentate ligands coordinated to the Ti ions via azomethine-N, pyridine-S and thiophenylic-S and a Ti–O band was also revealed. However, this band is present in the range of the TiO band at 1022 cm−1, which indicated that all of the complexes contain a Ti
O bond36 (Fig. S4a–c†). For this reason, it is concluded that all complexes have a 1
:
1 ratio of Ti and ligands, from the molar conductivity data, TGA, mass and IR studies. The Raman spectra of all of the Ti(IV) complexes are shown in Fig. 3. The O
Ti stretching mode of O
Ti(IV) was also consigned, which has been described in O
Ti containing porphyrins.20c,37 The O
Ti bond is considered to result from the σ-donor between the filled O2− pz orbital and empty Ti4+ dz2 orbital, and the π-donor interactions between the filled px and py orbitals and the vacant dxz and dyz orbitals. This results in a strong O
Ti bond, which is reflected in the high stretching frequency at 920 cm−1. The Raman data of all of the complexes show that the phenyl ring, azomethine, pyridine-N and aryl-S peaks are identified as per the reported data.38
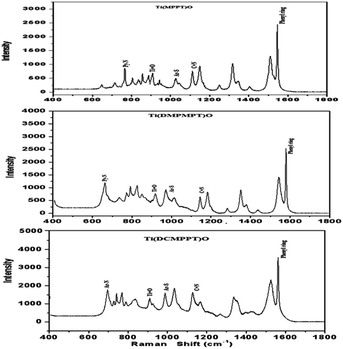 |
| Fig. 3 Raman spectra of Ti(MPPT)O, Ti(DMPMPT)O and Ti(DCMPPT)O. | |
The UV-visible spectra of ligands L1 to L3 recorded in methanol show sharp and broad absorption bands at approximately 362 and 294 nm. The first lowest energy band arises because of the n → π* transition from the central ring to the aromatic ring. The higher energy band observed at 232 nm is due to the π → π* transitions (Fig. S5†). In all of the spectra of ligands L1 to L3, the bands are onset at 365 to 391 nm, which indicates that the bandgap of the ligands was between 3.39 and 3.17 eV. Therefore, the reaction between the aldehyde and aromatic amines to give the Schiff base. The ligand consists a donating group is present HOMO as shifted downwards, and in the case of withdrawn group is present LUMO shifted direction downwards. Theoretical calculations was studied in the presence of donating and withdrawn groups by K. Kaleta et al.39 and bands shifted to higher wavelength region depends on small dipoles groups present on the system.
In the complexes with the metal (Ti(IV)) the n–π* transition slightly shifts to a lower value, signifying coordination of the ligand to the metal and the bands are onset towards a longer wavelength region.40 The change in the onset values of the complexes, which indicate that the bandgap energies of the complexes are marginally changed compared to the ligands, is shown in Fig. 4. Since the Ti4+ ion has a d0 arrangement, there is no chance of a d–d transition. The wideness of the band can be taken as a suggestion of a distorted octahedral geometry.
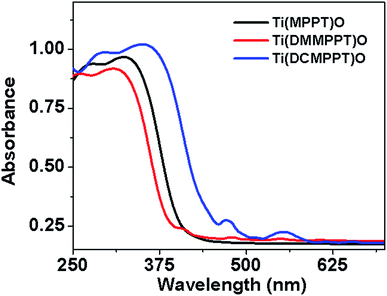 |
| Fig. 4 Kubelka–Munk-transformed diffuse reflectance spectra (DRS) of all of the complexes. | |
The purity of the ligands (MPPT, DMPMPT, and DCMPPT) is also evidenced from 1H NMR spectra recorded in d6-DMSO (Fig. S6†). In these ligands, two important characteristic peaks are present; one azomethine one thiol proton. The most typical and characteristic signals revealed by all of the ligand spectra are at δ values in the range of 8.9, 7.2–8.5, 5.8–6.2 and 3.4 ppm, due to the existence of the protons of –CH
N, ArHs, pyridine–SH, and phenolic–SH, respectively. The signal exposed at δ 2.42 ppm is attributed to the methyl group protons present in the ligand DMPMPT. The 13C-NMR spectra of all of the ligands exhibit one standard carbon atom at ∼160 ppm that supports the above NMR data, and this carbon atom is from the azomethine group. Another important peak present in all ligand spectra is a functional group attached to the various aromatic carbon atoms, that are Py-C–SH and Ar-C–SH at 180 and 125 ppm, respectively. All of the ligand spectra show peaks from 125 to 156 ppm. These peaks are belong to aromatic ring carbon atoms.
The NMR spectra of the complexes are compared with the ligands (SH’s) and one of the characteristic peaks are absent, indicating the formation of Ti–S bonds (Fig. S7†). As azomethine nitrogen atoms participated in complexation with Ti(IV) ions, the protons of the azomethine group were shielded in the complex spectra.41 Finally, the remaining aromatic protons also moved towards lower ppm values. These data revealed that the Schiff base ligands were complexed with Ti(OiPr)4 and all of the complexes had distorted octahedral geometries.
Oxidative cyclization
In allylic oxidations a very frequently used catalyst is SeO2.42–44 However, purification of the final products is a very lengthy process as Se reacts with the substrate, forming unwanted byproducts. Therefore, metal–organic frameworks act as solid heterogeneous catalysts for the synthesis of nitrogen atom enclosing heterocycles.45 Oxidative cyclization is one of the most significant reactions in organic synthesis. Benzoxazoles and benzothiazoles are crucial scaffolds in various fields of research such as medicinal and materials chemistry.46–50 Stilbenes and Styryl group-containing systems are mimic in the structural features and for this reason, styryl substituted heterocycles are used as cis–trans molecular switches under UV-visible light.51,52 The synthesis of new analogues of stilbenes is essential for the development of molecular electronics. Synthetic conditions that do not affect the environment (both aquatic & soil), support sustainability of the catalyst for the number cycles in the solvent medium and utilize green energy sources, are critical for the preparation of materials. For this reason, we are focusing on better conditions, a modest catalyst based on Ti(IV) complexes. Product formation of the reaction was continuously monitored through the UV-visible spectra of the reaction mixture after every 10 min as shown in Fig. 5.
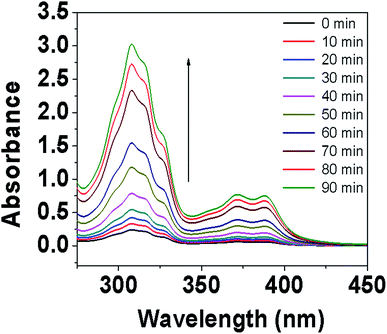 |
| Fig. 5 Temporal absorbance spectral pattern of the reaction mixture during the oxidative cyclization process on Ti(DCMPPT)O. | |
All of the substrates can be converted to the corresponding products in excellent yields with a moderate reaction time in the presence of the Ti(DCMPPT)O complex, compared with the other complexes. All of the reactions were run at the same time intervals by optimization with the substrate prop-1-en-1-ylbenzene, represented in Scheme 3 in the ESI†. The yields usually ranged between 76 and 95% with respect to the Ti(IV) complex. The product was isolated and purified using simple recrystallization from ethanol, resulting in a still quite impressive isolated yield of 80%.
All of the Ti(IV) complexes of MPPT, DMPMPT and DCMPPT were used as catalysts for the oxidation of prop-1-en-1-ylbenzene, converted into cinnamaldehyde. Cinnamaldehyde condensation with 2-aminophenol and finally dehydrogenation gave the respective styryl derivatives.53 For ease of evaluation with other catalytic systems, we performed the oxidation on a millimole scale using 0.5 mol% catalysts. The reaction conditions in the presence of the catalyst were also studied. The Ti(MPPT)O, Ti(DMPMPT)O and Ti(DCMPPT)O catalysts are added to 1
:
1 ratio of ethanol and water was used as the solvent for the system. All of the reactions were monitored by TLC. Within 90 min of the termination of the reaction, the yield of the corresponding product was shown from TLC and no further reaction was observed. Theoretically, only two equivalents of ˙OH radicals were consumed for the oxidation of the allyl methyl group to afford the primary alcohol. After one more hydroxyl radical attached to the primary alcohol to obtain the aldehyde, under solar light irradiation hydroxyl radicals were produced in situ. For this reason, our method is eco-friendly, and the stability of the catalysts means they are reusable. The catalyst was used for the preparation of various functional groups present on (E)-prop-1-en-1-ylbenzene to give the styryl product as shown in Table S1.† The oxidative cyclization reaction was continuously monitored by using UV-visible spectra as illustrated in Fig. 5. These spectra indicate that the passing of time increased the intensity of the new absorption band up to 90 min. Moreover, the photoreaction is continuous for 180 minutes; no change in the absorption band is observed and the stability of the product is high in the solution. All of the final products were confirmed from 1H-NMR spectra as shown in Fig. S8a & b.†
Photodegradation of methylene blue (MB)
MB is extensively used as an indicator for acid–base titrations in the laboratory and in the textile industry as an organic dye. Organic dyes are used for dying agents in various applications in daily life have the advantage of high solubility in water. At the same time, this enhances aquatic pollution, and for this reason the degradation of dyes is crucial for saving the environment.54 Here, we examine the photoactivity of the catalyst towards the pollutant MB . Therefore, we have selected MB as the model contaminant to evaluate the photocatalytic ability of Ti(MPPT)O, Ti(DMPMPT)O and Ti(DCMPPT)O under visible light irradiation. The experiments were carried out in the presence and absence of light. The dark experiments were conducted to create the adsorption–desorption equilibrium. After the one-hour dark test, the reaction was exposed to light irradiation and the decrement of the concentration of aqueous MB dye over the catalysts with increasing time intervals was observed. With the increase of time, the color of MB disappeared progressively. Fig. 6 reveals the characteristic photodegradation efficiencies of Ti(MPPT)O, Ti(DMPMPT)O and Ti(DCMPPT)O. From Fig. 6, it can be clearly seen that Ti(DCMPPT)O exhibited higher activity than that of its parent analogues. The extent of MB degradation by Ti(MPPT)O, Ti(DMPMPT)O and Ti(DCMPPT)O during 120 min of visible light irradiation is found to be 88%, 78% and 94%, respectively. It is well-known that an aqueous solution of MB undergoes self-photolysis under visible light radiation. To distinguishe between the photocatalysis and photolysis of MB, experiments were carried out in the presence and absence of the catalysts under identical conditions. The degradation curve obtained without the addition of the photocatalyst (Fig. 6, MB photolysis) shows only about 25% degradation. Therefore, the results indicate that the degradation of MB increases considerably in the presence of the catalysts due to photodegradation only and not due to the photosensitization of MB. After 20 min of visible light irradiation the sample was subjected to mass spectral analysis. The data revealed that some significant fragment peaks and a degradation path was proposed as follows (Fig. S9†).
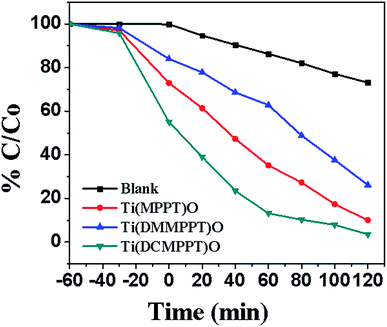 |
| Fig. 6 Degradation curves of methylene blue solution under visible light irradiation over all photocatalysts. | |
Mass spectral studies of methylene blue after 20 minutes
The efficiency and sustainability of all of the Ti-complexes depend on their chemical and photostability after the photoreaction and their capability to catalyze MB in several cycles. As shown in Fig. 7, the photocatalysts exhibit identical performance even after the third cycle. The 1H-NMR spectrum of Ti(DCMPPT)O matched with that of unused Ti(DCMPPT)O after the three cycles, indicating the stability of the complex (Fig. 8). Nevertheless, the other two complexes did not show the same amount of degradation as compared with the first cycle and even the 1H-NMR spectra of the recovered materials were not equivalent and very complex in nature. For this reason, Ti(DCMPPT)O is better than the other two Ti-complexes due to its high surface area and low bandgap energy. As the DCMPPT ligand contains chlorine atoms which handle the increased the photocatalytic activity as the electron affinity is increased, the electronic coupling increased then the recombination energy decreased.55
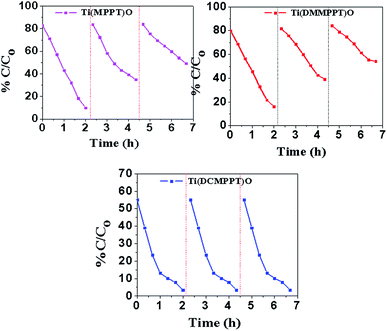 |
| Fig. 7 Recyclability of the Ti(IV) complexes for the photodegradation of MB under visible light irradiation. | |
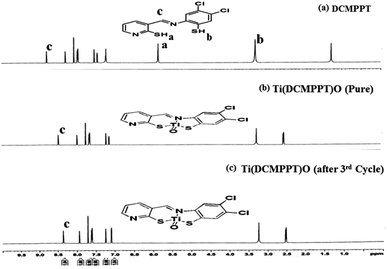 |
| Fig. 8 1H-NMR spectra of (a) ligand DCMPPT; (b) pure Ti(DCMPPT)O and (c) after the third cycle. | |
Mechanistic studies of photodegradation process
The oxidative cyclization process is one of the most significant methods for process development. However, the mechanistic study of product formation in the presence of solar energy is essential for material design, in which either ˙OH or O2˙− radicals handle both the oxidative cyclization and degradation of the pollutants.56 The production of ˙OH radicals in a photoreaction is as follows: the incident light energy is larger or equivalent to the bandgap energy of the Ti(IV) complex. Electrons will be transferred from the valence band to the conduction band, creating a hole in the valence band (eqn (1)). Both excited electrons and holes move to the surface of the Ti-complex and interact with adsorbed O2 and water to produce O2˙− and ˙OH radicals, respectively (eqn (3)).
H+ ion reacts very quickly with O2˙− radicals to form HO2˙ (eqn (4)) species that react among themselves and form H2O2 and O2 (eqn (5)). Subsequently, H2O2 decomposes to form ˙OH radicals (eqn (6)) that react at the allylic position to give the obtained aldehydes, Schiff base, and cyclization as shown in the proposed mechanism pathway-1. All the dye molecules interacts with ˙OH radicals non-selectively and non-direction with the dye (MB) which decomposes into a simple mineral. The mechanistic pathway-1 and 2 are shown below.
Proposed mechanism for oxidative cyclization (pathway-1)
Possible degradation mechanism of MB (pathway-2)
|
Catalyst + hv → ecb− + hvb+
| (1) |
|
H2O + hvb+ → O˙H + H+
| (2) |
|
Dye + O˙H → mineralization
| (7) |
For the proposed mechanism, the interaction of hydroxyl radicals with the dye or C–H activation is supported by the evidence. For this reason, we chose the photocatalytic hydroxylation of terephthalic acid (TA) as further evidence of the facility of the photocatalysts to create ˙OH radicals.57 It is well-known that the ˙OH radical attacks TA to form 2-hydroxy terephthalic acid (TAOH), which radiates a unique fluorescence signal at 440 nm. A photocatalytic test with TA was conducted in the presence of the Ti-complexes (Fig. 9).
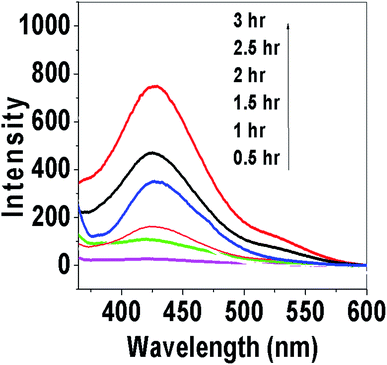 |
| Fig. 9 PL spectral variations under visible light irradiation at regular intervals of time on the Ti(DCMPPT)O sample in TA solution. | |
The fluorescence intensity of 2-hydroxy terephthalic acid, produced in the photoreaction in the presence of a Ti-complex catalyst under solar light irradiation, was indicated. Therefore, continuous irradiation of light for certain time periods, the intensity of the fluorescence signals improved gradually, signifying an enhanced concentration of ˙OH radicals in the photocatalytic reaction that are accountable for the enriched photocatalytic activity of the Ti-complexes. Thus, the increase in the photoactivity of the Ti-complex for oxidative cyclization and MB degradation can be attributed to the intensification of ˙OH radical generation.
Conclusion
Ti(IV) complexes of (E)-3-(((2-mercaptophenyl)imino)methyl)pyridine-2-thiol, (E)-3-(((2-mercapto-4,5-dimethyl phenyl)imino)methyl)pyridine-2-thiol and (E)-3-(((4,5-dichloro-2-mercaptophenyl)imino)methyl)pyridine-2-thiol were synthesized and characterized by numerous spectroscopy techniques. The photocatalytic activity of the prepared complexes and oxidative cyclization of MB and (E)-prop-1-en-1-ylbenzene were studied analytically under visible light irradiation. The photocatalytic activity was more efficient in the presence of the Ti(DCMPPT)O complex than the other two complexes and this complex was also more stable after three cycles. The photooxidation and degradation studies indicate that these metal complexes with greater photocatalytic efficacy will be prospective candidates for green cleansing under visible light and room temperature conditions. The prepared metal complexes in this work may find other similar applications with a more diverse range of reactants containing organic dye pollutants and for the aromatization of polycyclic hydrocarbons.
Acknowledgements
The authors would like to thank the DST-FIST schemes and UGC, New Delhi. One of us (Mahesh Subburu) thanks the University Grants Commission (UGC), New Delhi for the award of Junior Research Fellowship.
Notes and references
-
(a) R. Chinchilla and C. Nájera, Chem. Soc. Rev., 2011, 40, 5084–5121 RSC;
(b) C. P. Vinod, K. Wilson and A. F. Lee, J. Chem. Technol. Biotechnol., 2011, 86, 161–171 CrossRef CAS PubMed;
(c) Y. Ishii, S. Sakaguchi and T. Iwahama, Adv. Synth. Catal., 2001, 343, 393–427 CrossRef CAS;
(d) S. K. Das, S. P. Mahanta and K. K. Bania, RSC Adv., 2014, 4, 51496–51509 RSC.
- N. B. Gopal Reddy, P. M. Krishna and N. Kottam, Spectrochim. Acta, Part A, 2015, 137, 371–377 CrossRef CAS PubMed.
- J. J. Pignatello and G. Chapa, Environ. Toxicol. Chem., 1994, 13, 423–427 CrossRef CAS PubMed.
- F. Chen, J. He, J. Zhao and C. J. Yu, New J. Chem., 2002, 26, 336–341 RSC.
- M. R. Hoffmann, S. T. Martin, W. Choi and D. W. Bahnemann, Chem. Rev., 1995, 95, 69–96 CrossRef CAS.
-
(a) L. Kavan, M. Grtzel, S. E. Gilbert, C. Klemenz and H. J. Scheel, J. Am. Chem. Soc., 1996, 118, 6716–6721 CrossRef CAS;
(b) E. Beyers, P. Cool and E. F. Vansant, J. Phys. Chem. B, 2005, 109, 10081–10086 CrossRef CAS PubMed.
- M. A. Subramanian, G. Aravamudan and G. V. S. Rao, Prog. Solid State Chem., 1983, 15, 55–143 CrossRef CAS.
-
(a) V. Etacheri, M. K. Seery, S. J. Hinder and S. C. Pillai, Inorg. Chem., 2012, 51, 7164–7173 CrossRef CAS PubMed;
(b) M. B. Fishera, D. A. Keaneb, P. Fernández-Ibáñez, J. Colreavy, S. J. Hinder, K. G. McGuigan and S. C. Pillai, Appl. Catal., B, 2013, 130–131, 8–13 CrossRef PubMed;
(c) S. Banerjee, S. C. Pillai, P. Falaras, K. E. O’Shea, J. A. Byrne and D. D. Dionysiou, J. Phys. Chem. Lett., 2014, 5, 2543–2554 CrossRef CAS.
- K. Dutta, S. Bhattacharjee, B. Chaudhuri and S. Mukhopadhyay, J. Environ. Sci. Health, Part A: Toxic/Hazard. Subst. Environ. Eng., 2003, 38, 1311–1326 CrossRef.
- C. C. Liu, T. S. Lin, S. I. Chan and C. Y. Mou, J. Catal., 2015, 322, 139–151 CrossRef CAS PubMed.
- B. Agboola, K. Ozoemena and T. Nyokong, J. Mol. Catal. A: Chem., 2005, 227, 209–216 CrossRef CAS PubMed.
- G. R. Reddy, S. Balasubramanian and K. Chennakesavulu, J. Mater. Chem. A, 2014, 2, 15598–15610 Search PubMed.
- X. Song, H. Wen, C. Ma and C. Chen, Polyhedron, 2014, 81, 639–645 CrossRef CAS PubMed.
- D. Dey, G. Kaur, M. Patra, A. R. Choudhury, N. Kole and B. Biswas, Inorg. Chim. Acta, 2014, 421, 335–341 CrossRef CAS PubMed.
- A. Fujishima, T. N. Rao and D. A. Tryk, J. Photochem. Photobiol., C, 2000, 1, 1–21 CrossRef CAS.
- A. L. Linsebigler, G. Q. Lu and J. T. Yates, Chem. Rev., 1995, 95, 735–758 CrossRef CAS.
- D. Li, H. Haneda, S. Hishita and N. Ohashi, Chem. Mater., 2005, 17, 2588–2595 CrossRef CAS.
- R. Bacsa, J. Kiwi, T. Ohno, P. Albers and V. Nadtochenko, J. Phys. Chem. B, 2005, 109, 5994–6003 CrossRef CAS PubMed.
- A. L. Sobolewski and W. Domcke, Phys. Chem. Chem. Phys., 2012, 14, 12807–12817 RSC.
-
(a) S. Y. Ryu, M. Yoon, J. H. Choy, S. H. Hwang, A. Frube, T. Asahi and H. Masuhara, Bull. Korean Chem. Soc., 2003, 24, 446–452 CrossRef CAS;
(b) K. S. Jeon, T. S. Park, Y. D. Suh and M. Yoon, J. Photochem. Photobiol., A, 2009, 207, 20–27 CrossRef CAS PubMed;
(c) Y. H. Kim, S. D. Junga, Y. J. Jang, C. Im, S. K. Kim and D. W. Cho, J. Photochem. Photobiol., A, 2013, 270, 7–13 CrossRef CAS PubMed.
- D. Owiny, S. Parkin and F. T. Ladipo, J. Organomet. Chem., 2003, 678, 134–141 CrossRef CAS.
- S. L. Hancock, M. F. Mahon and M. D. Jones, Chem. Cent. J., 2013, 7, 135 CrossRef PubMed.
-
(a) R. Zhao, T. Liu, L. Wang and H. Ma, Dalton Trans., 2014, 12663–12677 RSC;
(b) K. K. Bania, G. V. Karunakar and L. Satyanarayana, RSC Adv., 2015, 5, 33185–33198 RSC.
- Y. Li and R. T. Yang, Langmuir, 2007, 23, 12937–12944 CrossRef CAS PubMed.
- H. Wang, K. Yao, Z. Zhang, J. Jagiello, Q. Gong, Y. Han and J. Li, Chem. Sci., 2014, 5, 620–624 RSC.
- T. G. Glover, G. W. Peterson, B. J. Schindler, D. Britt and O. Yaghi, Chem. Eng. Sci., 2011, 66, 163–170 CrossRef PubMed.
- R. J. Alamino, J. Heterocycl. Chem., 1971, 8, 309–310 CrossRef PubMed.
- N. Sharma, R. Gupta, M. Kumar and R. R. Gupta, J. Fluorine Chem., 1999, 98, 153–157 CrossRef CAS.
- H. Zhang and J. F. Banfield, J. Phys. Chem. B, 2000, 104, 3481–3487 CrossRef CAS.
- W. J. Geary, Coord. Chem. Rev., 1971, 7, 81–122 CrossRef CAS.
- S. Arockiasamy, K. Manoj, M. M. Bhadbhade, C. Mallika and K. S. Nagaraja, Inorg. Chim. Acta, 2010, 363, 2243–2249 CrossRef CAS PubMed.
- V. A. Tuskaev, S. C. Gagieva, D. A. Kurmaev, N. A. Kolosov, I. V. Fedyanin and B. M. Bulychev, Inorg. Chim. Acta, 2015, 425, 275–281 CrossRef CAS PubMed.
- N. T. Nolan, M. K. Seery, S. J. Hinder, L. F. Healy and S. C. Pillai, J. Phys. Chem. C, 2010, 114, 13026–13034 CAS.
- L. Chen, N. Zhao, Q. Wang, G. Hou, H. Song and G. Zi, Inorg. Chim. Acta, 2013, 402, 140–155 CrossRef CAS PubMed.
- D. Owiny, S. Parkin and F. T. Ladipo, J. Organomet. Chem., 2003, 678, 134–141 CrossRef CAS.
- D. M. Boghaei and S. Mohebi, Tetrahedron, 2000, 58, 5357–5366 CrossRef.
- D. L. Akins, H.-R. Zhu and C. Guo, J. Phys. Chem., 1994, 98, 3612–3618 CrossRef CAS.
- M. Yoon, J.-R. Chang and D. Kim, Bull. Korean Chem. Soc., 1988, 9, 40–44 CAS.
- K. Kaleta, M. Ruhmann, O. Theilmann, S. Roy, T. Beweries, P. Arndt, A. Villinger, E. D. Jemmis, A. Schulz and U. Rosenthal, Eur. J. Inorg. Chem., 2012, 611–617 CrossRef CAS PubMed.
- Y. M. Issa, A. L. Al-ansary, O. E. Sharif and M. M. El-ajaily, Transition Met. Chem., 1997, 22, 441–446 CrossRef CAS.
- L. Chen, N. Zhao, Q. Wang, G. Hou, H. Song and G. Zi, Inorg. Chim. Acta, 2013, 402, 140–155 CrossRef CAS PubMed.
- B. E. Langner, Selenium and Selenium Compounds, 2000 Search PubMed.
- L. M. Stephenson and D. R. Speth, J. Org. Chem., 1979, 44, 4683–4689 CrossRef CAS.
- W. D. Woggon, F. Ruther and H. Egli, J. Chem. Soc., Chem. Commun., 1980, 706–708 RSC.
- M. S. Malamas, E. S. Manas, R. E. McDevitt, I. Gunawan, Z. B. Xu, M. D. Collini, C. P. Miller, T. Dinh, R. A. Henderson, J. C. Keith and H. A. Harris, J. Med. Chem., 2004, 47, 5021–5040 CrossRef CAS PubMed.
- Y. Tagawa, H. Koba, K. Tomoike and K. Sunmoto, Heterocycles, 2011, 83, 867–874 CrossRef CAS.
- D. R. Chancellor, K. E. Davies, O. D. Moor, C. R. Dorgan, P. D. Johnson, A. G. Lambert, D. Lawrence, C. Lecci, C. Maillol, P. J. Middleton, G. Nugent, S. D. Poignant, A. C. Potter, P. D. Price, R. J. Pye, R. Storer, J. M. Tinsley, R. V. Well, R. Vickers, J. Vile, F. J. Wilkes, F. X. Wilson, S. P. Wren and G. M. Wynne, J. Med. Chem., 2011, 54, 3241–3250 CrossRef CAS PubMed.
- C. J. Smith, A. Ali, L. Chen, M. L. Hammond, M. S. Anderson, Y. Chen, S. S. Eveland, Q. Guo, S. A. Hyland, D. P. Milot, C. P. Sparrow, S. D. Wright and P. J. Sinclair, Bioorg. Med. Chem. Lett., 2010, 20, 346–349 CrossRef CAS PubMed.
- A. Dhakshinamoorthy and H. Garcia, Chem. Soc. Rev., 2014, 43, 5750–5765 RSC.
- E. D. O. Vizioli, C. M. Chin, R. F. Menegon, L. Blau, J. L. D. Santos and M. D. C. Longo, PCT Int. Appl, WO 2009/124371 A2, 15 October 2009.
- A. M. Schoevaars, W. Kruizinga, R. W. J. Zijlstra, N. Veldman, A. L. Spek and B. L. Feringa, J. Org. Chem., 1997, 62, 4943–4948 CrossRef CAS.
- B. L. Feinga, R. A. van Delden, N. Koumura and E. M. Geertsema, Chem. Rev., 2000, 100, 1789–1816 CrossRef PubMed.
- L. Wang, Z. G. Ma, X. J. Wei, Q. Y. Meng, D. T. Yang, S. F. Du, Z. F. Chen, L. Z. Wu and Q. Liu, Green Chem., 2014, 16, 3752–3757 RSC.
-
(a) M. Vithal, S. R. Krishna, G. Ravi, S. Palla, R. Velchuri and S. Pola, Ceram. Int., 2013, 39, 8429–8439 CrossRef CAS PubMed;
(b) G. Subramanian, P. Nalawade, S. J. Hinder, S. C. Pillai and H. Prakash, RSC Adv., 2015, 5, 31716–31724 RSC;
(c) A. Jinasan, T. Poonsawat, L. Chaicharoenwimolkul, S. Pornsuwan and E. Somsook, RSC Adv., 2015, 5, 31324–31328 RSC.
- L. Wang, G. Nan, X. Yang, Q. Peng, Q. Li and Z. Shuai, Chem. Soc. Rev., 2010, 39, 423–434 RSC.
-
(a) C. C. Wang, J. R. Li, X. L. Lv, Y. Q. Zhang and G. Guo, Energy Environ. Sci., 2014, 7, 2831–2867 RSC;
(b) Y. Cui, S. M. Goldup and S. Dunn, RSC Adv., 2015, 5, 30372–30379 RSC.
-
(a) S. E. Page, W. A. Arnold and K. McNeill, J. Environ. Monit., 2010, 12, 1658–1665 RSC;
(b) X. Qu, L. J. Kirschenbaum and E. T. Borish, Photochem. Photobiol., 2000, 71, 307–313 CrossRef CAS;
(c) J. Yu, W. Wang, B. Cheng and B. L. Su, J. Phys. Chem. C, 2009, 113, 6743–6750 CrossRef CAS;
(d) Q. Xiang, J. Yu and M. Jaroniec, Phys. Chem. Chem. Phys., 2011, 13, 4853–4861 RSC;
(e) G. K. Pradhan, N. Sahu and K. M. Parida, RSC Adv., 2013, 3, 7912–7920 RSC.
Footnote |
† Electronic supplementary information (ESI) available: All of the experimental processes for oxidative cyclization, photodegradation of methylene blue and mechanistic studies of hydroxyl radicals with terephthalic acid. Some of the spectral data are also included. See DOI: 10.1039/c5ra07616a |
|
This journal is © The Royal Society of Chemistry 2015 |