DOI:
10.1039/C5RA06451A
(Paper)
RSC Adv., 2015,
5, 37789-37799
Polymer organogelation with chitin and chitin nanocrystals†
Received
25th March 2015
, Accepted 16th April 2015
First published on 16th April 2015
Abstract
In this paper, we show that biodegradable and biocompatible organogels can be formed with chitin as the filler material and triglycerides as the continuous hydrophobic phase. When crude chitin was used, a large degree of aggregation was observed that prevented the formation of stable organogels. Two approaches were used to diminish this degree of aggregation and increase the stability. Either surfactants were used to increase the dispersability of the crude chitin, or the crude chitin was transformed into smaller rod-like nanocrystals by acid hydrolysis. Both approaches led to the formation of stable organogels with storage moduli up to 106 Pa for high chitin concentrations (20 wt%). Three different types of surfactants were used, namely phosphatidylcholine, enzymatically modified phosphatidylcholine and sorbitan monostearate (Span 60). The choice of surfactant has a large influence on the gel strength and the temperature sensitivity of the gels. With chitin nanocrystals, in the presence of surfactants, larger gel strengths were observed for lower concentrations (1–10 wt%), indicating more efficient packing of the particles. Gels were stable even after addition of considerable amounts of water up to 25 wt%. The increase in gel strength in the presence of water (storage modulus) was most likely an effect of the water absorption ability of chitin that increased the effective volume fraction of the fillers.
1. Introduction
Polymer hydrogelation is a topic that has been thoroughly studied.1 A polymer hydrogel can be classified as a semi-solid material consisting of a solid polymer network holding large amounts of water in the interstitial areas. The polymer network imparts the visco-elastic behavior of the system, which provides resistance against large deformation. According to the type of the three dimensional network, these structures can be divided in two main classes; covalently cross-linked materials, and those that are formed through physical interactions and entangled networks.2,3 Although hydrogels are very common and their behaviour is well understood, their counterpart, gels containing organic liquids (organogels or oleogels in the case of oil) has always been of less interest. Despite not extensively studied, organogelation is a topic with rapidly growing interest and is of high importance.4,5 The term organogel covers the gelation of organic liquids, using different types of network formation. Similar to hydrogels, different network categories can be classified. Most organogels known in literature are created via self-assembly of low molecular weight compounds into fibrous networks.6 A lot of different compounds are known to result in network formation (supramolecular gels) but a complete understanding of the relationship between molecular architecture and organogel properties is still lacking.6,7 Examples of organogelators include porphyrins,8 oligopeptides,9 amino acids,10 ureas,11 fatty alcohols,12 lecithins13,14 and phytosterols.15 The physical nature of the intermolecular interactions results in gels with special characteristics, like thermo reversibility and chemical sensitivity; therefore they could have structural similarities with hydrogels.16 These characteristics, along with the diversity of nanostructures that can be created with these compounds, makes molecular organogels excellent candidates for numerous potential applications from drug-delivery,17 catalysis,18 biomimetics,19 oil spills10 to foods.20
Supramolecular assembly is the main mechanism of structuring organic liquids, but they can also be structured by macromolecules (polymeric gels), which is the second organogel category.21 These organogels are comprised of polymers that interact through physical forces, such as hydrogen bonding, van der Waals and electrostatic interactions.22 There are only a few studies focussing on the behaviour of polymer organogels. These studies reveal that the quasi-solid nature is mainly defined by the polymer–polymer and polymer–solvent interactions, which exist due to hydrogen bonding and electrostatic interactions.21 Macromolecules most exhaustively studied are cellulosic derivatives, with ethylcellulose (EC) being the one with the highest functionality.21,23 EC has been used as a gelator for various organic solvents, such as monoesters, diesters and triglycerides.21,24 Sanchez and co-workers showed that EC can be used to create organogels and that by changing the molecular weight of the polymer, the mechanical properties of the organogel could be altered.24 In different types of organic solvents, like monoester and diester phthalates, due to a competition between polymer–polymer interactions and stronger polymer–solvent interactions, the solvent acted as a bridge between EC chains.25 Stronger phthalate–EC interactions occurred due to electrostatic forces between the carbonyls (C
O) of the solvent and the hydroxyl groups (OH) of the polymer, while intermolecular ethylcellulose interactions occurred due to hydrogen bonding.25 The properties of the final EC organogel are to a great extent determined by the balance between the polymer–polymer hydrogen bonding and solvent–polymer electrostatic interactions. Some examples of different non-aqueous solvents used are phthalates,25 ethanol,26 vegetable oils,21,24 and propylene glycol diester of caprylic acid and capric fatty acids.27
Although EC is a usable organogelator, very few (other) biopolymers have been identified to provide similar behavior. In this study, we show that the biopolymer chitin also contains organogelating ability. Due to its biocompatible and biodegradable nature, chitin has potential to be used in a wide range of bio-related applications.28–35 Chitin organogels with common edible lipid material (oils), could also be used as an alternative for solid fat in many food-products or food-related applications.
Next to cellulose, chitin is the second most abundant organic material in nature, existing in exoskeletons of crustaceans (such as crabs and shrimps),36,37 and each year, roughly 1011 tons of chitin is produced, of which a large part is considered as waste material.38
Fig. 1a illustrates the chemical structure of chitin, which is comprised of repeating units of 2-acetamido-2-deoxy-D-glucopyranose (Fig. 1b). Chitin is actually similar to ethylcellulose, with the only difference being that chitin contains an acetamido group in the C-2 position instead of a hydroxyl or ethyl ester groups in the case of ethylcellulose. Chitin is a highly crystalline material with strong hydrogen bonding, and is very difficult to be dissolved in aqueous systems. Along with its deacetylated form (chitosan), chitin recently attracts an increasing amount of attention as the inherent biological and physicochemical characteristics are better understood.39
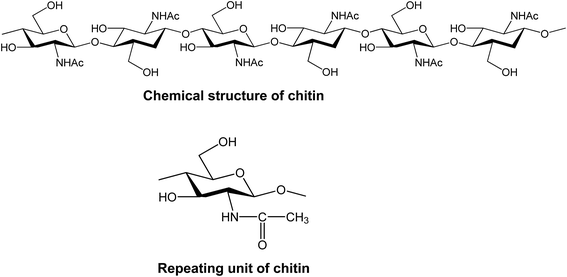 |
| Fig. 1 Molecular structure of chitin and its repeating unit. | |
Chitin and its derivatives have already been proposed as thickeners of vegetable oil for bio-lubricant applications, but chitin solely, has not been shown to provide a strong and stable network.40 In this work we studied mixtures of chitin with different types of surfactants for gelation of triacylglycerides. We used commercial available crude chitin and chitin nanocrystals that were formed after hydrolysis of chitin as potential structural elements for network formation.
2. Experimental section
Materials
Practical grade chitin from shrimp shells (Lot#051M7013V) was obtained from Sigma-Aldrich (Steinheim, Germany). Before any use, it was dried overnight at 50 °C. Refined sunflower oil was purchased from commercial sources. Sunflower oil (triacylglyceride solvent) was stored over sodium sulphate (Na2SO4) to minimize the presence of moisture. Oil-free soy lecithin (SOLEC™, FP30, >30% phosphatidylcholine) was kindly provided by Solae (Le Grand-Saconnex, Switzerland). Only the ethanol soluble fraction was used, which contained around 70 wt% of phosphatidylcholine (PC). Phospholipase D from Streptomyces cromofuscus, Span 60, sodium hypochlorite solution (NaOCl) and acetic acid (≥99.0%) were purchased from Sigma-Aldrich (Steinheim, Germany). Potassium hydroxide pellets (KOH) and hydrochloric acid (HCl, 38%) were obtained from Merck KGaA (Darmstadt, Germany).
Formation of chitin nanocrystals (ChN)
Chitin nanocrystals (ChN) were produced based on a protocol described by Tzoumaki et al.41 In the original protocol, the crude chitin was first purified before use. However, EDX analysis (data not shown) showed that the provided crude chitin already had a purity of >98%, and therefore the purification steps were omitted. Chitin (40 g) was mixed and bleached with 700 mL NaOCl solution (17 g NaOCl in 800 mL distilled water, adjusted to pH 4 with acetic acid) for 2 hours at 80 °C. The sample was heated on a heating plate under continuous stirring. After 2 hours, the dispersion was cooled down to room temperature and filtered using a glass fibre pre-filter and a membrane filter with a pore size of 0.45 μm (OE67; Whatman) attached to a vacuum pump. The retentate was dispersed in 700 mL 5% w/w KOH solution and stirred for 36 hours at room temperature, to remove residual proteins. Afterwards, the dispersion was centrifuged for 15 minutes at 3400 rpm (3500 g). The resulting pellet was collected and redispersed in 1 L HCl (3 N). This dispersion was boiled for 90 min to hydrolyse the chitin. Subsequently, the dispersion was diluted (1
:
2) with distilled water and centrifuged at 4900 rpm (5000 g) for 15 minutes. The pellet was collected and redispersed in distilled water and transferred into a dialysis membrane. Dialysis was performed for a total of 12 hours with distilled water which was changed after 3 hours for 4 times. After dialysis, the pH of the dispersion was adjusted to 3 with 1 N HCl. The chitin nanocrystal dispersion was then mixed with lecithin in a chitin nanocrystal
:
lecithin ratio of 2
:
1. Finally, the mixture was frizzed at −32 °C and eventually freeze-dried at −60 °C, at 0.011 mbar for 5 days to obtain dry chitin powder.
Lecithin modification with phospholipase D
High phosphatidylcholine (PC) content (70 wt%) lecithin was mixed with demi water in a ratio of 5
:
1 and 15 μL of phospholipase D was added to enzymatically modify PC to phosphatidic acid. The mixture was initially stirred with a spatula until being homogeneous and kept overnight at room temperature. After the end of the reaction, the mixture was finally frizzed at −32 °C and freeze-dried. at −60 °C, at 0.011 mbar for 5 days to obtain dry chitin powder.
Chitin size analysis
The particle size (d3.2) of both crude chitin and ChN in sunflower oil were determined by dynamic light scattering (DLS ZetasizerNanoZS, Malvern Instruments Ltd, UK). Dispersions of crude chitin and ChN in sunflower oil (0.01 wt%) were prepared by mixing with a high speed blender (IKA(R) Ultra-Turrax T25, IKA Works, Inc. Malaysia) at 17
500 rpm for 60 s. All measurements were performed in a disposable capillary cell at room temperature and each sample was measured in triplicate to obtain an average value.
Organogel preparation
Mixing of specific amounts of freeze-dried ChN/phosphatidylcholine or chitin–surfactants with water and oil (triglyceride solvent) was initially performed with a high speed blender (IKA(R) Ultra-Turrax T25, IKA Works, Inc. Malaysia) at 17
500 rpm for 90 s. Afterwards, the dispersions were heated on a stirring plate for 30 minutes at 85 °C. The organogels were then cooled down at room temperature and stored in the fridge at 4 °C. Organogels with a chitin concentration of 2, 3, 5, 8, 10 or 20 wt% in the form of crude chitin or ChN were made. Crude chitin samples were mixed with 5, 10 or 15 wt% of phosphatidylcholine (PC), enzymatically modified phosphatidylcholine or Span 60 and ChN were always present with 2
:
1 ratio with phosphatidylcholine (PC). Finally, 5 to 25 wt% of water was added to the organogels containing 10 wt% ChN and 5 wt% PC or to 10 wt% crude chitin and 5 wt% PC.
Characterization of the gel structure
Cryo-scanning electron microscopy (cryo-SEM).
Organogels containing either 20 wt% of chitin and 10 wt% PC or ChN with 10% PC (ratio of 2
:
1) were prepared. Small pieces of the gels were glued on a brass sample holder with carbon glue (Leit-C, Neubauer Chemicalien, Germany), and subsequently frozen with liquid nitrogen. All manipulations were carried out under liquid nitrogen level. The sample holder was fitted in the transfer cryogenic Leica holder. The Leica sample holder was transferred to a non-dedicated cryo-preparation system (MED 020/VCT 100, Leica, Vienna, Austria) onto a sample stage at −93 °C. In this cryo-preparation chamber, the samples were immediately freeze-dried for 23 minutes at −93 °C at 1,3 × 10−6 mBar to remove contaminating water vapor. The sample was then sputter coated with a layer of 4 nm Tungsten at the same temperature. The samples were transferred cryo-shielded into the field emission scanning microscope (Magellan 400, FEI, Eindhoven, the Netherlands) onto the sample stage at −122 °C at 4 × 10−7 mBar. The analysis was performed with SE at 2 kV, 13 pA. All images were recorded digitally.
Confocal laser scanning microscopy (CLSM).
CLSM images were obtained at room temperature on a LEICA TCS SP5 Confocal Laser Scanning Microscope (Leica Microsystems GmbH., Mannheim, Germany) equipped with an inverted microscope (model Leica DMI6000), containing a set of four visible light lasers. The used objectives were HC PL APO 10×/0.40 CS and HC PL APO 20 × /0.70 IMM/CORR CS. Digital image files were acquired in 1024 × 1024 pixel resolution. Samples were carefully placed on a microscope slide and stained with Nile Blue.
Transmission electron microscopy.
TEM analysis was performed on aqueous suspensions of ChN (0.1 wt%) with a JEOL JEM-2000FX transmission microscope operated at an acceleration voltage of 80 kV. One drop of the ChN suspension was deposited on a carbon-coated copper grid and allowed to dry upon air.
Rheological measurements.
Rheological characterisation of organogels was performed with a stress-controlled rotational Physica MCR 300 rheometer (Physica Messtechnic GmbH, Stuttgart, Germany) with a PP50-TEKP CF56 setup, using a 50 mm parallel plate configuration with 1.0 mm gap width. Temperature was regulated by a Paar Physica circulating water bath and a Peltier system (TEZ 150P/MCR) with an accuracy of ±0.1 °C. The linear viscoelastic region was assessed by amplitude sweep experiments at a constant frequency of 1 Hz. For all organogels a constant deformation of γ = 0.01 was used, which was well within the linear viscoelastic region for all samples. Small deformation oscillatory measurements were performed over a frequency range of 0.01–100 Hz at 20 °C to obtain the storage and loss moduli as a representative for the visco-elastic properties. To explore the behaviour of the oil body emulsions upon heating, the samples were kept at 5 °C for 4 hours and then heated from 5 to 90 °C at a scan rate of 3 °C min−1. After remaining for 20 min at 90 °C they were cooled back to 5 °C at the same scan rate.
3. Discussion
Chitin and ChN
Commercially available chitin is a white powder that is comprised of the polysaccharide in an aggregated state. The structure of the dry powder is visualized by cryo-SEM images as illustrated in Fig. 2a. Here, it can be clearly seen that the chitin aggregates have an average diameter of roughly 100 ± 50 μm. As we use chitin as a structural element for organogelation, we also determined their aggregated size with dynamic light scattering. When present in sunflower oil in the dilute regime (0.01 wt%), the average size was found to be around 70 μm, similar to the size found with cryo-SEM. This shows that both in dry state and hydrophobic environments, crude chitin is aggregated to a large extent. The degree of aggregation plays an important role in the network formation and subsequent behaviour of chitin organogels, since the number of contact points (junction zones) between the structural elements determines the efficiency of the network formation and the final gel characteristics. Large aggregation minimizes the amount of contact points and should therefore be avoided. At larger magnification (Fig. 2b), it can be seen that the aggregates are comprised of fibres that are around 10 nm in width and several hundred nanometres in length. To decrease the chitin aggregation and investigate the effect of size and shape of the chitin structural elements on the network formation and organogelation ability, we have also used their hydrolyzed product as a potential oil gelator. Acid hydrolysis of purified chitin leads to the formation of smaller ChN which a length ranging from 200 to 500 nm and their transverse dimensions are about 10 to 15 nm.42,43 The formed ChN in an aqueous suspension are illustrated in Fig. 2c, which shows that aggregation is minimized. This size is significantly smaller than the aggregate size of the crude chitin. Due to the change in size and shape, a different network is formed. According to literature, when chitin nanocrystals are dispersed in water, a nematic ordering occurs under specific conditions and at specific concentrations of the dispersions, inducing network and gel formation.41 Roughly 3.6 wt% of ChN is required to form a hydrogel.
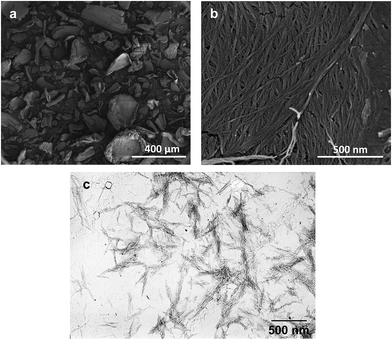 |
| Fig. 2 Cryo-SEM (a and b) and TEM (c) images of crude chitin powder (a and b) and chitin nanocrystals dispersed in water (c). | |
Organogels formed with crude chitin, crude chitin/PC and chitin nanocrystals/PC
Addition of crude chitin to sunflower oil resulted initially in a very viscous dispersion, which appeared to be an organogel. However, these organogels were very unstable, since the chitin aggregates started to precipitate a couple of days later, which is visualized in Fig. 3a. This behavior is similar to the results found by Sánchez et al.,40 who, amongst others, studied the potential of chitin to form chitin-based organogels in soybean and castor oil. Despite the fact that they did not specifically study the physical stability after storage, they observed a phenomenon called “oil bleeding”, indicating that crude chitin is not able to form a stable network that can hold substantial amounts of oil.
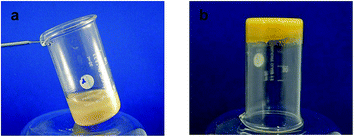 |
| Fig. 3 Digital images of systems with crude chitin (a) and chitin/PC (2 : 1) (b) in purified sunflower oil (chitin concentration was 20 wt%). | |
The sedimentation of crude chitin in sunflower oil could be attributed to the fact that the chitin is extensively aggregated, which induces fast sedimentation and prevents network formation. Although there might be weak inter-particle interactions, like dipole–dipole and hydrogen bonding, these were not strong enough to create a stable system. To decrease aggregate formation, we have added different surfactants to improve the stability of the dispersions. As the surfactants have an amphiphilic nature, they can interact with hydrophilic sites on the surface of the chitin, while leaving their hydrophobic sites in the continuous oil phase. In this way, the dispersability of the chitin and the stability of the chitin dispersion could be improved since hydrophilic chitin–chitin interactions leading to extensive aggregation can be minimized. Additionally, the surfactants also act as a plasticizer. The general function of plasticizers is to reduce friction between polymers and reduce rigidity in polymers, thereby altering the mechanical properties of the system.44 Minimization of inter-polymer interactions through the addition of a compatible surfactant plasticizer could also lead to the delay of the gelation process, which can be a desirable effect.45 The effect of the addition of such surface active agents as plasticizers has been thoroughly investigated in the case of dispersions of ethylcellulose in vegetable oils.45 A number of different non-ionic hydrophobic surfactants were added to the ethylcellulose–oil mixture such as Span 60, Span 80, Glyceryl monooleate (GMO), Glyceryl monostearate (GMS) and Polyglyceryl ester of lauric acid – polyglyceryl laurate (PGPL). The results showed that the addition of the surfactants can tailor the organogels for any desired applications. In this research, the dispersibility of crude chitin in sunflower oil was enhanced by the addition of either a non-ionic surfactant (Span 60), a zwitter-ionic surfactant (phosphatidylcholine, PC)46 or an anionic, enzymatically modified PC. The enzyme (phospholipase D) catalyzes the hydrolysis of PC to form a phosphatidic acid and a choline group (Fig. 4).47,48 As can be seen in Fig. 4, unmodified PC is electrically neutral at a pH of 7, but its enzymatically modified derivative is negatively charged due to the phosphate group. Besides the difference in charge, due to the removal of the hydrophilic choline group, the hydrophobicity of the modified derivative is expected to be higher than the hydrophobicity of unmodified PC. It is therefore hypothesized that modified PC is more flexible to interact through hydrophilic forces with chitin and at the same time has probably more affinity to interact through hydrophobic forces with the solvent and hence, be a more effective plasticizer.
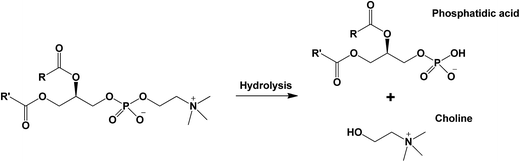 |
| Fig. 4 Schematic overview of the enzymatic hydrolysis of phosphatidylcholine (PC) by phospholipase D. | |
As expected, addition of different surfactants to crude chitin dispersions increased the organogel stability. Fig. 3b shows an example of the dispersion with crude chitin and PC (2
:
1). As can be seen, a stable quasi-solid material was formed upon cooling. This indicates that with the use of surfactants, crude chitin is a good candidate for organogelation.
The differences between organogels made with crude chitin and PC or ChN and PC were first studied by a cryo-Scanning Electron Microscope (Fig. 5) to gain insight in their microstructure. Fig. 5a–c illustrate organogels with crude chitin and PC (2
:
1), while Fig. 5d–f, illustrate organogels with ChN and PC (2
:
1). The appearance of all gels is found to be very different from the images of the crude chitin powder (Fig. 2a and b). All images in Fig. 5 show that these large chitin particles of the dry chitin powder are now absent, which confirms the hypothesis that surfactants would aid the dispersibility of the polysaccharide. Apart from the apparent differences of the dry chitin powder with the dispersed chitin, there are also significant differences between the two types of organogels. At first sight, the main difference is the apparent roughness of the surface of the gel containing crude chitin and PC (Fig. 5a–c) compared to the smoothness of the surface of the gels with ChN and PC (Fig. 5d–f). Within all the images, there are features that can be assigned to the strands, which is due to network formation of the chitin. However, the strands in gels with crude chitin seem to be less organized than the strands in gels with ChN. When PC and chitin are combined in a hydrophobic solvent (triglycerides), they initially interact through hydrophilic interactions. It is likely that strong hydrogen bonds are further formed or weaker dipole–dipole interactions occur to strengthen the interactions. Due to these interactions, PC molecules are probable anchored on the chitin strands and their fatty acid chains create a lipophilic surface that penetrates into the more hydrophobic solvent. Similar results were also obtained when ethylcellulose was combined with small molecular weight amphiphilic compounds.45 The lipophilic coverage yields to higher flexibility of the polysaccharide molecules and subsequently their ordered arrangement and eventually solidification of the system.49–51 The length of the strands probably plays an important role in defining their flexibility and their ability to form ordered morphologies. The effect of the length was further investigated by creating chitin nanocrystals (ChN). By hydrolyzing crude chitin to nanocrystals, the length of the strands is decreased and the nanocrystals behave more as stiff particles instead of flexible strands. After creating the ChN, the dispersion was freeze dried before the ChN powder was redispersed in oil. To prevent aggregation of ChN during the drying step, the aqueous dispersion was first mixed with PC in a 2
:
1 nanocrystal to surfactant ratio (ChN/PC). The systems containing ChN therefore always contain PC at the same concentrations, and the type and the amount of surfactant was not varied. Addition of this compound in the hydrophobic triglycerides solvent resulted in the formation of homogeneous and very stable semi-solid material. These organogels showed less aggregation and as can be observed in the images of Fig. 5, they had a smoother and more homogeneous surface than those containing the crude chitin and PC with the same composition. To visualize the network within these ChN organogels and compare these to the organogels of the crude chitin, confocal laser scanning microscope (CLSM) was used. Fig. 6 shows the images of these organogels, for which Fig. 6a represents the organogels with crude chitin and PC and Fig. 6b the organogels with ChN and PC. From the results of the confocal microscopy it can be concluded that the crude chitin strands interact to form more ordered morphologies that are bridged with long chains. Subsequently, these ordered and connected chitin strands interact to form a coarser network with large voids in which oil is entrapped (Fig. 6a). On the other hand, in the case of the chitin nanocrystals (ChN/PC) (Fig. 6b), the chitin is dispersed more homogeneously compared to crude chitin, but there are also regions where extensive aggregation can be seen. These aggregates are probably assemblies of the nanocrystals that also occur when ChN are in an aqueous environment.41
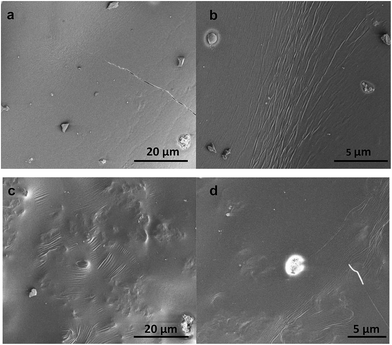 |
| Fig. 5 Cryo-SEM images of purified sunflower oil organogel surface with chitin/PC (Ch/PC, 2 : 1) (a–c) and chitin nanocrystals/PC (2 : 1) (d–f) (chitin or ChN concentration was 20 wt%). | |
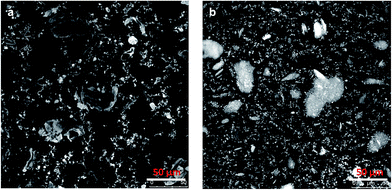 |
| Fig. 6 CLSM images of purified sunflower oil organogels with chitin/PC (2 : 1) (a) and chitin nanocrystals/PC (2 : 1) (b) (chitin or ChN concentration was 20 wt%). | |
Properties of the chitin organogels
Rheological characteristics.
As discussed in the previous section, the properties of the organogel can be changed by altering the length and the flexibility of the chitin strands, in combination with the interactions between the chitin strands. In our case, the interactions between the chitin strands and the surfactants were partly changed by heating the sample to 85 °C. Although this temperature is insufficient to induce a glass to rubber transition, it does change the structure of chitin to such extent that certain interactions are altered. According to literature,51,52 at temperatures above 70 °C, some local changes occur in chitin strands that in our case led to less polymer–polymer and more polymer–solvent or polymer–surfactant interactions.
The interactions between the chitin strands were also altered by using three different types of surfactants (PC, modified PC and Span 60) and were added at 3 different concentrations, while the added amount of chitin was fixed at 20 wt%.
Fig. 7 shows that the systems with 20 wt% crude chitin and 10 wt% PC exhibited a gel-like behaviour as the values of G′ exceeded those of G′′ at the frequency range explored, while both moduli exhibited a weak frequency dependence. The rheological data (storage modulus G′) of gels with different type and amounts of surfactants are shown in Fig. 8. More detailed information on strain sweeps can be found as ESI.† As it can be observed, the concentration of the added surfactant plays an important role. When a low amount (5%) of surfactant was added (in a 4
:
1 chitin
:
surfactant ratio), stable organogels were formed, but they behaved more like viscous liquids (tan
δ ∼ 1). As can be seen, increasing surfactant concentration enhanced the gel strength of the gels. Apparently, the presence of the surfactants limited chitin aggregation to such extent that they were more able to participate in the formation of a stable network. Also the nature of the surfactant has an effect on the gel strength of the systems. Span 60 leads to gels with the larger gel strength (G′), while addition of PC leads to gels with the lowest gel strength. This may be explained by the hydrophobicity of the surfactant and the subsequent interactions with the hydrophobic environment. Surfactants are anchored through their hydrophilic head onto the chitin53,54 and their hydrophobicity determines the interaction of the polymer–surfactant complex with the triglycerides of the solvent. Modified PC47 and Span 60 (ref. 55) are more hydrophobic than PC, which apparently led to a better dispersibility in the hydrophobic medium, and therefore higher gel strength. Additionally, the charge density on the modified lecithin could also have an effect. Modified lecithin has a negative charge due to the phosphate group. As chitin is known to have a positive charge at a wide pH range,56 the electrostatic interactions may have led to a stronger attraction between the chitin and the surfactant. This may partly explain the difference with the unmodified PC, in which the lack of the negative group does not provide this high electrostatic attraction. Higher surfactant concentrations led to even higher G′ values, which is an effect of the better coverage of chitin with surfactant molecules and thus higher overall hydrophobicity of the chitin–surfactant complex and better dispersibility. These results show that the gel strength can be modified by the concentration of the components and the type of surfactants used. Free surfactants may also be responsible for the gel formation due to formation of larger structures such as micelles. However, according to our findings, at these concentrations the used surfactants are not able to form a network.
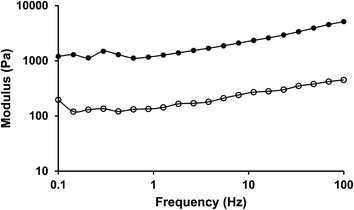 |
| Fig. 7 Mechanical spectra (20 °C, γ = 0.01) of purified sunflower oil organogels with chitin/PC (2 : 1) (chitin concentration was 20 wt%) (G′ •, G′′ ○). | |
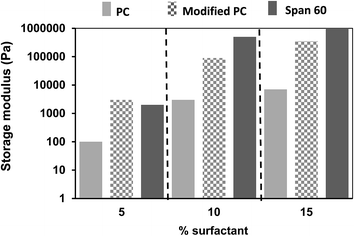 |
| Fig. 8 Storage moduli (20 °C, γ = 0.01) of 20 wt% chitin in purified sunflower oil organogels containing different amounts and different types of surfactants. | |
Temperature sensitivity.
In order to use organogels for different types of applications, their response to temperature (or melting profile) is of interest. Therefore, gel strength (G′) as a function of temperature in a range of 10 to 90 °C was examined. As can be seen in Fig. 9, the formed organogels have a different thermal response. Organogels made with crude chitin and PC or Span 60 were temperature sensitive, in contrast to organogels with modified PC where no changes occurred up to 100 °C. These data point out that the type of surfactant plays an important role in the “melting behaviour” of the gels. As it can be seen, the strength of the gels decreases with increasing temperature, probably due to rearrangements within the samples. Upon an increase in temperature, the mobility of the hydrophobic triacylglycerides increases and changes the interaction between the different components within the system. Increased temperature may enhance the mobility of the surfactant-covered chitin within the system, and therefore an enhanced lubrication between the particles may decrease the gel strength. It may also increase the solubility of the surfactants in the oil and thereby less coverage of the chitin strands due to desorption of the surfactants from the chitin surface. A decrease in gel strength is observed for both PC and Span 60 during heat treatment (Fig. 9). During the subsequent cooling step, the mobility was lowered and intermolecular bonds reformed, but were less strong than in the initial organogel. As a result, a lower storage modulus was found. Apparently, the components in the system did not have time to restructure into a similar network. The fact that the samples with modified PC show less temperature dependency, indicates that these samples are more robust. As mentioned previously, modified PC might be a more flexible molecule with a smaller hydrophilic head, and it might interact stronger with the chitin molecules due to enhanced electrostatic interactions. The enhanced interactions between the surfactant and the chitin might prevent desorption of the surfactants from the chitin, thereby making the samples less sensitive to temperature. In this way, the increased mobility of the surrounding triacylglycerides does not affect the rheological behavior of the system to a large extent.
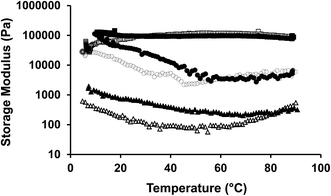 |
| Fig. 9 Temperature sweeps of 20 wt% chitin organogels with PC (heating ▲, cooling Δ) modified PC (heating ■, cooling □) and Span 60 (heating ●, cooling ○) at 2 : 1 ratio in purified sunflower oil. | |
Stability after addition of water.
It would be beneficial to create organogel networks that immobilizes the liquid solvent and is stable in polar environments.58 Water migration and low stability of organogels in aqueous environments is up to now a problem with no efficient or practical solution.20,57 Different strategies have been applied to limit solvent migration,57 but until now very few organogels are available that can resist aqueous environments. As the network formation of oleogelators is often a result of hydrophilic interactions, addition of water often leads to collapse of the network. We have investigated the stability of crude chitin/PC (2
:
1) organogels by addition of different amounts of water. For gels with a high concentration of chitin (20 wt%), the gels become very stiff and difficult to handle, so only the results of a lower chitin concentration of 10 wt% will be discussed. Upon addition of water, the organogels become harder but they were stable (by visual observation), even upon addition of 25 wt% of water. As shown in Fig. 10, the more water added, the stronger the organogels becomes. At water concentrations of 30 wt% or above, systems showed lower stability and phase separation occurred. An interesting observation was that at high water concentrations (>30 wt%) only water leaked out, while the triacylglycerides were still entrapped in the chitin/PC network. These results indicate that a certain amount of water can be incorporated within the network without losing its stability. However, it remained unclear where the water was located in the system. It is possible that water acts as a plasticizer, weakens the polymer–polymer interactions and yields to higher dispersibility.
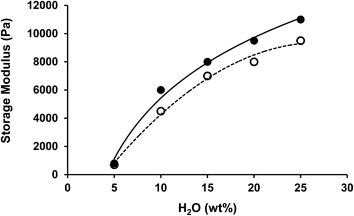 |
| Fig. 10 Dependence of storage modulus on water concentration of chitin/PC (2 : 1) (●) and chitin nanocrystals/PC (2 : 1) (○) organogels in sunflower oil (chitin or ChN concentration was 10 wt%). | |
As ChN have been shown to be capable to form stable oil-in-water emulsions,59 our initial thought was that a water-in-oil emulsion may had been formed. Therefore, we performed CLSM on different water-containing systems to get an insight in the distribution of the water. The results are given in Fig. 11, for systems containing 5 wt% and 15 wt% of water. As is illustrated in Fig. 11, the water, that appears with green color, seems to be mainly located within the chitin, where somewhat non-spherical areas can be observed. The water does not seem to be present as separate spherical water droplets stabilized by chitin at the oil water interface. The excess of hydrophilic water seems to be forced within the chitin and for both the crude chitin and the nanocrystals (Fig. 11a and c), this absorption of water is observed. Upon such water absorption, swelling of the chitin clusters would occur. Swelling of the chitin gelators would increase the effective volume fraction, and therefore a stronger network can be formed, as observed in the enhanced gel strength (Fig. 10).
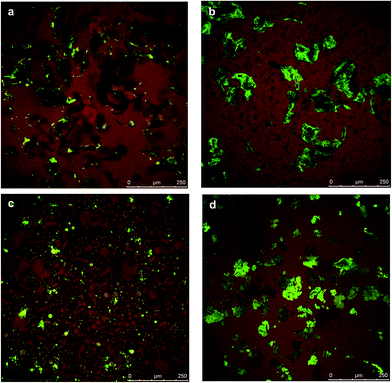 |
| Fig. 11 CLSM images of purified sunflower oil organogels with chitin/PC (2 : 1) and 5 wt% of water (a) chitin/PC (2 : 1) and 15 wt% of water (b) chitin nanocrystals/PC (2 : 1) and 5 wt% of water (c) and chitin nanocrystals/PC (2 : 1) and 15 wt% of water (d) (chitin or ChN concentration was 20 wt%). | |
Besides the effect of water absorption of the chitin, the water could also be located near the hydrophilic regions of the surfactants. For most amphiphilic molecules, when they are present in organic solvents, the presence of water could lead to structural organization into lamellar, cylindrical or cubic phases, that can lead to a network formation and subsequently to solid-like materials.60 Such network formations have been also observed for lecithin. According to literature,61 when lecithin is combined with water in organic solvents, liquid crystals that influence the overall viscosity are formed. The phase behavior is mainly determined by the concentration of lecithin and the lecithin to water molar ratio. In some cases,61 above 25 wt% of lecithin is required (at a ratio with water above 0.5) to form homogeneous organogels.62 Below this concentration and molar ratio a diluted solution of inverse spherical micelles is formed.63 In the case of the chitin–surfactant organogels, the surfactant concentration in the oil phase never exceeded the 20 wt%. According to literature,61 at this relatively low concentrations, the formed inverse spherical micelles cannot overlap to form a three-dimensional network, so, we assume that the presence of surfactant had probably minor influence on the gel strength of chitin organogels. Furthermore, since in the current research not lecithin, but purified phospatidylcholine was used, we experimentally tested the influence of this particular lecithin fraction. Indeed, according to our findings more than 25 wt% of PC is required to form a gel-like structure.14 Additionally, at PC to water ratios above 2, PC molecules precipitated and severe phase separation occurred. These experimental results exclude the possibility of the formation of an extended network due to PC and hence a significant influence on the rheological behavior of the organogels.
Addition of greater amounts of water (15 wt%, Fig. 11b and d) led to even more swelling of the chitin assemblies and the formation of a more jammed system. Besides the increased volume fraction, due to the addition of water, polymers can interact with each other through hydrogen bonding. Thereby, the added water molecules enhance the polymer–polymer interactions of the network elements which may enhance the strength of the network. As it was found, the polymer–surfactant complex could hold only a limited amount of water, the water is expelled from the chitin and results in phase separation of the system. The maximum amount of water that could be absorbed was found to be similar for both the chitin/PC and the ChN/PC systems and was found to be 25 wt%, indicating an absorption capacity of roughly 2.5.
Behavior at low gelator concentrations.
To gain understanding on the network formation, we measured the storage modulus as a function of the gelator concentration, as depicted in Fig. 12. The lowest amount of chitin used was 2.0 wt%, at a 2
:
1 ratio with PC, as this was the concentration needed to create a semi-solid system (tan
δ < 1). The results show that the storage modulus increases with the chitin concentration in a non-linear fashion. The storage modulus is exponentially dependent on the concentration, as G′ ~ eαφ.64,65 This behavior indicates that the polymer–polymer interactions are weak at this concentration range and that rearrangements most likely take place. As is shown in the same graph, the behavior depends on the state of the chitin. ChN organogels always have a higher storage modulus than for the same concentration of crude chitin. At lower chitin concentrations, the increase is roughly one order of magnitude. This is likely to be a result of their size and rod-like shape. As they are much smaller and less flexible, they are apparently more efficient to form a network.
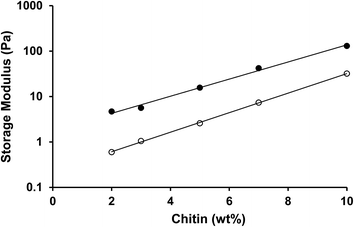 |
| Fig. 12 Dependence of storage modulus of purified sunflower oil organogels on chitin concentration (Ch/PC 2 : 1 ○ and ChN/PC 2 : 1 ●). | |
4. Conclusions
The aim of this study was to investigate the potential of chitin to form polymeric organogels in a solvent comprised of triglycerides. When crude chitin was dispersed in sunflower oil, the structural elements were not able to form a stable network due to extensive aggregation. This aggregation was diminished by the addition of surfactants, such as Span 60, PC and modified PC. This led to the formation of stable organogels, for which the organogels were characterized by a storage modulus between 102 and 106 Pa. The temperature sensitivity depends on the type of surfactant used. Organogels with PC and Span 60 showed structural changes during heating, while the ones with modified PC did not exhibit any change in G′ up to 90 °C.
Treating the chitin with an acid hydrolysis step, changed the long and flexible chitin strands into more rigid and shorter ChN of roughly 200–500 nm. Using ChN led to smoother gels and an increase in the storage modulus of 1 order of magnitude compared to crude chitin. The organogels were also investigated for their solvent migration and stability in aqueous environments. Even upon addition of large amounts of water (up to 25 wt%), the organogels were stable, and even increased 10 times in gel strength compared to 5 wt% water. The results showed that water is most likely absorbed by the chitin, and the maximum amount of water that can be added depends on the amount of chitin present and its absorption capacity. This work gives information on new biocompatible and biodegradable polymers that can form polymeric organogels. It may be used as a novel polymeric structural agent that may provide opportunities to design materials in the field of organogelation.
References
- Y. Osada and J. P. Gong, Soft and wet materials: Polymer gels, Adv. Mater., 1998, 10(11), 827–837 CrossRef CAS.
- G. M. Kavanagh and S. B. Ross-Murphy, Rheological characterisation of polymer gels, Prog. Polym. Sci., 1998, 23(3), 533–562 CrossRef CAS.
- K. Almdal, J. Dyre, S. Hvidt and O. Kramer, Towards a phenomenological definition of the term ‘gel’, Polym. Gels Networks, 1993, 1(1), 5–17 CrossRef CAS.
- O. Gronwald, E. Snip and S. Shinkai, Gelators for organic liquids based on self-assembly: a new facet of supramolecular and combinatorial chemistry, Curr. Opin. Colloid Interface Sci., 2002, 7(1–2), 148–156 CrossRef CAS.
- G. D. Rees and B. H. Robinson, Microemulsions and organogels – properties and novel applications, Adv. Mater., 1993, 5(9), 608–619 CrossRef CAS PubMed.
- L. E. Buerkle and S. J. Rowan, Supramolecular gels formed from multi-component low molecular weight species, Chem. Soc. Rev., 2012, 41(18), 6089–6102 RSC.
- G. Yu, X. Yan, C. Han and F. Huang, Characterization of supramolecular gels, Chem. Soc. Rev., 2013, 42(16), 6697–6722 RSC.
- S. Tanaka, M. Shirakawa, K. Kaneko, M. Takeuchi and S. Shinkai, Porphyrin-based organogels: Control of the aggregation mode by a pyridine-carboxylic acid interaction, Langmuir, 2005, 21(6), 2163–2172 CrossRef CAS PubMed.
- R. Y. Gong, Y. B. Song, Z. X. Guo, M. Li, Y. Jiang and X. B. Wan, A clickable, highly soluble oligopeptide that easily forms organogels, Supramol. Chem., 2013, 25(5), 269–275 CrossRef CAS PubMed.
- S. Basak, J. Nanda and A. Banerjee, A new aromatic amino acid based organogel for oil spill recovery, J. Mater. Chem., 2012, 22(23), 11658–11664 RSC.
- J. W. Steed, Anion-tuned supramolecular gels: a natural evolution from urea supramolecular chemistry, Chem. Soc. Rev., 2010, 39(10), 3686–3699 RSC.
- F. G. Gandolfo, A. Bot and E. Floter, Structuring of edible oils by long-chain FA, fatty alcohols, and their mixtures, J. Am. Oil Chem. Soc., 2004, 81(1), 1–6 CrossRef CAS.
- R. Kumar and O. P. Katare, Lecithin organogels as a potential phospholipid-structured system for topical drug delivery: A review, AAPS PharmSciTech, 2005, 6, 298–310 CrossRef PubMed.
- C. V. Nikiforidis and E. Scholten, Self-assemblies of lecithin and alpha-tocopherol as gelators of lipid material, RSC Adv., 2014, 4(5), 2466–2473 RSC.
- A. Bot and W. G. M. Agterof, Structuring of edible oils by mixtures of gamma-oryzanol with beta-sitosterol or related phytosterols, J. Am. Oil Chem. Soc., 2006, 83(6), 513–521 CrossRef CAS PubMed.
- B. Jeong, S. W. Kim and Y. H. Bae, Thermosensitive sol-gel reversible hydrogels, Adv. Drug Delivery Rev., 2012, 64, 154–162 CrossRef PubMed.
- S. S. Sagiri, B. Behera, R. R. Rafanan, C. Bhattacharya, K. Pal, I. Banerjee and D. Rousseau, Organogels as Matrices for Controlled Drug Delivery: A Review on the Current State, Soft Mater., 2014, 12(1), 47–72 CrossRef CAS PubMed.
- B. Escuder, F. Rodriguez-Llansola and J. F. Miravet, Supramolecular gels as active media for organic reactions and catalysis, New J. Chem., 2010, 34(6), 1044–1054 RSC.
- R. J. H. Hafkamp, B. P. A. Kokke, I. M. Danke, H. P. M. Geurts, A. E. Rowan, M. C. Feiters and R. J. M. Nolte, Organogel formation and molecular imprinting by functionalized gluconamides and their metal complexes, Chem. Commun., 1997,(6), 545–546 RSC.
- N. E. Hughes, A. G. Marangoni, A. J. Wright, M. A. Rogers and J. W. E. Rush, Potential food applications of edible oil organogels, Trends Food Sci. Technol., 2009, 20(10), 470–480 CrossRef CAS PubMed.
- T. Laredo, S. Barbut and A. G. Marangoni, Molecular interactions of polymer oleogelation, Soft Matter, 2011, 7(6), 2734–2743 RSC.
- M. Suzuki and K. Hanabusa, Polymer organogelators that make supramolecular organogels through physical cross-linking and self-assembly, Chem. Soc. Rev., 2010, 39(2), 455–463 RSC.
- R. Sanchez, J. M. Franco, M. A. Delgado, C. Valencia and C. Gallegos, Development of new green lubricating grease formulations based on cellulosic derivatives and castor oil, Green Chem., 2009, 11(5), 686–693 RSC.
- R. Sanchez, J. M. Franco, M. A. Delgado, C. Valencia and C. Gallegos, Thermal and mechanical characterization of cellulosic derivatives-based oleogels potentially applicable as bio-lubricating greases: Influence of ethyl cellulose molecular weight, Carbohydr. Polym., 2011, 83(1), 151–158 CrossRef CAS PubMed.
- E. Lizaso, M. E. Munoz and A. Santamaria, Formation of gels in ethylcellulose solutions. An interpretation from dynamic viscoelastic results, Macromolecules, 1999, 32(6), 1883–1889 CrossRef CAS.
- C. K. Lee, K. Kitagawa, T. Uchida, N. S. Kim and S. Goto, Transdermal delivery of theophylline using an ethanol panasate 800-ethylcellulose gel preparation, Biol. Pharm. Bull., 1995, 18(1), 176–180 CAS.
- P. W. S. Heng, L. W. Chan and K. T. Chow, Development of novel nonaqueous ethylcellulose gel matrices: Rheological and mechanical characterization, Pharm. Res., 2005, 22(4), 676–684 CrossRef CAS PubMed.
- P. K. Dutta, M. N. Ravikumar and J. Dutta, Chitin and chitosan for versatile applications, J. Macromol. Sci., Part C: Polym. Rev., 2002, 42(3), 307–354 CrossRef.
- H. Nagahama, N. Nwe, R. Jayakumar, S. Koiwa, T. Furuike and H. Tamura, Novel biodegradable chitin membranes for tissue engineering applications, Carbohydr. Polym., 2008, 73(2), 295–302 CrossRef CAS PubMed.
- A. Bornet and P. L. Teissedre, Applications and interest of chitin, chitosan and their derivatives in enology, J. Int. Sci. Vigne Vin, 2005, 39(4), 199–207 CAS.
- S. Ifuku and H. Saimoto, Chitin nanofibers: preparations, modifications, and applications, Nanoscale, 2012, 4(11), 3308–3318 RSC.
- R. Jayakumar, D. Menon, K. Manzoor, S. V. Nair and H. Tamura, Biomedical applications of chitin and chitosan based nanomaterials-A short review, Carbohydr. Polym., 2010, 82(2), 227–232 CrossRef CAS PubMed.
- R. A. A. Muzzarelli, Chitins and Chitosans as Immunoadjuvants and Non-Allergenic Drug Carriers, Mar. Drugs, 2010, 8(2), 292–312 CrossRef CAS PubMed.
- R. Singh, M. P. Chacharkar and A. K. Mathur, Chitin membrane for wound dressing application – preparation, characterisation and toxicological evaluation, Int. Wound J., 2008, 5(5), 665–673 CrossRef PubMed.
- J. Synowiecki and N. A. Al-Khateeb, Production, properties, and some new applications of chitin and its derivatives, Crit. Rev. Food Sci. Nutr., 2003, 43(2), 145–171 CrossRef CAS PubMed.
- B. A. Juárez-de la Rosa, P. Quintana, P. L. Ardisson, J. M. Yáñez-Limón and J. J. Alvarado-Gil, Effects of thermal treatments on the structure of two black coral species chitinous exoskeleton, J. Mater. Sci., 2012, 47(2), 990–998 CrossRef.
- K. Kurita, Chitin and chitosan: Functional biopolymers from marine crustaceans, Mar. Biotechnol., 2006, 8(3), 203–226 CrossRef CAS PubMed.
- P. K. Dutta, J. Dutta and V. S. Tripathi, Chitin and chitosan: Chemistry, properties and applications, J. Sci. Ind. Res., 2004, 63(1), 20–31 CAS.
- R. N. Tharanathan and F. S. Kittur, Chitin - The undisputed biomolecule of great potential, Crit. Rev. Food Sci. Nutr., 2003, 43(1), 61–87 CrossRef CAS PubMed.
- R. Sanchez, G. B. Stringari, J. M. Franco, C. Valencia and C. Gallegos, Use of chitin, chitosan and acylated derivatives as thickener agents of vegetable oils for bio-lubricant applications, Carbohydr. Polym., 2011, 85(3), 705–714 CrossRef CAS PubMed.
- M. V. Tzoumaki, T. Moschakis and C. G. Biliaderis, Metastability of Nematic Gels Made of Aqueous Chitin Nanocrystal Dispersions, Biomacromolecules, 2010, 11(1), 175–181 CrossRef CAS PubMed.
- J. D. Goodrich and W. T. Winter, Alpha-chitin nanocrystals prepared from shrimp shells and their specific surface area measurement, Biomacromolecules, 2007, 8(1), 252–257 CrossRef CAS PubMed.
- R. H. Marchessault, F. F. Morehead and N. M. Walter, Liquid crystal sytsems from fibrillar polysaccharides, Nature, 1959, 184(4686), 632–633 CrossRef CAS PubMed.
-
G. Wypych, Plasticizers use and selection for specific polymers, in Handbook of Plasticizers, ed. G. Wypych, William Andrew Publishing, New York, 2004 Search PubMed.
-
A. G. Marangoni, Polymer Gelation of Oils, 2012 Search PubMed.
-
Q. Xu, M. Nakjima, Z. Liu and T. Shiina, Soybean-based surfactants and their applications, in Soybean - Applications and Technology, ed. T.-B. Ng, InTech, Shangai, 2011 Search PubMed.
- S. D. Doig and R. M. M. Diks, Toolbox for modification of the lecithin headgroup, Eur. J. Lipid Sci. Technol., 2003, 105(7), 368–376 CrossRef CAS PubMed.
- Y. Nakazawa, Y. Sagane, S.-i. Sakurai, M. Uchino, H. Sato, K. Toeda and K. Takano, Large-Scale Production of Phospholipase D from Streptomyces racemochromogenes and Its Application to Soybean Lecithin Modification, Appl. Biochem. Biotechnol., 2011, 165(7–8), 1494–1506 CrossRef CAS PubMed.
- C. Sachs, H. Fabritius and D. Raabe, Hardness and elastic properties of dehydrated cuticle from the lobster Homarus americanus obtained by nanoindentation, J. Mater. Res., 2006, 21(08), 1987–1995 CrossRef CAS.
- R. J. Kline and M. D. McGehee, Morphology and charge transport in conjugated polymer, Polym. Rev., 2006, 46(1), 27–45 CAS.
- E. L. Mogilevskaya, T. A. Akopova, A. N. Zelenetskii and A. N. Ozerin, The crystal structure of chitin and chitosan, Polym. Sci., Ser. A, 2006, 48, 216–226 CrossRef CAS.
-
V. U. S. Nemtsev, N. Kokurina, D. Zagorskaya, N. Kovacheva and V. Larina, Physicochemical properties of chitin from chitin–protein complex of various stages of molting cycle of kamtchatsky crab (Paralithodes camtchaticus) shell, in Progress on Chemistry and Application of Chitin and Its derivatives, ed. M. M. Jaworska, Polish Chitin Society, Lodz, Poland, 2009, vol. XIV Search PubMed.
- I. Henriksen, G. Smistad and J. Karlsen, Interactions between liposomes and chitosan, Int. J. Pharm., 1994, 101(3), 227–236 CrossRef CAS.
- S. Magdassi, U. Bach and K. Y. Mumcuoglu, Formation of positively charged microcapsules based on chitosan-lecithin interactions, J. Microencapsulation, 1997, 14(2), 189–195 CrossRef CAS PubMed.
- T. Yoshioka, B. Sternberg and A. T. Florence, Preparation and properties of vesicles (niosomes) of sorbitan monoesters (Span 20, 40, 60 and 80) and a sorbitan triester (Span 85), Int. J. Pharm., 1994, 105(1), 1–6 CrossRef CAS.
- L. Klapiszewski, M. Wysokowski, I. Majchrzak, T. Szatkowski, M. Nowacka, K. Siwinska-Stefanska, K. Szwarc-Rzepka, P. Bartczak, H. Ehrlich and T. Jesionowski, Preparation and Characterization of Multifunctional Chitin/Lignin Materials, J. Nanomater., 2013, 1–13 CrossRef PubMed.
- K. W. Smith, F. W. Cain and G. Talbot, Effect of nut oil migration on polymorphic transformation in a model system, Food Chem., 2007, 102(3), 656–663 CrossRef CAS PubMed.
- M. A. Rogers, A. J. Wright and A. G. Marangoni, Nanostructuring fiber morphology and solvent inclusions in 12-hydroxystearic acid/canola oil organogels, Curr. Opin. Colloid Interface Sci., 2009, 14(1), 33–42 CrossRef CAS PubMed.
- M. V. Tzoumaki, T. Moschakis, V. Kiosseoglou and C. G. Biliaderis, Oil-in-water emulsions stabilized by chitin nanocrystal particles, Food Hydrocolloids, 2011, 25(6), 1521–1529 CrossRef CAS PubMed.
- L. Sagalowicz, R. Mezzenga and M. E. Leser, Investigating reversed liquid crystalline mesophases, Curr. Opin. Colloid Interface Sci., 2006, 11(4), 224–229 CrossRef CAS PubMed.
- Y. A. Shchipunov, Lecithin organogel: A micellar system with unique properties, Colloids Surf., A, 2001, 183–185(0), 541–554 CrossRef CAS.
- Y. A. Shchipunov, S. A. Mezzasalma, G. J. M. Koper and H. Hoffmann, Lecithin organogel with new rheological and scaling behavior, J. Phys. Chem. B, 2001, 105(43), 10484–10488 CrossRef CAS.
- Y. A. Shchipunov and E. V. Shumilina, Lecithin bridging by hydrogen bonds in the organogel, Mater. Sci. Eng., C, 1995, 3(1), 43–50 CrossRef.
- L.-S. Lai and H.-F. Chiang, Rheology of decolorized hsian-tsao leaf gum in the dilute domain, Food Hydrocolloids, 2002, 16(5), 427–440 CrossRef CAS.
-
B. S. Mitchell, An Introduction to Materials Engineering and Science: For Chemical and Materials Engineers, John Willey & Sons. Inc., New Jersey, 2004 Search PubMed.
Footnote |
† Electronic supplementary information (ESI) available. See DOI: 10.1039/c5ra06451a |
|
This journal is © The Royal Society of Chemistry 2015 |
Click here to see how this site uses Cookies. View our privacy policy here.