DOI:
10.1039/C5RA04544D
(Paper)
RSC Adv., 2015,
5, 40588-40594
Identifying the existence of highly-fluorescent carboxylic group-rich carbon nanodots during a one-pot synthesis of branched polyethylenimine-passivated amine group-rich carbon nanodots†
Received
15th March 2015
, Accepted 20th April 2015
First published on 20th April 2015
Abstract
Carbon nanodots (C-Dots) have recently been proposed as promising alternatives to traditional semiconductor quantum dots and organic fluorophores. However, C-Dots were often prepared and studied as a mixture, and the ignorance of the real composition significantly limits the accurate understanding and control of physical/chemical properties of C-Dots and further practical applications. Herein, we demonstrated and identified for the first time the co-existence of highly-fluorescent carboxylic group-rich C-Dots (CR-C-Dots) and amine group-rich C-Dots (AR-C-Dots) during the one-pot pyrolysis of citric acid and branched polyethylenimine precursors. Transmission electron microscopy demonstrated that the average size of CR-C-Dots was slightly smaller than that of amine group-rich C-Dots (AR-C-Dots), 1.5 nm vs. 1.9 nm. Photophysical characterization indicated that CR-C-Dots had a longer fluorescence lifetime and higher quantum yields (QYs) than the AR-C-Dots, despite the same excitation-independent emission at 445 nm, unlike most previous reports, which stated that amine group passivation enhanced the QYs of C-Dots. Both the C-Dots showed excellent biocompatibility and great potential for cellular imaging. A fluorescence- and electron spin resonance-responsive dual-model probe for ascorbic acid detection was also constructed, further proving the existence of CR-C-Dots. This study facilitates the understanding of the nucleation/surface passivation process during C-Dots formation and the development of a new synthetic and purification strategy for C-Dots.
Introduction
As a new member in the carbon material family, fluorescent carbon nanodots (C-Dots) have attracted tremendous interest since their serendipitous discovery during the purification of single walled carbon nanotubes by arc-discharge methods.1 Owing to their significant advantages in resistance to photobleaching, good biocompatibility, easy preparation and rich surface functional groups for further modification,2,3 C-Dots have been applied in bioimaging,4,5 sensing,6,7 energy conversion,8,9 and drug delivery.10–12 To tune the photophysical properties and structures of C-Dots, several physical or chemical strategies have been developed, which can be classified into top-down (arc-discharge,1 laser-ablation,13 and electrochemical-oxidation14) and bottom-up strategies (pyrolytic methods,15,16 microwave methods,17 and hydrothermal methods18). Among these methodologies, bottom-up methods based on suitable molecular precursors and appropriate carbonization conditions have been demonstrated as an effective pathway to control the structure, size, morphology and photophysical properties of C-Dots.15,19,20
Despite the controversial nature of the photoluminescence of C-Dots, surface passivation with nitrogen-containing organic molecules has been widely accepted as an effective strategy to tune the photophysical properties and functions of C-Dots (such as selective molecule/ion recognition).21 Pioneering work by Sun et al.13 demonstrated the significantly enhanced fluorescence intensity of C-Dots upon surface passivation by diamine-terminated polyethylene glycol. Zeng et al.22 also controlled the emission profiles of C-Dots (excitation-dependent or independent) by simply changing the surface passivation degree of the amine group. Notably, branched polyethylenimine (bPEI) was recently considered as a promising passivation agent due to its excellent ability for surface states control of C-Dots and unique metal ion/nucleic acid binding. For example, a one-pot carbonization and surface functionalization strategy has been recently demonstrated by Chi et al.23,24 to prepare highly luminescent and Cu2+-responsive polyamine-functionalized C-Dots by the pyrolysis of citric acid and bPEI. Liu et al.25 also prepared highly luminescent bPEI-passivated C-Dots for DNA delivery based on the one-pot microwave irradiation of glycerol and bPEI. It should be noted that only a simple dialysis process for purification was conducted in most studies, neglecting the real composition of the C-Dots produced in the mysterious carbonization and passivation process. Is it true that amine-group passivated C-Dots are the solely formed species when preparing bPEI-passivated C-Dots?
It is well-known that the inhomogeneous C-Dots might hinder the accurate understanding and control of physical/chemical properties and limit further practical applications.26 Apparently, the co-existence of unpassivated C-Dots or free bPEI may lead to an incorrect evaluation of the metal/nucleic acid binding or even cellular uptake of the bPEI-passivated C-Dots. Therefore, it is significant to understand the confusing process during the one-pot preparation of bPEI-passivated C-Dots. The possible mechanism of the carbonization of citric acid and bPEI at low temperature has been proposed by Chi et al. as follows: the initially completed carbonization of citric acid and formation of carbonaceous core at low temperature (<200 °C) is followed by the surface passivation of bPEI upon heating for a long-time.24 This suggested that carboxylic group-rich C-Dots (CR-C-Dots) and amine group-rich C-Dots (AR-C-Dots) might simultaneously exist. However, the identification and assignment of the existing species during this process is still challenging.
In the present work, highly-fluorescent CR-C-Dots and AR-C-Dots were simultaneously synthesized and identified for the first time during a one-pot pyrolysis of citric acid and bPEI precursors. Separated by simple Sephadex gel chromatography, two types of C-Dots with different surface properties and fluorescent profiles were obtained and further characterized in detail. A fluorescence and electron spin resonance-responsive dual-model probe for ascorbic acid was also constructed based on as-prepared CR-C-Dots, further proving the existence of CR-C-Dots. Preliminary biocompatibility tests and a cellular imaging study were also conducted.
Experimental
Reagents
Citric acid (anhydrous, 99.5%) was purchased from Alfa Aesar. Branched polyethylenimine (bPEI, MW 1800 Da, 99%), 1-ethyl-3-(3-dimethylaminopropyl) carbodiimide hydrochloride (EDC, 98.5%) and N-hydroxysuccinimide (NHS, 98.5%) were purchased from Aladdin Reagent (Shanghai, China). 4-Amino-2,2,6,6-tetramethylpiperidine 1-oxyl free radical (4-AT, >97.0%) was bought from TCI Co., Ltd (Shanghai, China) and used as received. L-Ascorbic acid (AA, 99%) was obtained from J&K Chemical Ltd (Beijing, China). The dialysis bag (molecular weight cutoff, MWCO 500–1000 Da) was obtained from Ebioeasy Corporation (Shanghai, China). Sephadex G-25 was purchased from GE Healthcare Bio-Science AB (Beijing, China) and used according to the manufacturer's instructions. CuCl2, FeCl3, ammonia water, NaOH and HCl were of analytical grade and purchased from Guangzhou Chemical Reagent Factory. Ultra-pure water (18.2 MΩ) was used in all of the experiments.
Synthesis and separation of C-Dots
C-Dots were synthesized by modifying a previously reported pyrolytic method.23,24 In a typical experiment, 1 g of citric acid and 0.5 g of bPEI were mixed uniformly with 10 mL of water in a 25 mL three-necked round bottomed flask and heated up to 200 °C on a heating mantle in air. Once the yellow gel was formed, 1 mL of ultra-pure water was added. This process was repeated 10 times in 1 h before cooling the reaction mixture to room temperature. The resulting yellow gel was dissolved in 10 mL of ultra-pure water and dialysed against pure water for one day (MWCO 500–1000 Da). The obtained solution was then concentrated with a rotary evaporator and passed through a GE Sephadex G25 chromatography column (2.5 cm × 65 cm), eluted by 0.5% ammonia water for purification. Two types of C-Dots were obtained as yellow powders following lyophilization for further characterization and application.
Synthesis of C-Dots-4-AT
An aqueous solution of CR-C-Dots (fraction 2, 7.5 mg mL−1, 4 mL) and an aqueous solution of NHS (0.9 mM, 4 mL) were mixed under N2 atmosphere in a 25 mL three-necked flask followed by the addition of 4 mL of an aqueous solution of EDC (0.9 mM). After vigorous stirring for 20 min, 60 mg of 4-AT dissolved in 5 mL of ultrapure water was added dropwise into the flask over a period of 5 min. Then, the reaction mixture solutions were adjusted to a pH value of 8–9 with a sodium hydroxide solution (1 M). Followed by stirring overnight in the dark at room temperature, the solution was dialysed for a week in the dark against ultra-pure water through a dialysis membrane (MWCO 500–1000 Da).
Characterization
Absorption spectra were obtained on a Varian Cary 300 UV-vis spectrometer, and photoluminescence spectra were recorded by a Shimadzu RF-5301PC spectrofluorophotometer. Fluorescence lifetime measurements were conducted on an Edinburgh Instruments FLS 920 combined fluorescence lifetime and steady state spectrometer. Lifetime decay curves were fitted to an exponential formula F(t) = A + B1
exp(−t/τ1) + B2
exp(−t/τ2) + B3
exp(−t/τ3) + B4
exp(−t/τ4), and the average lifetime was calculated according to the equation τav = (B1τ12 + B2τ22 + B3τ32 + B4τ42)/(B1τ1 + B2τ2 + B3τ3 + B4τ4). Fourier transform infrared (FT-IR) spectra were obtained on a NEXUS FT-IR spectrometer (Thermo Nicolet, USA) using KBr pellets. Transmission electron microscope (TEM) measurements were performed on a JEOL JEM-2010HR microscope with an accelerating voltage of 200 kV. Samples for TEM measurements were deposited onto an ultrathin carbon film supported by 300-mesh copper grids. X-ray photoelectron spectroscopy (XPS) was performed on a Thermo-VG Scientific ESCALAB 250 spectrometer. Photoelectrons were generated by a mono Al Kα X-ray radiation of energy 1486.6 eV and the calibration was based on C1s with the binding energy at 284.8 eV. Samples for XPS analysis were prepared by depositing aqueous solutions of C-Dots onto a piranha solution and aqua regia treated silicon wafer and then dried in air at room temperature for one day. All the spectral peaks were de-convoluted by the XPSPEAK software version 4.1 with the Shirley method for background subtraction and fitted by Gaussian–Lorentzian functions. Confocal microscopy images were obtained with a confocal laser scanning fluorescence microscope (Zeiss LSM-710, Carl Zeiss, Göttingen, Germany). ESR measurements were carried out using a Bruker EMX A300 spectrometer.
Quantum yields measurements
The quantum yields (QYs) of the as-synthesized C-Dots, following excitation at 360 nm, were measured according to an established procedure.19,27 Quinine sulfate in 0.1 M H2SO4 (QYs 0.54 at 360 nm) was chosen as a standard. To minimize reabsorption effects, the absorbance of C-Dots at 360 nm was controlled to be ca. 0.05 when using a 10 mm fluorescence cuvette.
Cell cytotoxicity tests (MTT)
MTT assay was carried out to evaluate the cytotoxicity of the C-Dots on A549 cell, MCF7 and HeLa cell lines. Cells were cultured in 96-well tissue culture plates (Corning) at a density of 1 × 104 cells per well. After 24 h of incubation, the cells were treated with various concentrations (0.0625, 0.125, 0.25, 0.5, and 1 mg mL−1) of the C-Dots. After incubation for 48 h, 20 μL of MTT (5 mg mL−1) was added into each well. The cells were further incubated for 4 h, the culture medium was removed and 150 μL of DMSO was added to dissolve the formazan product. The cell viability was evaluated by measuring the absorbance at 490 nm after shaking for 6 min.
Cell imaging
A549 cells were cultured in 35 mm dishes for 24 h and cells were incubated with C-Dots (0.5 mg mL−1) for 5 h. Then, the medium was removed and the cells were washed three times with cold PBS. Cellular images were obtained immediately by confocal microscopy.
Electron spin resonance (ESR)
ESR measurements were carried out at ambient temperature. 50 μL of sample solutions were placed in glass capillary tubes with internal diameters of 1 mm and sealed. The tubes were inserted into the ESR cavity and measured. ESR parameter settings were as follows: microwave frequency 9.87 GHz, modulation amplitude 0.04 G, modulation frequency 100 kHz, scan range 500 G, microwave power 1 mW and time constant 40.96 ms.
Result and discussion
Synthesis, separation and identification of CR-C-Dots and AR-C-Dots
Following the pyrolysis of citric acid and bPEI at 200 °C for 1 h, the resulting reaction mixture was dialysed against pure water to remove the small molecule precursor.28 As illustrated in Scheme 1, when separated and eluted from a Sephadex G25 column, two fractions on the column exhibited strong blue fluorescence, while the spacing between them were weakly emitted upon excitation at 365 nm using a handed UV lamp. This allowed for easy separation and collection of these two fluorescent fractions, denoted as fraction 1 and 2, respectively (Scheme 1). It should be noted that the extremely weak emitting fraction could also be collected prior to fraction 1, the morphology of which was like a viscous oil following evaporation and fitted well with the phenotype of unreacted bPEI. The sufficient difference of retention time between bPEI and fraction 1 provided the possibility to remove unreacted bPEI. Low content of ammonia water was necessary to reduce the interaction between amino group-rich composition and the hydroxyl group of dextrin during chromatography separation. The weight yields of fraction 1 and 2 were 14.6% and 25.2%, respectively, with respect to the content of citric acid and bPEI. The two highly fluorescent fractions were systematically characterized by TEM, XPS, FT-IR, UV-vis, FL, and fluorescence lifetime decays.
 |
| Scheme 1 Schematic representation of one-pot pyrolysis of citric acid and bPEI precursors and separation of formed AR-C-Dots and CR-C-Dots. | |
TEM characterization indicated that both the fractions consisted of small-sized C-Dots, which were nearly spherical in shape and uniformly distributed without any agglomeration (Fig. 1a and b). Their size distribution histograms (inset in Fig. 1a and b) indicated that the average size of fraction 1 was slightly larger than that of fraction 2 (1.9 nm vs. 1.5 nm). This is quite identical to the gel filtration principle, which states that the retention time on the G25 column is mainly determined by the molecular size, i.e., larger molecules elute first followed by smaller ones.
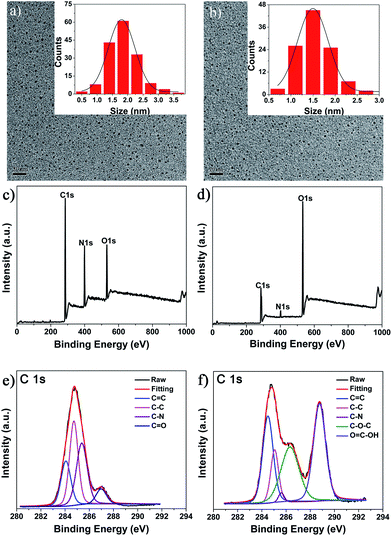 |
| Fig. 1 TEM and XPS characterization of the as-synthesized C-Dots. (a, c and e) for fraction 1 and (b, d and f) for fraction 2. The insets are the corresponding size distribution of fractions. Scale bars in the TEM images: 20 nm. | |
An XPS assay was used to characterize the chemical composition of the obtained C-Dots. Both the as-synthesized C-Dots were composed of carbon, nitrogen and oxygen (Fig. 1c and d), but more nitrogen content was present in fraction 1, while more oxygen was present in fraction 2. As shown in Fig. 1e, the C1s peaks of fraction 1 at 284.1, 284.5, 285.2 and 287.2 eV can be assigned to carbon in the form of C
C (sp2), C–C (sp3), C–N (sp3) and C
O (sp2), respectively.24,29 For fraction 2, the observed C1s signals at 284.1, 284.5, 286.2, 287.8 and 289.1 eV can be assigned to carbon in the form of C
C (sp2), C–C (sp2), C–N (sp3), C–O–C (sp3) and O
C–OH, respectively.24,30 Quantitative analysis indicated that fraction 1 had less C
C (sp2) groups, but more C–C (sp3) and C–N (sp3) groups than fraction 2, suggesting that more bPEI passivation occurred in fraction 1. Much more C–O–C (sp3) and O
C–OH groups could be observed in fraction 2, further supporting that fraction 2 was less passivated by bPEI. Much more C–N (sp3) and the low content of C
O group in fraction 1 may be explained by the further reaction of bPEI with C–O–C (sp3) and O
C–OH groups surrounding the formed carbon core. The slightly bigger size of fraction 1 than 2 might also support the covalent bonding of bPEI onto the surface of the carbon core of fraction 1.
FT-IR spectroscopy was used to identify the organic functional groups on the surface of the as-synthesized C-Dots. As shown in Fig. 2, the absorbance of fraction 1 at 3404, 2950, 2839, 1577, 1459 and 1297 cm−1 was considerably similar to that of bPEI, suggesting that fraction 1 was successfully passivated by bPEI.31 New peaks at 1644 cm−1 can be assigned to the C
O stretching vibration of amide group (Amide I), indicating the amide linkage of bPEI in fraction 1.25 In contrast, the infrared absorbance of fraction 2 was considerably different from pure citric acid, bPEI or fraction 1. A new absorbance peak at 1728 cm−1 and a broad absorbance at 3130 cm−1 is attributed to C
O and OH stretching vibration of carboxyl group, respectively. The strong absorbance at 1400 cm−1 can be assigned to the in-plain bending vibration of the OH group on the surface of fraction 2. The absence of absorbance peaks at 2950 and 2839 cm−1 (CH2 stretching vibration) also suggested that fraction 2 was not passivated by bPEI.
 |
| Fig. 2 FT-IR characterization of the as-synthesized C-Dots. | |
The UV-vis absorption spectra of fraction 1 and 2 and their photoluminescence (PL) spectra are shown in Fig. 3a and b, respectively. Both the C-Dots exhibited similar absorbance bands centered at 240 nm and 360 nm, which can be attributed to the π–π* transition of nanocarbon and the n–π* transition of C
O; this observation is similar to previous reports.24,32,33 Interestingly, the emission peak at 445 nm was observed in emission spectra of both fraction 1 and 2, although nanoparticles in both fractions have different sizes. This phenomenon further supported that the photoluminescence of C-Dots was determined not only by the size.19 When the excitation wavelength was increased from 360 to 400 nm, the emission peak of both the C-Dots did not shift, implying a relatively uniform surface emission trap state.13,34 However, the maxima excitation wavelength was slightly different, namely, 370 nm for fraction 1 and 360 nm for fraction 2, respectively, suggesting that the as-synthesized C-Dots had different structures. Quantum yields were 8.2% for fraction 1 and 17.4% for fraction 2, considerably different from most previous reports, which stated that surface passivation enhanced the quantum yields of C-Dots.10,13,35 One possible reason for this observation was that photoinduced electron transfer (PET) quenching of C-Dots occurred from nitrogen atoms of bPEI to the luminescent carbon core in fraction 1, similar to that observed for CdSe/ZnS core/shell quantum dots quenched by nitrogen atoms in a surface-linked azamacrocycle.36
 |
| Fig. 3 UV-vis/excitation/emission and fluorescence lifetime characterization of as-synthesized C-Dots. (a and c) for fraction 1 and (b and d) for fraction 2. | |
The fluorescence lifetime measurements indicated that the average decay time was 10.57 ± 0.13 ns for fraction 2, which is longer than 6.36 ± 0.12 ns for fraction 1, as shown in Fig. 3 and Table S1.† The influence of pH and sodium chloride concentration on both the types of C-Dots was also investigated. As shown in Fig. S1a and b,† the maximum fluorescence intensity of fraction 1 was observed at pH 5, whereas fraction 2 showed the highest fluorescence intensity at pH 6 (nearly neutral), suggesting that different surface groups surrounded the fluorescent C-Dots.37 Both the C-Dots displayed good fluorescence stability in the presence of sodium chloride (even up to 2 M) (Fig. S1c and d†), implying the potential of as-synthesized C-Dots for cell imaging applications.
The influences of Cu2+ and Fe3+ on the fluorescence of fraction 1 and fraction 2 were also checked. As shown in Fig. S2,† Cu2+ quenched the fluorescence of fraction 1 more effectively than Fe3+ (Kq of 8.27 × 106 for Cu2+ and 2.47 × 106 for Fe3+), which was different from the case of fraction 2 (Kq of 2.44 × 104 for Cu2+ and 3.25 × 106 for Fe3+). The result was considerably identical to previous reports that Cu2+ had a higher thermodynamic affinity and faster chelating process with “N” on the surface of C-Dots, whereas Fe3+ exhibited good binding affinity for hydroxyl groups surrounding the carbon core.18,38 All these results provided solid evidence that AR-C-Dots (fraction 1) and CR-C-Dots (fraction 2) were simultaneously formed during the one-pot pyrolysis of citric acid and bPEI precursors, which was not observed in previous investigations on the preparation of bPEI-functionalized C-Dots.23,24
ESR and fluorescence dual-responsive probe for ascorbic acid (AA) based on CR-C-Dots
Due to their excellent photophysical properties, good biocompatibility and rich surface functional group content, C-Dots have been used to fabricate sensors for inorganic ions6,23,39,40 and molecule (glucose,41 AA42 and H2S43). Inspired by this, we tried to utilize as-synthesized C-Dots to construct electron spin resonance and fluorescent dual model probes for typical antioxidant AA sensing to further prove the existence of CR-C-Dots. We conjugated as-synthesized CR-C-Dots with paramagnetic nitroxide radical 4-AT through amide condensation, as indicated in Fig. 4a. Through a prolonged time dialysis process (one week), C-Dots-4-AT complexes were obtained, as evidenced by the observed ESR signal (triplet peaks), UV-vis absorbance and FT-IR spectroscopy (Fig. S3 and S4†). Fig. 4b and c show the ESR and fluorescence spectra of the response of C-Dots-4-AT to AA, respectively. With the addition of AA, the prepared C-Dots-4-AT exhibited an ESR off–on and FL on–off dual-model response to AA at the μM level. The mechanism for ascorbic acid detection has been proposed by Guo et al. in Scheme S1.†42 Paramagnetic 4-AT with a singly occupied molecular orbital (SOMO) is an electron acceptor. The new non-radiative energy relaxation pathway competes with the fluorescence (FL) process of C-Dots, and therefore the C-Dots emitted weakly. Upon the addition of AA, paramagnetic 4-AT was reduced to diamagnetic hydroxylamine. The non-radiative electron transfer pathway was blocked and the FL of C-Dots was restored. Therefore, the existence of CR-C-Dots was further proven.
 |
| Fig. 4 Construction of ESR and fluorescence dual-responsive probe for ascorbic acid (AA) detection based on CR-C-Dots. (a) Rational design of AA dual-responsive nanoprobe C-Dots-4-AT. (b) and (c) The ESR signal diminishment and fluorescence enhancement of C-Dots-4-AT upon the addition of AA, respectively. | |
Cell viability and bioimaging application of the obtained C-Dots
The cytotoxicity of AR-C-Dots and CR-C-Dots was evaluated by an MTT assay. Fig. 5a and b show the viability of three cell lines after incubation with AR-C-Dots and CR-C-Dots at various concentrations for 48 h. The MTT results suggested that the cytotoxicity of the two types of C-Dots was negligible up to 250 μg mL−1. Owing to their unique PL properties and low cytotoxicity, the potential of the obtained AR-C-Dots and CR-C-Dots for bioimaging was demonstrated on A549 cells. Following incubation with both the C-Dots at the same concentration for 5 h, strong fluorescence was observed in the A549 cytoplasm in both the cases by confocal laser scanning microscopy (Fig. 5c and d). Control experiments without the addition of as-synthesized C-Dots were carried out to ensure that the autofluorescence from the cells under the same conditions were eliminated (data not shown). It was found that AR-C-Dots displayed lower quantum yields than CR-C-Dots in solutions; however, slightly stronger fluorescence signals were observed for AR-C-Dots in cell cytoplasm. This indicated easier cellular uptake of AR-C-Dots than CR-C-Dots. This may be due to enhanced interactions between positively charged AR-C-Dots and cell membrane, also suggesting that AR-C-Dots are rich in amino groups.
 |
| Fig. 5 Cell viability assay and bioimaging applications of the as-synthesized C-Dots. (a and c) for AR-C-Dots and (b and d) for CR-C-Dots. Scale bar: 20 μm. | |
Conclusions
In this study, the co-existence of highly-fluorescent CR-C-Dots and AR-C-Dots during the one-pot pyrolysis of citric acid and bPEI precursors was first identified. Based on the differences in the size and surface structure, both the C-Dots could be easily separated by Sephadex G25 chromatography. FT-IR spectra and XPS analysis verified the different surface properties of the two types of C-Dots; CR-C-Dots have a slightly smaller diameter than AR-C-Dots (1.5 nm vs. 1.9 nm). Interestingly, CR-C-Dots exhibit longer fluorescence lifetime and higher quantum yields than AR-C-Dots, which was considerably different from most previous reports, which stated that amine group passivation enhanced the quantum yields of C-Dots. A fluorescence and ESR dual-responsive nanoprobe for ascorbic acid detection was also constructed to further prove the existence of CR-C-Dots. Preliminary results suggested both the C-dots have excellent biocompatibility and great potential for cellular imaging. This study helps to understand the nucleation/surface passivation process during C-Dots formation and can facilitate the development of new synthetic/purification strategies for C-Dots.
Acknowledgements
This work was financially supported by the National Natural Science Foundation of China (no. 21231007), 973 Program (no. 2014CB845604), the Ministry of Education of China (nos IRT1298 and 313058), Guangdong Provincial Government and the Fundamental Research Funds for the Central Universities.
Notes and references
- X. Xu, R. Ray, Y. Gu, H. J. Ploehn, L. Gearheart, K. Raker and W. A. Scrivens, J. Am. Chem. Soc., 2004, 126, 12736–12737 CrossRef CAS PubMed.
- S. N. Baker and G. A. Baker, Angew. Chem., Int. Ed., 2010, 49, 6726–6744 CrossRef CAS PubMed.
- H. T. Li, Z. H. Kang, Y. Liu and S. T. Lee, J. Mater. Chem., 2012, 22, 24230–24253 RSC.
- L. Cao, X. Wang, M. J. Meziani, F. Lu, H. Wang, P. G. Luo, Y. Lin, B. A. Harruff, L. M. Veca, D. Murray, S.-Y. Xie and Y.-P. Sun, J. Am. Chem. Soc., 2007, 129, 11318–11319 CrossRef CAS PubMed.
- S.-T. Yang, L. Cao, P. G. Luo, F. Lu, X. Wang, H. Wang, M. J. Meziani, Y. Liu, G. Qi and Y.-P. Sun, J. Am. Chem. Soc., 2009, 131, 11308–11309 CrossRef CAS PubMed.
- W. Shi, X. Li and H. Ma, Angew. Chem., Int. Ed., 2012, 51, 6432–6435 CrossRef CAS PubMed.
- A. Zhu, Q. Qu, X. Shao, B. Kong and Y. Tian, Angew. Chem., Int. Ed., 2012, 51, 7185–7189 CrossRef CAS PubMed.
- L. Cao, S. Sahu, P. Anilkumar, C. E. Bunker, J. Xu, K. A. S. Fernando, P. Wang, E. A. Guliants, K. N. Tackett and Y.-P. Sun, J. Am. Chem. Soc., 2011, 133, 4754–4757 CrossRef CAS PubMed.
- H. Choi, S.-J. Ko, Y. Choi, P. Joo, T. Kim, B. R. Lee, J.-W. Jung, H. J. Choi, M. Cha, J.-R. Jeong, I.-W. Hwang, M. H. Song, B.-S. Kim and J. Y. Kim, Nat. Photonics, 2013, 7, 732–738 CrossRef CAS.
- P. Huang, J. Lin, X. Wang, Z. Wang, C. Zhang, M. He, K. Wang, F. Chen, Z. Li, G. Shen, D. Cui and X. Chen, Adv. Mater., 2012, 24, 5104–5110 CrossRef CAS PubMed.
- M. Zheng, S. Liu, J. Li, D. Qu, H. Zhao, X. Guan, X. Hu, Z. Xie, X. Jing and Z. Sun, Adv. Mater., 2014, 26, 3554–3560 CrossRef CAS PubMed.
- Y. Choi, S. Kim, M.-H. Choi, S.-R. Ryoo, J. Park, D.-H. Min and B.-S. Kim, Adv. Funct. Mater., 2014, 24, 5781–5789 CrossRef CAS PubMed.
- Y.-P. Sun, B. Zhou, Y. Lin, W. Wang, K. A. S. Fernando, P. Pathak, M. J. Meziani, B. A. Harruff, X. Wang, H. Wang, P. G. Luo, H. Yang, M. E. Kose, B. Chen, L. M. Veca and S.-Y. Xie, J. Am. Chem. Soc., 2006, 128, 7756–7757 CrossRef CAS PubMed.
- L. Bao, Z.-L. Zhang, Z.-Q. Tian, L. Zhang, C. Liu, Y. Lin, B. Qi and D.-W. Pang, Adv. Mater., 2011, 23, 5801–5806 CrossRef CAS PubMed.
- A. B. Bourlinos, R. Zbořil, J. Petr, A. Bakandritsos, M. Krysmann and E. P. Giannelis, Chem. Mater., 2012, 24, 6–8 CrossRef CAS.
- A. B. Bourlinos, M. A. Karakassides, A. Kouloumpis, D. Gournis, A. Bakandritsos, I. Papagiannouli, P. Aloukos, S. Couris, K. Hola, R. Zboril, M. Krysmann and E. P. Giannelis, Carbon, 2013, 61, 640–643 CrossRef CAS PubMed.
- H. Zhu, X. Wang, Y. Li, Z. Wang, F. Yang and X. Yang, Chem. Commun., 2009, 5118–5120 RSC.
- S. Zhu, Q. Meng, L. Wang, J. Zhang, Y. Song, H. Jin, K. Zhang, H. Sun, H. Wang and B. Yang, Angew. Chem., Int. Ed., 2013, 52, 3953–3957 CrossRef CAS PubMed.
- S. Hu, A. Trinchi, P. Atkin and I. Cole, Angew. Chem., Int. Ed., 2015, 54, 2970–2974 CrossRef CAS PubMed.
- D. Qu, M. Zheng, L. Zhang, H. Zhao, Z. Xie, X. Jing, R. E. Haddad, H. Fan and Z. Sun, Sci. Rep., 2014, 4, 5294 CAS.
- C. Ding, A. Zhu and Y. Tian, Acc. Chem. Res., 2013, 47, 20–30 CrossRef PubMed.
- X. Li, S. Zhang, S. A. Kulinich, Y. Liu and H. Zeng, Sci. Rep., 2014, 4, 4976 CAS.
- Y. Dong, R. Wang, G. Li, C. Chen, Y. Chi and G. Chen, Anal. Chem., 2012, 84, 6220–6224 CrossRef CAS PubMed.
- Y. Dong, R. Wang, H. Li, J. Shao, Y. Chi, X. Lin and G. Chen, Carbon, 2012, 50, 2810–2815 CrossRef CAS PubMed.
- C. Liu, P. Zhang, X. Zhai, F. Tian, W. Li, J. Yang, Y. Liu, H. Wang, W. Wang and W. Liu, Biomaterials, 2012, 33, 3604–3613 CrossRef CAS PubMed.
- J. C. Vinci, I. M. Ferrer, S. J. Seedhouse, A. K. Bourdon, J. M. Reynard, B. A. Foster, F. V. Bright and L. A. Colon, J. Phys. Chem. Lett., 2013, 4, 239–243 CrossRef CAS.
- A. M. Brouwer, Pure Appl. Chem., 2011, 83, 2213–2228 CrossRef CAS.
- M. J. Krysmann, A. Kelarakis, P. Dallas and E. P. Giannelis, J. Am. Chem. Soc., 2012, 134, 747–750 CrossRef CAS PubMed.
- K. Linehan and H. Doyle, J. Mater. Chem. C, 2014, 2, 6025–6031 RSC.
- Y. Hu, J. Yang, J. Tian, L. Jia and J.-S. Yu, Carbon, 2014, 77, 775–782 CrossRef CAS PubMed.
- L. Shen, L. Zhang, M. Chen, X. Chen and J. Wang, Carbon, 2013, 55, 343–349 CrossRef CAS PubMed.
- A. Jaiswal, S. S. Ghosh and A. Chattopadhyay, Chem. Commun., 2012, 48, 407–409 RSC.
- X. Zhai, P. Zhang, C. Liu, T. Bai, W. Li, L. Dai and W. Liu, Chem. Commun., 2012, 48, 7955–7957 RSC.
- Y. Liu, N. Xiao, N. Gong, H. Wang, X. Shi, W. Gu and L. Ye, Carbon, 2014, 68, 258–264 CrossRef CAS PubMed.
- C. J. Liu, P. Zhang, F. Tian, W. C. Li, F. Li and W. G. Liu, J. Mater. Chem., 2011, 21, 13163–13167 RSC.
- M. J. Ruedas-Rama and E. A. H. Hall, Anal. Chem., 2008, 80, 8260–8268 CrossRef CAS PubMed.
- S. H. Jin, D. H. Kim, G. H. Jun, S. H. Hong and S. Jeon, ACS Nano, 2012, 7, 1239–1245 CrossRef PubMed.
- A. Zhao, C. Zhao, M. Li, J. Ren and X. Qu, Anal. Chim. Acta, 2014, 809, 128–133 CrossRef CAS PubMed.
- H. X. Zhao, L. Q. Liu, Z. D. Liu, Y. Wang, X. J. Zhao and C. Z. Huang, Chem. Commun., 2011, 47, 2604–2606 RSC.
- J.-M. Liu, L.-p. Lin, X.-X. Wang, L. Jiao, M.-L. Cui, S.-L. Jiang, W.-L. Cai, L.-H. Zhang and Z.-Y. Zheng, Analyst, 2013, 138, 278–283 RSC.
- W. B. Shi, Q. L. Wang, Y. J. Long, Z. L. Cheng, S. H. Chen, H. Z. Zheng and Y. M. Huang, Chem. Commun., 2011, 47, 6695–6697 RSC.
- F. Lin, D. Pei, W. He, Z. Huang, Y. Huang and X. Guo, J. Mater. Chem., 2012, 22, 11801–11807 RSC.
- C. Yu, X. Li, F. Zeng, F. Zheng and S. Wu, Chem. Commun., 2013, 49, 403–405 RSC.
Footnote |
† Electronic supplementary information (ESI) available: Fig. S1–S4. See DOI: 10.1039/c5ra04544d |
|
This journal is © The Royal Society of Chemistry 2015 |
Click here to see how this site uses Cookies. View our privacy policy here.