DOI:
10.1039/C5RA03017J
(Paper)
RSC Adv., 2015,
5, 33546-33553
Non-solvent-based pretreatment of poly(3-hydroxybutyrate) for improved bio-based crotonic acid production
Received
17th February 2015
, Accepted 30th March 2015
First published on 30th March 2015
Abstract
In this study, high purity bio-based crotonic acid was obtained by a non-solvent-based pretreatment of poly(3-hydroxybutyrate), PHB, prior to pyrolysis. PHB was produced by Cupriavidus necator KCTC 2649 utilizing heat-treated oil palm frond juice followed by mild alkaline treatment with 0.05 M NaOH. It was found that NaOH-treated PHB was highly converted to its dehydrated monomer to give bio-based crotonic acid with 89% purity; 16% higher than that produced from chloroform-treated PHB. It is believed that pretreatment of PHB with low concentration NaOH assisted in high thermal conversion of PHB into crotonic acid by producing a crotonyl chain-end and Na-binding carboxyl terminal end, which both accelerate the β-chain scission of PHB into biocrotonic acid. The initial molar mass of PHB also played a role in biocrotonic acid production. Overall, improved biocrotonic acid production with high purity is an advantage for industrial production of crotonic acid from renewable resources.
Introduction
Chemicals from renewable resources have recently attracted attention from consumers.1 This has been contributed to by the global increase in oil prices as well as the depletion of natural gas. Hence alternative routes for industrial chemical production, such as crotonic acid, need to be considered.2
Crotonic acid is an unsaturated carboxylic acid which is usually produced from the oxidation of petroleum-based crotonaldehyde. It acts as an alternative to acrylic acid in the manufacturing of polymers and plastics.3 In addition, crotonic acid can be converted to crotonyl-CoA to be used as a precursor in fermentation or enzymatic studies.4 There has been research on the utilization of crotonic acid as a precursor for macrolide synthesis and to promote de novo synthesis.5 On the other hand, grafting of crotonic acid with hydrophobic plastics would also make the plastics more hydrophilic and hence suitable for biomedical applications while copolymerization of crotonic acid with other polymers can lead to hydrogel production.6,7 It has been widely reported that crotonic acid and its derivatives are mainly used as components of hair styling products, paints, insecticides, a softening agent for synthetic rubber, a coating resin and plasticizer.8–12 Recently, crotonic acid was suggested as a platform for the production of industrially important chemicals such as acrylic acid, n-butanol, 2-propylene, maleic anhydride and fumaric acid through chemical reactions such as metathesis, hydrogenation, decarboxylation and oxidation.2,13
Crotonic acid can be found in two forms by different arrangement of substituents at double bond which is trans-crotonic acid and cis-crotonic acid. In industrial production of crotonic acid, crotonaldehyde is oxidized to produce mainly trans-crotonic acid. trans-Crotonic acid is more stable and commercially available in the market. On the other hand, cis-crotonic acid is not usually produced in bulk because of its unstable structure.14
Current industrial crotonic acid produced through chemical synthesis involves many steps; from ethylene production by petroleum cracking, followed by oxidation of ethylene into acetaldehyde, aldol condensation of acetaldehyde into acetaldol, dehydration of acetaldol into crotonaldehyde and lastly oxidation of crotonaldehyde into crotonic acid.15 After all the complicated steps, the current yield of petroleum-based CA is only 30%.16 On the other hand, purification of CA in industry involves fractional distillation and crystallization from water in order to obtain pure crotonic acid. Highly contaminated effluent is formed during the crystallization process and this step also causes product loss.17 Overall, low yield of crotonic acid and environmental issue have been the shortcomings for CA production from petrochemical route.
There have been reports on biological crotonic acid production by transgenic cell from selective species such as Ralstonia eutropha, Escherichia coli, Corynebacterium glutamicum and Clostridium acetobutylicum.3 The method manipulated transgenic bacterial cell pathway via over expression of specific enzymes. Bio-based crotonic acid can be produced by altering the bacterial 2-oxoglutarate pathway with over expression of specific enzymes such as 2-hydroxyglutarate dehydrogenase, glutaconate-CoA transferase, hydroxyglutaryl-CoA dehydratase and glutaconyl-CoA decarboxylase. Other invention by Koch and Meurer (2012)18 introducing a recombinant cell with elevated activity of enzymes involved in 2-ketoglutarate pathway. Crotonic acid can be produced through bacterial fermentation of Corynebacterium glutamicum strain by altering the enzyme activity (CoA-transferase).
Van Walsem et al. (2012)13 reported on monomer production from genetically modified polyhydroxyalkanoate (PHA) producers; either plant or bacterial cells. The biomass containing PHA was heated in the presence of catalyst to release monomeric products. However the purity of crotonic acid produced was not reported. On the other hand, Mamat et al. (2014)16 has recently reported on alternative route for the production of crotonic acid, i.e. by pyrolysis of bacterial poly(3-hydroxybutyrate), PHB inclusions. This newly proposed bio-based crotonic acid production method is regarded advantageous over the current industrial production of crotonic acid as the material resource used is renewable, the method has less number of production steps and it contributes to the higher production yield. Furthermore, bio-based CA production method proposed by Mamat et al. (2014)16 is industrially applicable since the process needs no further crystallization step as the CA formed during pyrolysis is in the form of crystals. The overall yield recorded was 63.7% while the composition of trans- and cis-crotonic acid in pyrolyzates was only 51.7% and 2.8%, respectively. This has been the shortcoming of this method since the pyrolyzates contained other components such as 3-hydroxybutyric acid (3-HB), dimer, trimer and other impurities. This has led to the low purity of CA produced.
It is believed that pretreatment of PHA aimed at purifying the polymer prior to pyrolysis could contribute to the higher purity of crotonic acid produced. PHA can be purified either by chemical (chloroform, sodium hypochlorite, alkali digestion), biological (enzymatic digestion), mechanical (bead mill, high pressure homogenization) and physical (ultrasonication, osmotic shock, freezing) treatments. Mohammadi and colleague28 have recently developed a new method which involved the use of mild alkaline solution. It was reported that PHA purification by 0.05 M NaOH contributed to 96% purity of PHA. This result is comparable with conventional method of PHA purification using chloroform.
In this paper, we intend to demonstrate the production of high purity bio-based crotonic acid from bacterial PHB by introducing a mild alkaline pretreatment step prior to pyrolysis. PHB used in this study was produced from fermentation of heat-treated oil palm frond (OPF) juice by C. necator KCTC 2649. Mild NaOH treatment was conducted prior to pyrolysis as a pretreatment step in order to improve the purity of PHB and consequently, the purity of biocrotonic acid.
Results and discussion
Effect of OPF juice heating on PHB production
It has been recently reported that OPF juice can be an alternative novel fermentation feedstock for the production of PHB and other value-added products.16,19 OPF juice contains some amount of amino acids19 which might produce Maillard reaction during heat sterilization. In this study, effect of OPF juice heating on PHB fermentation was clarified. Fig. 1 shows the growth profile of cell dry weight (CDW), PHB content and sugar concentration throughout the fermentation of PHB by C. necator KCTC 2649 in three different carbon sources: mixture of synthetic sugars, autoclaved OPF juice and filter-sterilized OPF juice. It was seen that the cells grew steadily until stationary phase in all experiments. Similar trend was seen for sugar consumption profile in all experiments. Nevertheless, PHB production profile showed that PHB production in synthetic sugar medium was lower compared to the others. This was due to the low cell biomass production in the medium. Higher CDW at 15.9 and 16.7 g L−1 for autoclaved and filter-sterilized OPF juice was recorded, respectively (Table 1). The results showed that the bacterial cells can grow better in OPF juice than in synthetic sugars. This can be explained by the presence of other organic compounds such as amino acids, carbohydrates and other essential minerals for bacterial growth.19 Based on our results, we can conclude that heating the OPF juice prior to fermentation did not affect the growth of bacterium and PHB production from C. necator KCTC 2649. Our result is in agreement with Maail et al. (2014)20 whereby it was reported that there was no inhibition of growth for several microorganisms tested in heat-treated OPF juice. Overall, fed-batch fermentation of PHB in 20 L bioreactor produced 24 g L−1 of cells with 75% PHB content.
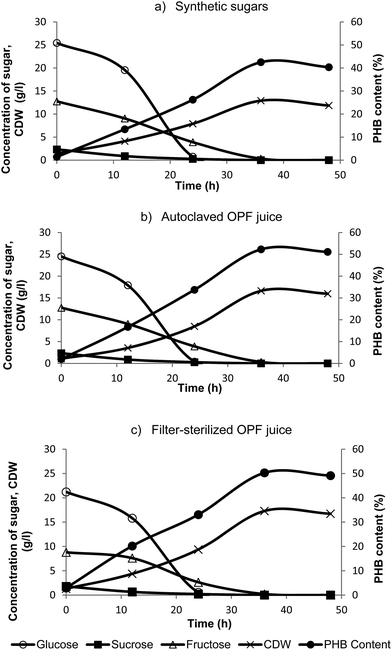 |
| Fig. 1 Fermentation profile of Cupriavidus necator KCTC 2649 in Erlenmeyer flask at 200 rpm and 30 °C with different carbon sources: (a) synthetic sugars, (b) autoclaved OPF juice and (c) filter-sterilized OPF juice. | |
Table 1 Comparison of PHB fermentation by Cupriavidus necator KCTC 2649 in different carbon sources
Carbon source for fermentation |
Cell dry mass (g L−1) |
PHB concentration |
(%) |
(g L−1) |
Mixture of synthetic sugars |
11.9 |
40.4 ± 2.5 |
4.8 |
Autoclaved OPF juice |
15.9 |
51.1 ± 1.5 |
8.2 |
Filter-sterilized OPF juice |
16.7 |
49.1 ± 1.9 |
8.2 |
Biocrotonic acid production from pre-treated PHB biomass
Pretreatment of PHB with NaOH and chloroform improved its purity to 92 and 99%, respectively, which was higher compared to untreated PHB (75%). TG analysis of untreated and treated PHB samples showed smooth decomposition from beginning until the end of the process (Fig. 2). Chloroform-treated PHB as control sample in this study was fully degraded and there was no carbonaceous residue left in the sample pan. On the other hand, untreated PHB biomass showed an early decomposition between 40 and 300 °C which was due to volatilization of other components in the cell (non-polymer cellular material, NPCM) such as moisture, proteins and cell wall components. Small amount of residue was observed at the end of TG analysis for PHB biomass (untreated PHB) and NaOH-treated PHB. Based on the degradation temperature in the TG curves, temperature of ∼310 °C was selected as pyrolysis temperature of PHB for biocrotonic acid production from NaOH-treated PHB and PHB biomass, while ∼320 °C was selected for chloroform-treated PHB.
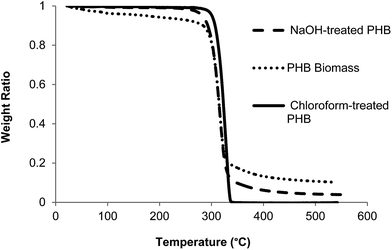 |
| Fig. 2 TG curves of chloroform-treated PHB, NaOH-treated PHB and PHB biomass. | |
Biocrotonic acid was produced in glass tube oven by pyrolyzing the PHB samples. Pyrolyzates were collected and analyzed for their composition by GC-MS (Table 2). Pyrolyzate recovery and crotonic acid recovery yield were calculated gravimetrically according to the method by Mamat et al. (2014).16 The highest pyrolyzate recovery was recorded by chloroform-treated PHB (94%) followed by NaOH-treated PHB (84%) and PHB biomass (70%). Distinct observation was seen in crotonic acid recovery yield whereby NaOH-treated PHB showed the highest crotonic acid recovery yield at 80%, followed by chloroform-treated PHB and PHB biomass at 69% and 65%, respectively. As comparison, the recovery yield of crotonic acid from NaOH-treated PHB obtained herewith was 50% higher than that obtained from petroleum-based crotonic acid.16 Detailed composition of the pyrolyzates were determined by GC-MS, and it was shown that crotonic acid (cis and trans) purity increased to about 89% for NaOH-treated PHB compared with PHB biomass at 62%. Meanwhile, chloroform-treated PHB showed only slight increment (73%) compared with PHB biomass. The composition of pyrolyzates recorded by GC-MS is supported by 1H-NMR spectra (Fig. 3) which clearly show the formation of mainly trans-crotonic acid as shown by methyl signal at ∼1.9 ppm.10,21 Overall mass balance for pyrolysis of PHB biomass, NaOH-treated PHB and chloroform-treated PHB is shown in Fig. 4. Calculation for recovery yield is similar to that reported by Mamat et al. (2014).16
Table 2 Recovery yield and composition of PHB pyrolyzates
|
Amount (%) |
PHB biomass |
Chloroform-treated PHB |
NaOH-treated PHB |
Initial PHB purity |
75 |
99 |
92 |
Pyrolyzate recovery |
70 |
94 |
84 |
CA recovery yield |
65 |
69 |
80 |
![[thin space (1/6-em)]](https://www.rsc.org/images/entities/char_2009.gif) |
GC-MS analysis |
Component |
trans-Crotonic acid |
57.1 ± 4.8 |
69.2 ± 0.5 |
86.6 ± 2.5 |
cis-Crotonic acid |
5.0 ± 0.6 |
3.9 ± 0.4 |
1.9 ± 0.2 |
Oligomer |
37.9 ± 5.4 |
26.9 ± 0.9 |
11.5 ± 2.7 |
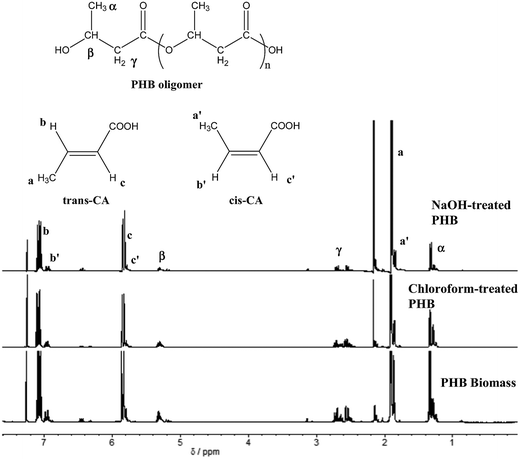 |
| Fig. 3 1H-NMR spectra of PHB pyrolyzates obtained from thermal degradation of PHB biomass, chloroform-treated PHB and NaOH-treated PHB. | |
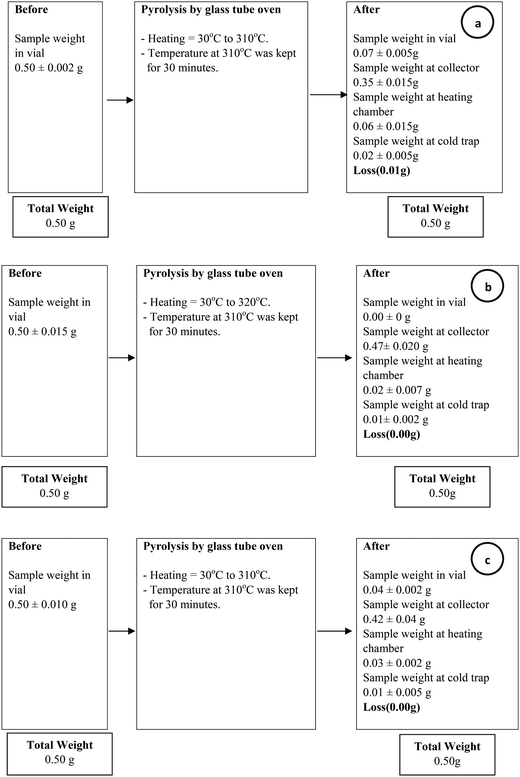 |
| Fig. 4 Mass balance of pyrolysis process for crotonic acid production from (a) PHB biomass, (b) chloroform-treated PHB and (c) NaOH-treated PHB. All data are average of triplicate experiments. | |
Effect of pretreatment method on biocrotonic acid production
Biocrotonic acid composition in pyrolyzate from NaOH-treated PHB was 27% and 16% higher compared to those from untreated and chloroform-treated PHB, respectively (Table 2). This can be explained by several reasons. The first reason could be due to the purity of the starting material, i.e. PHB. In comparison to untreated PHB biomass, NaOH-treated PHB had higher purity at 92% (Table 2). This provided a purer starting material for pyrolysis and hence the pyrolyzate from NaOH-treated PHB contained lesser impurities. This is supported by TEM images of untreated and NaOH-treated PHB biomass (Fig. 5), which showed that NaOH-treated PHB biomass had thinner cell wall. This observation is similar with that of Mohammadi et al. (2012a)22 which reported that thinner bacterial cell wall after NaOH treatment was due to protein and other NPCM released from the cells.
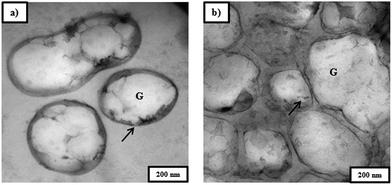 |
| Fig. 5 TEM images of Cupriavidus necator KCTC 2649 cell (a) before and (b) after NaOH pretreatment. Arrow indicates the cell wall of Cupriavidus necator KCTC 2649 cell; G indicates PHA granule. | |
It is interesting to note that even though chloroform-treated PHB had very high purity at 99%, this did not contribute to the high purity of biocrotonic acid produced. Therefore we concluded that the purity of pyrolysis starting material was not the sole reason for the high purity of biocrotonic acid produced. Detailed analysis showed that this observation can be related to thermal degradation pathway of alkaline-treated PHB. It has been reported that when PHB is treated in an alkaline solution at moderate temperature, PHB has high flexibility to form transient structure (6-membered ring state). This allows carboxylate anions which are formed during hydrolysis to accelerate β-elimination chain scission and subsequently producing crotonic acid and PHB with crotonyl-chain end.23 Our group has previously reported that PHB thermal degradation into crotonic acid can be accelerated in the presence of crotonyl chain-end through unzipping reaction.10,24 In order to check the possibility of crotonyl chain-end formation during NaOH pretreatment, FTIR (Fourier transform infrared spectroscopy) analysis was conducted. It was evident from the FTIR spectrum of NaOH-treated PHB sample (Fig. 6) that a strong absorption was found at 1600–1660 cm−1, indicating the C
C stretching. This signal was not observed in chloroform-treated PHB spectrum. From this result, it was confirmed that crotonyl-chain end was present in NaOH-treated PHB and it accelerated the pyrolysis of NaOH-treated PHB into biocrotonic acid. On the other hand, FTIR spectrum of NaOH-treated PHB also showed a more intense signal at 3300 cm−1, indicating the abundance of hydroxyl group forming during hydrolysis. Yu & Marchessault (2000)25 reported that hydrolysis of PHB occurs in NaOH solution and the reaction is heterogeneous and non-random.
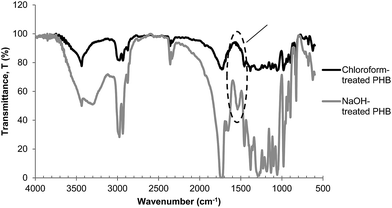 |
| Fig. 6 FTIR spectra of PHB pyrolyzates. | |
Another interesting finding from this research was selective formation of trans-biocrotonic acid. Our group has reported earlier that selective formation of trans-crotonic acid can be accelerated in the presence of metallic compounds.24 The presence of alkali earth compounds such as Na, Ca and Mg assisted in catalytic thermal degradation of PHB.10,24,26,27 In our study, elemental analysis of all the three PHB samples by Atomic Absorption Spectrometry (AAS) showed that NaOH-treated PHB had the highest Na content at 140 ppm, which is 40% higher compared to those in chloroform-treated PHB and PHB biomass. Higher Na content in NaOH-treated sample could be contributed by the replacement of sodium ions at the end of PHB carboxylic chain-end during treatment in alkaline solution. The presence of Na is believed to promote NaOH-treated PHB degradation into trans-crotonic acid. Kim et al. (2008)27 specifically mentioned that Na compound accelerated random chain scission of PHB by cis-elimination to produce crotonic acid.
Additional explanation to the higher biocrotonic acid recovery yield and purity was due to the low molar mass of NaOH-treated PHB. The use of low molar mass PHB may assist in rapid degradation of PHB into crotonic acid. Previous study reported that NaOH pretreatment caused hydrolysis of PHB.25 Mohammadi et al. (2012b)28 also reported a marked reduction in molar mass of PHA after treatment in alkaline solution. PHB molar mass of treated samples is shown in Table 3 and it is seen that NaOH-treated PHB had Mw of 510 kDa compared to chloroform-treated PHB which had Mw of 860 kDa. Since NaOH-treated PHB has lower molar mass, this is another reason for higher biocrotonic acid formation from NaOH-treated PHB compared to chloroform-treated PHB.
Table 3 Molar mass of PHB samplesa
Sample |
Mn (kDa) |
Mw (kDa) |
Mw/Mn |
Mw: weight average molar mass, Mn: number average molar mass, Mw/Mn: polydispersity index. |
PHB biomass |
1100 |
3330 |
1.21 |
Chloroform-treated PHB |
420 |
860 |
2.05 |
NaOH-treated PHB |
220 |
510 |
2.32 |
The overall findings from this research showed that there was interaction between pretreatment method and pyrolysis of PHB for biocrotonic acid production. Despite of the higher purity starting material obtained from chloroform treatment, it did not contribute to the high formation of biocrotonic acid from PHB. Scheme 1 summarizes the differences between chloroform-treated and NaOH-treated PHB, which led to the formation of biocrotonic acid.
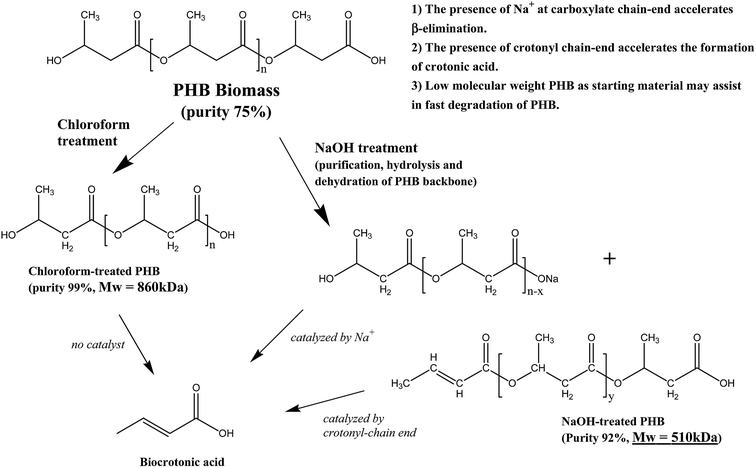 |
| Scheme 1 Pathway of biocrotonic acid production from chloroform-treated and NaOH-treated PHB. | |
Experimental
Oil palm frond
OPF petioles were collected from oil palm plantation located in Universiti Putra Malaysia, Serdang, Selangor. OPF juice was obtained from the OPF petiole according to the method by Zahari et al. (2012).19 OPF juice used in this study had an initial total sugar concentration of 40 g L−1, consisted of 25 g L−1 glucose, 13 g L−1 sucrose and 2 g L−1 fructose.
Microorganism
Cupriavidus necator KCTC 2649 was used in this study for the production of PHB. The bacterium was purchased from Korean Collection for Type Cultures (KCTC). The culture was kept in −80 °C as a frozen stock in 20% glycerol prior to use.
Culture media
The strain was cultivated in nutrient rich medium composed of (per litre of distilled water); nutrient broth (8 g), peptone (5 g), yeast extract (3 g) and glucose (10 g). Mineral Salt Medium (MSM) was used for PHB production, composed of (per litre of distilled water); carbon source (20 g), KH2PO4 (5 g), K2HPO4 (1.5 g), (NH4)2SO4 (1.0 g) and MgSO4 (0.2 g). One mL of trace element was added after sterile filtration.29 OPF juice was used as carbon source while mixture of synthetic sugars (glucose, sucrose and fructose) having similar concentration with OPF juice was used as control carbon source. In order to study effect of OPF juice heating on PHB production, heat-sterilized OPF juice was used and compared with filter-sterilized OPF juice.
Biosynthesis of PHB in 20 L bioreactor
PHB production was carried out in 20 L bioreactor with working volume of 15 L through fed-batch fermentation. The culture was incubated for 72 h at 34 °C with agitation speed of 200 rpm. pH was controlled at pH 6.8 with 10% H2SO4 solution and 25% NH4OH solution, while dissolved oxygen level was maintained at 20% saturation throughout fermentation by using cascade mode and supplied with air at 1.0 vvm.29 Sugar concentration was measured by using DNS method. Cells containing PHB were harvested by centrifuge with 10
000×g for 10 min at 4 °C in a Sorvall Legend RT+ Centrifuge and lyophilized using freeze-dryer. The cells were then ground and stored at −20 °C prior to storage.22
Pretreatment of cell containing PHB by low concentration sodium hydroxide (NaOH)
About 20 g L−1 of dry cell was treated with 20 mL of 0.05 M NaOH and incubated at 4 °C for 3 h with no agitation. After the NaOH pretreatment, biomass pellet containing PHA was recovered by centrifugation at 15
000×g for 20 min at 4 °C in a Sorvall Legend RT+ Centrifuge. Purification process was carried out by adding 1% (v/v) of ethanol (96%, Fisher, analytical grade) to the pellet and incubated at 30 °C with an agitation speed of 200 rpm for 3 h. The washed pellet was then centrifuged at 15
000×g for 10 min at 4 °C. Finally, the pellet was harvested and resuspended in distilled water for further washing and centrifuged at 15
000×g for 10 min at 4 °C prior to freeze-drying.22 NaOH-treated PHB cells were then used for the production of biocrotonic acid. PHB extracted by chloroform was used as control sample.
Biocrotonic acid production in glass tube oven
Dynamic pyrolysis in thermogravimetric analyzer (TGA, Perkin Elmer, USA) was performed to estimate the PHB degradation temperature.16 Biocrotonic acid production was conducted in a glass tube oven (Shibata GTO-350D) by pyrolyzing about 500 mg of PHB samples16 according to the following steps: (i) heated the sample from room temperature to 200 °C, hold at 200 °C for 30 min, (ii) heated the sample from 200–310 °C, and hold at 310 °C for 30 min. Vaporized pyrolyzates were condensed in a cold trap and collected by dissolving in acetone and left to dry into white crystals. The obtained product was analyzed by 1H NMR and GCMS for characterization of the product. Three types of PHB samples were used in this experiment: untreated PHB cells, NaOH-treated PHB and chloroform-treated PHB (control sample). Effect of NaOH pretreatment on recovery and purity of biocrotonic acid was also determined by 1H NMR and GCMS.
Analytical procedures
Atomic absorption spectrometry (AAS). Sodium residue in PHB was quantified using atomic absorption spectrophotometer (AAS). PHB sample was degraded by 25% ammonia solution followed by dissolving in 1 M HCL and then measured by AAS.10
Gas chromatograph and mass spectrometry (GC-MS). Pyrolyzates from isothermal pyrolysis was analyzed using Perkin Elmer Clarus 600 GC-MS. Highly pure helium gas was used as a carrier gas at a constant flow rate of 6 mL min−1. Pyrolyzates were dissolved in chloroform prior to analysis and were introduced into MS through 5% phenyl polysilphenylene-siloxane column; 30 m × 0.25 mm I.D × 0.25 μm film thickness (BPX-5, SGE analytical science). The ion source temperature used for MS was 200 °C. The data was taken from 3 min until 32 min.16
Gas chromatography (GC). PHB content and composition in lyophilized cell was determined using GC. Approximately 20 mg of lyophilized cells were subjected to methanolysis in the presence of methanol and sulfuric acid [85
:
15 (% v/v)]. Organic layer containing reaction products was separated, dried over sodium sulphite (Na2SO), and analyzed by GC according to the standard method, with benzoic acid as an internal standard.30
Gel permeation chromatography (GPC). Molar mass of the samples was measured by gel permeation chromatography (GPC) on TOSOH HLC-8120 GPC system with a refractive index (RI) detector at 40 °C using TOSOH TSK gel Super HM-M column and chloroform eluent (0.6 mL min−1). Approximately, 12 mg of the sample was dissolved in 2 mL chloroform and the solution was filtered through a membrane filter with 0.45 mm pore size.10
Proton-NMR spectrometry (1H-NMR). Chemical composition of pyrolyzate was also determined by 1H-NMR to support the result obtained from GC-MS. The spectrum was recorded on a JEOL NMR 500 MHz system. Chloroform-d (CDCl3) was used as solvent. Chemical shifts were reported as δ values (ppm) relative to internal tetramethylsilane (TMS) in CDCl3 unless otherwise noted. The expected 1H-NMR chemical shifts were predicted using a ChemNMR program in a CS ChemDraw Ultra version 6.0.10,11
Sample preparation for TEM analysis
Freeze-dried cells were pre-fixed with 2.5% glutaraldehyde and washed in sodium phosphate buffer. Then, 1% of osmium tetroxide was used for post-fixation of cell at 4 °C for 2 h. Similar buffer was used to wash post-fixed cells and this was done three times. Cells were later dehydrated with graded acetone series. Infiltration of cells was made with propylene oxide and resin mixtures and after that 100% resin was used for cell embedding. Ultra-thin sections of the embedded sample with an ultramicrotome were completed in epoxy resin. Finally, it was stained with 2% uranyl acetate and lead citrate respectively, for 10 min to develop contrast between the different polymer phases. The obtained specimens were analyzed by TEM (Technai G2 20S TwinTEM).
Conclusion
Our study shows that biocrotonic acid formation from PHB was greatly affected by PHB pretreatment method prior to pyrolysis. NaOH-treated PHB showed high purity and recovery yield at 89 and 80%, respectively. It is interesting to note that mild NaOH pretreatment assisted in high thermal conversion of PHB into biocrotonic acid by the creation of crotonyl chain- and Na-binding carboxyl terminal-ends; of which both accelerated the formation of crotonic acid. Reduced molar mass of PHB after NaOH treatment also contributed to the acceleration of PHB conversion into crotonic acid. Overall, improved biocrotonic acid production with high purity biocrotonic acid is an advantage for industrial production of crotonic acid from renewable resource.
Acknowledgements
The authors would like to thank Universiti Putra Malaysia (UPM) for the provision of scholarship (GRF) to the first author. Authors are greatly appreciated with the assistance from technical staff from UPM and Universiti Malaysia Pahang (UMP). Authors also would like to acknowledge Che Mohd Hakiman Che Maail for his assistance in this research. This project was funded by Research University Grant Scheme (RUGS) no. 9389100 from Universiti Putra Malaysia.
References
- M. Fache, E. Darroman, V. Besse, R. Auvergne, S. Caillol and B. Boutevin, Green Chem., 2014, 16, 1987 RSC.
- M. N. Somleva, O. P. Peoples and K. D. Snell, Plant Biotechnol. J., 2013, 11, 233–252 CrossRef CAS PubMed.
- W. Schmid, Joachim and K. Mauch, European Patent, PCT/EP2008/007827, 2008.
- S. Tomizawa, M. Yoshioka, K. Ushimaru and T. Tsuge, Polym. J., 2012, 44, 982–985 CrossRef CAS.
- P. Salmon, J. Wilson and N. Connors, J. Ferment. Bioeng., 1998, 86, 261–265 CrossRef.
- P. H. Larsson, A. Eklund, S. G. Johansson and K. Larsson, J. Immunol. Methods, 1997, 210, 41–49 CrossRef CAS.
- E. Karadağ, O. B. Uzüm, D. Saraydin and O. Güven, Int. J. Pharm., 2005, 301, 102–111 CrossRef PubMed.
- S. P. Singh, A. K. Singh and B. Singh, J. Mol. Catal. A: Chem., 2007, 266, 226–232 CrossRef PubMed.
- A. N. Babayig and M. Pulat, J. Appl. Polym. Sci., 2001, 2690–2695 Search PubMed.
- H. Ariffin, H. Nishida, Y. Shirai and M. A. Hassan, Polym. Degrad. Stab., 2008, 93, 1433–1439 CrossRef CAS PubMed.
- H. Ariffin, H. Nishida, Y. Shirai and M. Ali, Polym. Degrad. Stab., 2010, 95, 1375–1381 CrossRef CAS PubMed.
- S. Bassaid, M. Chaib, A. Bouguelia and M. Trari, React. Funct. Polym., 2008, 68, 483–491 CrossRef CAS PubMed.
- J. Van Walsem, E. Anderson, J. Licata, K. A. Sparks, C. Mirley and S. M. Sivasubramanian, US Pat. Appl. 20120315681A1, 2012.
- R. P. Schulz, Crotonaldehyde and Crotonic Acid, Ullmann's Encyclopedia of Industrial Chemistry, 2002 Search PubMed.
- H. J. Arpe and K. Weissermel, Industrial Organic Chemistry, VCH, New York, 3rd edn, 1997 Search PubMed.
- M. R. Z. Mamat, H. Ariffin, M. A. Hassan and M. A. K. M. Zahari, J. Cleaner Prod., 2014, 83, 463–472 CrossRef CAS PubMed.
- S. Rittner, H. Gortz and K. Riedel, US Pat. 4,918,225, 1990.
- D. Koch and G. Meurer, Eur. Pat. Appl. 2522377A1, 2012.
- M. A. K. M. Zahari, M. Khushairi, M. Rafein, H. Ariffin, M. Noriznan, J. Salihon, Y. Shirai and M. Ali, Bioresour. Technol., 2012, 110, 566–571 CrossRef CAS PubMed.
- C. M. H. C. Maail, H. Ariffin, M. A. Hassan, U. K. M. Shah and Y. Shirai, BioMed Res. Int., 2014, 2014, 465270 Search PubMed.
- H. Morikawa and R. H. Marchessault, Can. J. Chem., 1981, 59, 2306–2313 CrossRef CAS.
- M. Mohammadi, M. A. Hassan, L.-Y. Phang, H. Ariffin, Y. Shirai and Y. Ando, Biotechnol. Lett., 2012, 34, 253–259 CrossRef CAS PubMed.
- J. Yu, D. Plackett and L. X. L. Chen, Polym. Degrad. Stab., 2005, 89, 289–299 CrossRef CAS PubMed.
- H. Ariffin, H. Nishida, Y. Shirai and M. A. Hassan, Polym. Degrad. Stab., 2010, 95, 1375–1381 CrossRef CAS PubMed.
- G. Yu and R. H. Marchessault, Polymer, 2000, 41, 1087–1098 CrossRef CAS.
- K. J. Kim, Y. Doi and H. Abe, Polym. Degrad. Stab., 2006, 91, 769–777 CrossRef CAS PubMed.
- K. J. Kim, Y. Doi and H. Abe, Polym. Degrad. Stab., 2008, 93, 776–785 CrossRef CAS PubMed.
- M. Mohammadi, M. A. Hassan, L.-Y. Phang, Y. Shirai, H. C. Man and H. Ariffin, J. Cleaner Prod., 2012, 37, 353–360 CrossRef CAS PubMed.
- M. A. K. Mohd Zahari, H. Ariffin, M. N. Mokhtar, J. Salihon, Y. Shirai and M. A. Hassan, J. Biomed. Biotechnol., 2012, 2012, 125865 Search PubMed.
- G. Braunegg, B. Sonnleitner and R. M. Lafferty, Eur. J. Appl. Microbiol. Biotechnol., 1978, 29–37 CrossRef CAS.
|
This journal is © The Royal Society of Chemistry 2015 |