DOI:
10.1039/C5RA01144B
(Paper)
RSC Adv., 2015,
5, 24231-24238
A glucosyl triblock copolymer: synthesis and its injectable thermo- and pH-responsive behaviours
Received
20th January 2015
, Accepted 26th February 2015
First published on 2nd March 2015
Abstract
A new glucosyl triblock copolymer, poly(acryloyl glucofuranose)-block-poly(N-isopropyl acrylamide)-block-poly(acrylic acid) (PAG-b-PNIPAM-b-PAA, i.e., PGNA), was first synthesized from 3-O-acryloyl-1,2;5,6-di-O-isopropylidene-α-D-(−)-glucofuranose (ADIPG), tert-butyl acrylate (tBA) and N-isopropyl acrylamide (NIPAM) in the presence of the initiator 2,2-azoisobutyronitrile (AIBN) by sequential reversible addition–fragmentation chain transfer (RAFT) polymerization. In this RAFT process, 2,2′-thiocarbonylbis(sulfanediyl)bis(2-methylpropanoic acid) (CMP) was synthesized and used as a chain transfer agent. PGNA and the reaction intermediates were characterized by FT-IR, HNMR and GPC. When heated, the aqueous PGNA copolymer solution exhibits good sol–gel phase transition behaviours. Meanwhile, the formed hydrogels are also sensitive to the pH. When the copolymer solution is injected into a living Sprague Dawley (SD) rat, an in situ gel is formed within 3 minutes. By using acetylsalicylic acid (aspirin) as a model, drug release tests show that the maximum releasing value is approximately 76% under physiological conditions (pH 7.4, 37 °C). The synthesized triblock copolymer shows no apparent cytotoxicity towards L929 fibroblast cells according to an in vitro cytotoxicity test (MTT assay).
1. Introduction
In recent decades, smart hydrogels have received more and more research attention for their extensive applications in many fields such as industrial,1–4 agricultural,5–9 biological10–14 and materials15–19 fields, and multiple intelligent hydrogels have attracted especially great interest for their ability to adapt to complex environments. In particular, as drug carriers, hydrogels have gained much attention for their great application prospects and value. Under the premise that hydrogels are going to be used in the human body, the parameters of the physical environment must be considered, such as body temperature and humoral pH values, in drug delivery systems. In other words, a drug carrier should make full use of the difference between the external and body temperatures, and the gel pH should be controlled to meet the physical pH value. Poly(N-isopropyl acrylamide) (PNIPAM) is one of the most widely studied thermoresponsive monomers with a phase transition temperature of approximately 32 °C in water, which is close to the human physiological temperature.20,21 Poly(acrylic acid) (PAA) is one of the typical pH sensitive monomers that can exhibit swelling–deswelling phenomenon due to the protonation–deprotonation of its carboxyl groups in aqueous solution.22 In addition, the linkage of NIPAM with AA usually produces a dual sensitive hydrogel.23–26 To date, the reported dual sensitive hydrogels are random structures prepared from mostly petrochemical monomers, and their biocompatibility needs to be improved. These studies give full consideration to problems such as the temperature and pH sensitivity, but there is not sufficient attention to the biocompatibility of the hydrogels. Our group previously reported a diblock glucosyl thermosensitive hydrogel and also found that its biocompatibility was greatly improved after the introduction of a glycosyl group.27
To the best of our knowledge, there are no reports of a sol–gel system based on triblock copolymers that contain glucofuranose, N-isopropyl acrylamide, and acrylic acid. Herein, we designed and synthesized a triblock double sensitive smart hydrogel, poly(3-O-acryloyl-glucofuranose)-block-poly(N-isopropyl acrylamide)-block-poly(acrylic acid) (PAG-b-PNIPAM-b-PAA, i.e., PGNA). The triblock copolymers were synthesized by sequential reversible addition–fragmentation transfer (RAFT) polymerization28,29 with PNIPAM entities as the thermosensitive units, PAA entities as the pH sensitive units and glucosyl units to enhance the biocompatibility. The advantages of the RAFT process are that excellent control of the molecular structures and weights, a narrow molecular weight distribution and dual sensitive properties would be achieved in the triblock copolymer. This strategy can also avoid some limitations, such as a high polydispersity (PDI) and unwanted random or branched polymerization. The resulting copolymers were also characterized by FT-IR, HNMR and GPC. Furthermore, the properties of PAG-b-PNIPAM-b-PAA were investigated, such as the sol–gel–syneresis phase transition behaviour as well as the thermo- and pH-responsive behaviours. The copolymer proved to be injectable and have a low cytotoxicity via a SD rat experiment and an in vitro cytotoxicity test, and its drug release behaviour was also investigated using acetylsalicylic acid (aspirin) as a model.
2. Materials and experiments
2.1 Materials
The inhibitor in monomer tert-butyl acrylate (tBA, Aladdin, 99%) was washed with NaOH and distilled under a reduced pressure. N-Isopropyl acrylamide (NIPAM, Tokyo Kasei Kogyo Co., Ltd, 98%) was recrystallised three times from hexane and dried under vacuum. 3-O-Acryloyl-1,2;5,6-di-O-isopropylidene-α-D-(−)-glucofuranose (ADIPG) was prepared via the reaction between allyl bromide and 1,2;5,6-di-O-isopropylidene-α-D-(−)-glucofuranose (from Aladdin).30 2,2′-Thiocarbonylbis(sulfanediyl)bis(2-methylpropanoic acid) (CMP) was synthesized according to a literature.31 The initiator 2,2-azoisobutyronitrile (AIBN, Shanghai Reagent Co., China, 99%) was recrystallized from ethanol and dried with vacuum drying. 1,4-Dioxane (AR, Shanghai Richjoint Chemical Reagents Co., Ltd) was refluxed for 1–2 hours with NaH and Na using diphenyl ketone as the indicator, and then it was distilled under normal pressure prior to each use. All of the other reagents were of analytical grade and used as received.
2.2 Synthesis of the PAG-b-PNIPAM-b-PAA triblock copolymer
The thermo- and pH-responsive triblock copolymer was synthesized from the following step by step synthesis procedure: (1) synthesis of macromolecular chain transfer agent PtBA–CMP by RAFT polymerization; (2) polymerization of the diblock copolymer PNIPAM-b-PtBA; (3) polymerization of the triblock copolymer PAIDPG-b-PNIPAM-b-PtBA; (4) desulfurization of PAIDPG–PNIPAM–PtBA; and (5) hydrolysis to obtain PAG–PNIPAM–PAA. The reaction scheme is shown in Scheme 1.
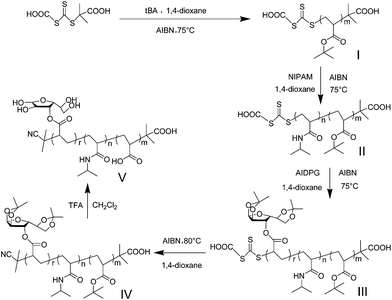 |
| Scheme 1 Synthesis of PAG-b-PNIPAM-b-PAA (PGNA) triblock copolymers. | |
2.2.1 Synthesis of PtBA–CMP (I). PtBA–CMP was prepared from tBA in the presence of the initiator AIBN by RAFT polymerization and used CMP as a chain transfer agent. A typical run was as follows: a 50 mL Schlenk flask was charged with tBA (10.24 g, 0.08 mol), CMP (451.2 mg, 1.6 mmol), AIBN (26.2 mg, 0.16 mmol), and 1,4-dioxane (11 mL). The reaction system was degassed by four freeze–pump–thaw cycles, and the reaction was continued at 75 °C for 3 h. The reaction was terminated by immersing the flask into ice-salt baths. The product was precipitated twice into a 15-fold excess solution of water and MeOH (1
:
1, v/v). After drying in a vacuum oven overnight at 35 °C, the macro-RAFT agent PtBA–CMP (I) was obtained.
2.2.2 Polymerization of PNIPAM-b-PtBA (II). With AIBN as the initiator, PtBA–CMP as the macro-RAFT agent, diblock copolymer PNIPAM-b-PtBA was synthesized from NIPAM. The typical procedure was showed as follows: a 50 mL Schlenk flask was charged with PtBA–CMP (2.3 g, 1.25 mmol) as the macro-RAFT agent, NIPAM (14.0 g, 123.9 mmol), AIBN (10.2 mg, 0.06 mmol), and 1,4-dioxane (22 mL). The reaction system was degassed by four freeze–pump–thaw cycles, and the reaction was continued at 75 °C for 12 h. The reaction was terminated by immersing the flask into ice-salt baths. The product was precipitated twice into a water and MeOH solution (1
:
1 v/v). After drying in a vacuum oven overnight at 35 °C, the diblock copolymer PNIPAM-b-PtBA (II) was obtained.
2.2.3 Polymerization of PAIDPG-b-PNIPAM-b-PtBA (III). Triblock copolymer PAIDPG-b-PNIPAM-b-PtBA was prepared by reacting AIDPG with PNIPAM-b-PtBA in the presence of AIBN as an initiator. A typical procedure was as follows: a 100 mL Schlenk flask was charged with PNIPAM–PtBA (5.4 g, 0.33 mmol) as the macro-RAFT agent, ADIPG (10.0 g, 31.8 mmol), and 1,4-dioxane (25 mL). After complete dissolution, AIBN (2.6 mg, 0.016 mmol) was added. The reaction system was degassed by four freeze–pump–thaw cycles, and the reaction was continued at 75 °C for 16 h. The reaction was terminated by immersing the flask into ice-salt baths. The product was precipitated twice into a solution of MeOH and water (1
:
1, v/v). After drying in a vacuum oven overnight at 35 °C, the triblock copolymer PAIDPG-b-PNIPAM-b-PtBA (III) was obtained.
2.2.4 Synthesis of NC(Me2)C-PAIDPG-b-PNIPAM-b-PtBA-C(Me2)COOH (IV). In a typical procedure, a 50 mL Schlenk flask was charged with copolymer III (2.0 g, 0.01 mmol), AIBN (0.93 g, 5.67 mmol), and 1,4-dioxane (15 mL). After degassing by three freeze–pump–thaw cycles, the reaction was continued at 80 °C for 20 h. The solution was concentrated by rotating evaporation, and then the concentrated solution was precipitated twice into a 15-fold excess of n-hexane. After being dried in a vacuum oven overnight at the normal temperature, a faint yellow powder NC(Me2)C-PAIDPG-b-PNIPAM-b-PtBA-C(Me2)COOH (IV) was collected.
2.3 Characterization of the copolymers
The FT-IR spectra were obtained on a Nicolet Avatar 370 Fourier transform spectrometer at room temperature, with the samples being prepared by the KBr squash method. 1H NMR spectra of the block copolymers were recorded at room temperature on a Varian Gemini 2000I 400 MHz NMR spectrometer by using deuterium chloroform or dimethyl sulfoxide as the solvents. Chemical shifts (δ) are given in ppm using tetramethylsilane (TMS) as an internal reference. The number averaged molecular weights and the polydispersity indices (PDIs) of copolymers (I)–(V) were measured by GPC with THF as the eluent at a flow rate of 1.00 mL min−1, and a sample concentration of 0.10 mg mL−1 was used. The polyethylene glycol in a molecular weight range of 600–40
000 Da were used as the molecular weight standards.
2.4 Measurement of the sol–gel phase transition
By using a test method based on inversion, the sol–gel phase transition of the PGNA triblock copolymer in aqueous solution was investigated.32,33 The copolymer was dissolved in a phosphate buffered saline (PBS) solution in 2 mL (10 mm diameter) vials at a given concentration and stabilized at 4 °C for 3 h. Then, the vials were placed in a water-bath and heated slowly at a rate of 0.1 °C min−1 from 15 °C to the syneresis temperature. The transition temperatures were determined by observing whether there was flow when the vial was horizontally inverted. To reach equilibrium, each step was maintained for 10 min. Once the vial was inverted without flow for 30 s, the sample was considered to be a gel. With a further increase in temperature, phase separation happened, and the corresponding temperature was recorded as the syneresis temperature. Each point is an average of three measurements.
2.5 In vivo experiments
To confirm the injectability of the synthetic triblock copolymer hydrogel, Sprague-Dawley (SD) rats were used for in vivo experiments. The PGNA copolymer was dissolved in physiological saline at a concentration of 10 wt% and stored at 4 °C for 4 h so it could completely dissolve. Then, the copolymer solution was subcutaneously injected into a healthy SD rat by a syringe with a 20 gauge needle. After 3 min, the injection site was sacrificed to observe the morphology of the gel and the in situ gel formation ability of the triblock copolymer in physiological conditions.
2.6 In vitro release of aspirin from the PGNA hydrogel
Acetylsalicylic acid (aspirin) was used as a model drug to investigate the release property of the hydrogel. First, the aspirin-loaded hydrogels were prepared.34 The as-prepared PGNA copolymer was equilibrated in an absolute ethanol solution with aspirin for overnight loading in darkness. Ethanol in the hydrogels was evaporated in a vacuum oven and the aspirin-loaded dry hydrogels were obtained. The amount of aspirin in the dry gels was 1.5%. Subsequently, the drug-loaded hydrogels (0.1 g) were immersed in a volume of pH 7.4 buffer solutions in a shaking water bath at 37 °C. Then, 3 mL solution was collected at 1 h intervals and the buffer solution was replaced periodically to maintain a constant volume. The aspirin content was recorded by using a UV spectrophotometer at 296 nm. The in vitro release tests were conducted in triplicate. The concentration of the drug in the buffer solutions was calculated from the following calibrated standard curves: y = 0.2521 + 0.0029x (r = 0.9992).35
The cumulative release ratio of the aspirin was calculated from the following equation:35
where
Wdt is the weight of released drug at time
t and
W∞ is the total weight of loaded aspirin in the hydrogel.
2.7 In vitro cytotoxicity test
The in vitro cytotoxicity of the triblock copolymer hydrogel was evaluated by the direct contact method. L929 cells were placed in 96-well plates at a seeding density of 1 × 104 cells per well and incubated in a humidified incubator (37 °C, 5% CO2) for 12 h. The cells were treated with different concentrations of prepared sterile triblock copolymer (5.0, 10.0, 15.0, and 20.0 wt%) and incubated at 37 °C for 24 h. The metabolic activity of the cells was measured with a 3-(4,5-dimethylthiazol-2-yl)-2,5-diphenyltetrazolium bromide (MTT) assay using well-grown passage L929 mouse fibroblasts under the standard ISO 10993.5-1999. Then, copolymer-free wells cultured under the same conditions were used as controls. Briefly, 20 μL of MTT solution (5 mg mL−1) was added to each well and incubated for 4 h. Then, the MTT-containing culture medium was replaced with 150 μL of DMSO. After 15 min, the absorbances of the samples were measured at 490 nm in a microplate reader. The cytotoxicity is defined as the relative viability, with culture medium containing no triblock copolymer representing 100%.
3. Results and discussion
3.1 Characterization of the copolymers
FT-IR spectra of the intermediates and the desired copolymer are shown in Fig. 1. In the spectrum of Fig. 1(I), the typical band at 1730 cm−1 is ascribed to the carbonyl group of the ester group. As shown in Fig. 1(II), after copolymerization with NIPAM, another strong absorption peak at 1652 cm−1 appears, which can be attributed to the carbonyl group in the amide group. Furthermore, the vibration of the N–H of the amide group exhibits an obvious peak at 3437 cm−1. Fig. 1(III) is the spectrum of the triblock copolymer. In this structure, the protected glycosyl monomer was introduced, which results in a few absorption peaks of ether groups at 1024, 1077 and 1218 cm−1. The spectrum of the desired copolymer is shown in Fig. 1(V). From this spectrum, it can be seen that the absorption peak at 3453 cm−1 was greatly enhanced, which indicated that the hydrolysis of the protected glycosyl group was successfully carried out.
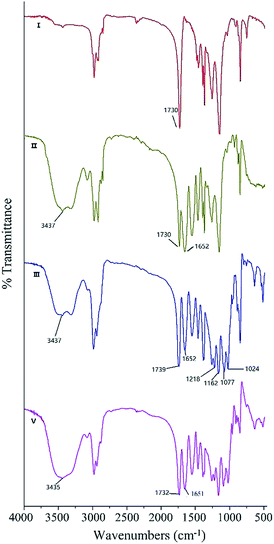 |
| Fig. 1 FT-IR spectra of macro-RAFT agent I, diblock copolymer II, triblock copolymer III and triblock copolymer V. | |
Fig. 2 is the GPC curves of macro-RAFT agent I, diblock copolymer II, and triblock copolymer III. The well-controlled PtBA–CMP (Mn = 1806 Da, PDI = 1.078) was successfully obtained as evident by its sharp and symmetric shape of GPC elution peak (Fig. 2(I)). The diblock copolymer PNIPAM-b-PtBA was synthesized by the following RAFT polymerization of NIPAM using PtBA–CMP as the macro-RFAT agent. As shown in Fig. 2(II), the narrow molecular weight distribution (Mn = 16
396 Da, PDI = 1.160) and an unimodal and symmetrical GPC curve indicated that the PtBA–CMP was an effective macro-RAFT agent for RAFT polymerization of NIPAM. The triblock copolymer PAIDPG-b-PNIPAM-b-PtBA was synthesized by the third step RAFT polymerization of AIDPG using PNIPAM-b-PtBA as the macro-RFAT agent. The narrow molecular weight distribution (Mn = 19
082 Da, PDI = 1.272) and an unimodal and symmetrical GPC curve in Fig. 2(III) indicated that the PNIPAM-b-PtBA was an effective macro-RAFT agent for RAFT polymerization of AIDPG. As a result of the second RAFT step, the molecular weight is increased to 16
396 Da, and after the second RAFT step, the increase in the molecular weight by 2686 proved that the triblock copolymer was prepared successfully.
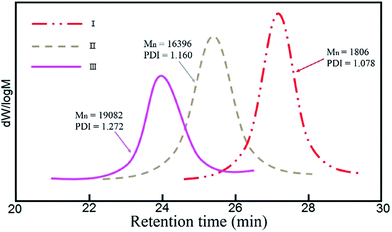 |
| Fig. 2 GPC traces of macro-RAFT agent I (dash dot dot line), diblock copolymer II (dashed line) and triblock copolymer III (solid line) (PGNA2). | |
The chemical structures of these copolymers were also characterized by 1H NMR (Fig. 3). The spectrum of macro-RAFT agent I exhibits the typical peaks at 1.99, 1.23 and 1.39 ppm, which are ascribed to CH–C
O, methylene and t-butyl groups, respectively. Compared with the spectrum of I, the spectrum of II displays new peaks at 3.84 and 1.04 ppm resulting from –NH and isopropyl groups, indicating the formation of a diblock copolymer. After the introduction of the protected glycosyl monomer, the spectrum of III exhibits typical peaks at 1.25–1.43 ppm, which are attributed to the protons of the isopropylidene groups of glycosyl ring. Furthermore, a series of new peaks at 4.03–5.87 ppm ascribed to the glycosyl ring appeared, which also proved that the glycosyl monomer was copolymerized into the triblock copolymer. In the spectrum of V, it is obvious that the isopropyl peaks at 1.25 and 1.31 ppm disappeared in PGNA2. Meanwhile, carboxyl peaks at 10.76 ppm appeared, which demonstrates that the desired copolymer was formed after the hydrolysis reaction.
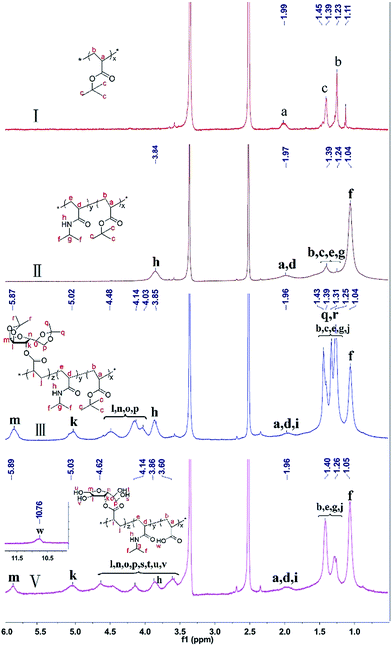 |
| Fig. 3 1H NMR spectra of macro-RAFT agent I, diblock copolymer II, triblock copolymer III and triblock copolymer V (PGNA2). | |
3.2 Sol–gel–syneresis phase transition behaviour
Fig. 4 shows three photographs of the in vitro sol–gel–syneresis phase transition of a 15 wt% PGNA2 copolymer hydrogel under different temperatures at a pH of 7.4. It was found that PGNA2 in aqueous solution exists in a solution state at 20 °C. At this temperature, all three chain segments of PNIPAM, PAA, and PAG are hydrophilic and solubilizable, as shown in Fig. 4(a). At 37 °C, the PGNA2 copolymer forms a gel in aqueous solution (Fig. 4(b)). Because the hydrogen bonds between thermosensitive units and water molecules are destroyed, the hydrophilic ability of the copolymer is reduced. Thus, it separates from water. When the temperature is raised to 52 °C, as a result of syneresis, the copolymer as a whole separates from solution, as shown in Fig. 4(c). This sol–gel–syneresis transition is a reversible process.
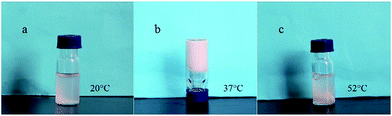 |
| Fig. 4 In vitro sol–gel–syneresis phase transition photographs of 15 wt% PGNA2 hydrogel at a pH of 7.4: (a) solution state (20 °C), (b) gel state (37 °C) and (c) syneresis state (52 °C). | |
The temperature-dependence of the sol–gel–syneresis phase transition diagram of PGNA in aqueous solution was investigated by a method based on inverting the test tubes at a pH of 7.4 (Fig. 5). To investigate the effect of the polymer molecular weight on the phase transition behaviours, a series of triblock copolymers were synthesized (Table 1). As we previously reported,27 PAG similarly to POAG mainly acts as a biocompatible segment, and the PNIPAM block acts as the temperature sensitive block. It should be mentioned that the LCST decreases and the gel window increases as the composition of PNIPAM is increased, which is agreement with previous studies.36,37 Due to the hydrophilic amide and PAG as well as the weak hydrophobic interaction between the PNIPAM blocks, the synthesized triblock copolymer was fully soluble at low temperature (below the LCST) for a long time. As shown in Fig. 5, the molecular weights have a great effect on the sol–gel–syneresis phase transition behaviour. At the same concentration, the larger the molecular weight of the polymer, the stronger its ability to form a gel. When the molecular weight is approximately 24
728 Da or above, a gel can be formed at low temperatures, making it not suitable for use as an injectable drug. However, when the molecular weight is approximately 12
147 Da or below, PGNA could not readily form a hydrogel at low concentrations. Furthermore, the syneresis temperature of PGNA1 is approximately 30–40 °C; consequently, it is not suitable for use as a slow release drug carrier. By comprehensive consideration, a molecular weight of approximately 18
614 Da (PGNA2) is most appropriate among the three molecular weights tested.
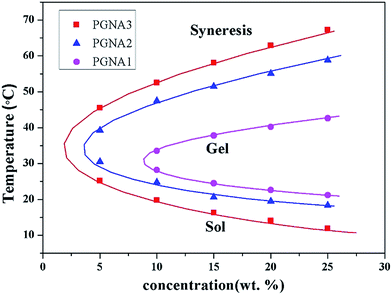 |
| Fig. 5 Sol–gel–syneresis phase transition diagram of the PGNA hydrogel with different copolymer molecular weights at a pH of 7.4. | |
The influence of the pH on the sol–gel phase transition was also studied. Fig. 6 shows the sol–gel phase transition diagram of PGNA2 in aqueous solutions with different concentrations under various pH values. The aqueous PGNA2 copolymer was a solution at every tested pH value at low temperature because of the complete protonation of its functional groups (hydroxy, amide and carboxyl groups included in the PAG, PNIPAM, PAA segments), resulting in the triblock copolymers being hydrophilic. The gel region shifted to higher temperatures with increasing pH values, suggesting that the polymer is sensitive to pH, which results from the ionization of the carboxyl groups. With the increasing amount of –COO−, the solubility of the copolymer increased in solution and consequently, made it more difficult for it to form a gel. Moreover, the copolymer concentration has an effect on the formation of a gel. At the same pH value, the gel region moved to a lower temperature with increasing copolymer concentrations.
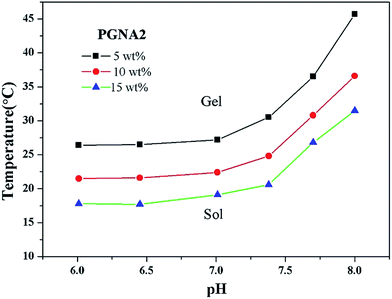 |
| Fig. 6 Sol–gel phase transition diagram of PGNA2 hydrogels with different concentrations and pH values. | |
As shown in Fig. 5 and 6, at a pH of 7.4, the LCST and syneresis temperatures of 5 wt% PGNA2 copolymer hydrogel are 30.5 °C and 39.2 °C, respectively. Meanwhile, these temperatures were varied to 20.6 °C and 51.5 °C when 15 wt% copolymer hydrogel was used. However, the two temperatures mentioned above are not feasible at the physiological temperature. Notably, when the concentration is 10 wt%, the LCST and syneresis temperatures are 24.8 °C and 47.5 °C, respectively. By comprehensive consideration, the PG hydrogel (e.g., pH 7.4, 10 wt%) is more suitable for use in physiological conditions. Therefore, we choose PGNA2 as the sample for the subsequent properties test.
3.3 In vivo gel formation
The injectability and in vivo gelation behaviour of PGNA triblock copolymer hydrogels were examined by subcutaneous injection. A triblock copolymer solution (10 wt%, PGNA2, 1 mL) was injected into the dorsal surface of a SD rat using a syringe needle (20G). After 3 min, the rat was sacrificed, and the morphology of the gel was observed. As shown in Fig. 7, a white gel formed quickly in situ due to the changes in the pH and temperature under the rat's physiological conditions. This result indicates that the PGNA copolymer solution can be used as an injectable hydrogel system, and it can be easily injected into the body and rapidly form a gel in situ.
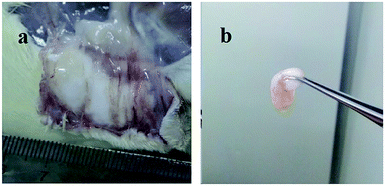 |
| Fig. 7 (a) In vivo gel morphology 3 min after the subcutaneous injection of 1 mL of a 10 wt% PGNA2 solution into a SD rat; and (b) the gel after tissue removal. | |
3.4 In vitro release of aspirin
Aspirin was employed as a model drug to study the release behaviour of PGNA copolymer hydrogels in a shaking water bath at a shaking speed of 40 rpm at 37 °C. Fig. 8 shows the in vitro release profiles of aspirin from the hydrogel at 37 °C. In the initial 2 h, the cumulative release of aspirin increased from 0 to 38.3% with a higher release rate from the hydrogels. Then, the cumulative amount increased to 67.2% in 6 h. Although the cumulative amount of the drug released increased, the cumulative release rate decreased with increasing amounts of time. After approximately 8 h, its release amounts tended to smooth out, and the maximum release was approximately 76%. As evident in Fig. 8, the sustained release was maintained for 7–8 h. There is still over 20% aspirin in the hydrogel due to tenacious adsorption. This result suggests that the triblock copolymer hydrogel could satisfy the need for a controllable release of a drug under physiological conditions.
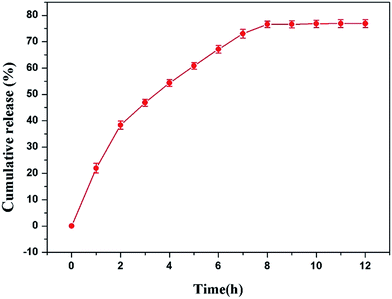 |
| Fig. 8 In vitro release of aspirin from PGNA2 hydrogel in pH 7.4 buffer solution at 37 °C. | |
3.5 In vitro cytotoxicity
The cytotoxicity of the PGNA copolymer is a critical property because it was designed for drug delivery applications. Herein, a MTT assay was performed to evaluate the in vitro cytotoxicity of the copolymer by the direct contact method using L929 fibroblast cells (Fig. 9). The cell viability after treatment with the PGNA copolymer is expressed as a percentage of the blank control cells, which were cultured without the copolymer in the culture medium (viability 100%). As shown in Fig. 9, the cell viabilities are all >80% and there is only a small decrease for all concentrations, indicating that the triblock copolymer has no significant effect on the cell viability and could be used as a drug carrier with a low cytotoxicity. A possible reason for the decrease in the cell viability might be that this triblock copolymer forms a very stable and dense gel covering on the cells, which leads to the cells being unable to get enough air and nutrients.
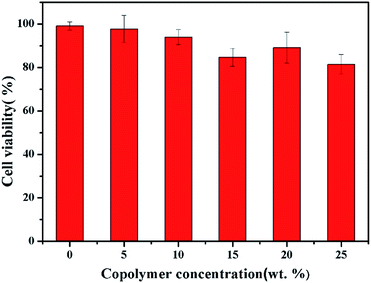 |
| Fig. 9 In vitro cell viability of L929 cells treated with PGNA2 copolymer at different concentrations for 24 h. | |
4. Conclusions
A novel simple glucosyl triblock copolymer was successfully synthesized by sequential RAFT polymerization with CMP as the chain transfer agent. It was found that the triblock copolymer PGNA is a biocompatible gelator that shows a reversible sol–gel–syneresis phase transition in aqueous solution, and the hydrogel exhibited excellent temperature and pH response behaviours. It formed a stable sol state at room temperature and low pH values and became a gel under physiological conditions (pH 7.4, 37 °C). A white gel was formed quickly in situ after the injection of the copolymer solution into an SD. The in vitro release of aspirin from the hydrogel implied that the hydrogel has controlled release properties. The in vitro cytotoxicity test showed that the copolymer is not cytotoxic. These results indicate that hydrogels are promising injectable pH/temperature-sensitive carriers for sustained drug delivery systems.
Acknowledgements
This work was supported by the National Natural Science Foundation of China (nos 21476117, 21376124, 21303090) and the Science and Technology Projects Fund of Nantong City (BK2014014).
References
- G. P. T. Dzinomwal, C. J. Woodl and D. J. T. Hill, Polym. Adv. Technol., 1997, 8, 767–772 CrossRef.
- D. Gao, R. B. Heimann and S. D. B. Alexander, Adv. Cem. Res., 1997, 35, 93–97 CrossRef.
- A. T. Paulino, M. R. Guilherme, A. V. Reis, G. M. Campese, E. C. Muniz and J. Nozaki, J. Colloid Interface Sci., 2006, 301, 55–62 CrossRef CAS PubMed.
- S. Chatterjee, M. W. Lee and S. H. Woo, Bioresour. Technol., 2010, 101, 1800–1806 CrossRef CAS PubMed.
- J. Woodhouse and M. S. Johnson, Agr. Water Manag., 1991, 20, 63–70 CrossRef.
- S. J. Kohls, D. D. Baker, D. A. Kremer and J. O. Dawson, Plant Soil, 1999, 214, 105–115 CrossRef CAS.
- A. Hüttermann, M. Zommorodi and K. Reise, Soil Tillage Res., 1999, 50, 295–304 CrossRef.
- V. Arbona, D. J. Iglesias, J. Jacas, E. Primo-Millo, M. Talon and A. Gómez-Cadenas, Plant Soil, 2005, 270, 73–82 CrossRef CAS PubMed.
- E. Chirino, A. Vilagrosa and V. R. Vallejo, Plant Soil, 2011, 344, 99–110 CrossRef CAS PubMed.
- Y. Tabata, A. Nagano and Y. Ikada, Tissue Eng., 1999, 5, 127–138 CrossRef CAS.
- S. Kiyonaka, K. Sada, I. Yoshimura, S. Shinkai, N. Kato and I. Hamachi, Nat. Mater., 2004, 3, 58–64 CrossRef CAS PubMed.
- Y. F. Li, X. Z. Li, L. C. Zhou, X. X. Zhu and B. N. Li, Polym. Adv. Technol., 2004, 15, 34–38 CrossRef CAS.
- L. S. Ferreira, S. Gerecht, J. Fuller, H. F. Shieh, G. Vunjak-Novakovic and R. Langer, Biomaterials, 2007, 28, 2706–2717 CrossRef CAS PubMed.
- J. Jagur-Grodzinski, Polym. Adv. Technol., 2010, 21, 27–47 CAS.
- I. Cifková, P. Lopour, P. Vondráček and F. Jelínek, Biomaterials, 1990, 11, 393–396 CrossRef.
- P. Hron, J. Šlechtová, K. Smetana, B. Dvořánková and P. Lopour, Biomaterials, 1997, 18, 1069–1073 CrossRef CAS.
- K. Thomas, Y. X. Li and U. Florian, Adv. Drug Delivery Rev., 2002, 54, 99–134 CrossRef.
- R. Fernandes, Q. W. Li, T. H. Chen, H. Yi, G. W. Rubloff, R. Ghodssi, W. E. Bentley and G. F. Payne, Langmuir, 2003, 19, 4058–4062 CrossRef CAS.
- I. Roy and M. N. Gupta, Chem. Biol., 2003, 10, 1161–1171 CrossRef CAS PubMed.
- M. Heskins and J. E. Guillet, J. Macromol. Sci., Chem., 1968, 2, 1441–1455 CrossRef CAS.
- H. G. Schild, Prog. Polym. Sci., 1992, 17, 163–249 CrossRef CAS.
- A. B. Lowe and C. L. McCormick, Prog. Polym. Sci., 2007, 32, 283–351 CrossRef CAS PubMed.
- G. Chen and A. S. Hoffman, Nature, 1995, 373, 49–52 CrossRef CAS PubMed.
- C. S. Brazel and N. A. Peppas, Macromolecules, 1995, 28, 8016–8020 CrossRef CAS.
- V. Bulmus, Z. Ding, C. J. Long, P. S. Stayton and A.
S. Hoffman, Bioconjugate Chem., 2000, 11, 78–83 CrossRef CAS PubMed.
- X. Yin, A. S. Hoffman and P. S. Stayton, Biomacromolecules, 2006, 7, 1381–1385 CrossRef CAS PubMed.
- Y. F. Tang, S. M. Zhang, M. Wang, J. L. Zhu, T. M. Sun and G. Q. Jiang, J. Polym. Res., 2014, 21, 1–8 CAS.
- X. P. Chen and A. Neil, Macromolecules, 2010, 43, 1341–1348 CrossRef CAS.
- X. P. Chen and A. Neil, J. Polym. Sci., Part A: Polym. Chem., 2011, 49, 3030–3037 CrossRef CAS.
- G. Koßmehl and J. Volkheime, Liebigs Ann. Chem., 1989, 1127–1130 CrossRef.
- D. Leung and C. N. Bowman, Macromol. Chem. Phys., 2012, 213, 198–204 CrossRef CAS.
- D. P. Huynh, W. S. Shim, J. H. Kim and D. S. Lee, Polymer, 2006, 47, 7918–7926 CrossRef CAS PubMed.
- D. P. Huynh, G. J. Im, S. Y. Chae, K. C. Lee and D. S. Lee, J. Controlled Release, 2009, 137, 20–24 CrossRef CAS PubMed.
- J. H. Han, J. M. Krochta, M. J. Kurth and Y. L. Hsieh, J. Agric. Food Chem., 2000, 48, 5278–5282 CrossRef CAS PubMed.
- X. F. Sun, H. H. Wang, Z. X. Jing and R. Mohanathas, Carbohydr. Polym., 2013, 92, 1357–1366 CrossRef CAS PubMed.
- W. S. Shim, S. W. Kim and D. S. Lee, Biomacromolecules, 2006, 7, 1935–1941 CrossRef CAS PubMed.
- Y. J. Oh, I. In and S. Y. Park, J. Ind. Eng. Chem., 2012, 18, 321–324 CrossRef CAS PubMed.
|
This journal is © The Royal Society of Chemistry 2015 |